On the reactivity of anodically generated trifluoromethyl radicals toward aryl alkynes in organic/aqueous media†
Received
23rd February 2019
, Accepted 12th March 2019
First published on 12th March 2019
Abstract
An in-depth study of the reaction of electrochemically generated trifluoromethyl radicals with aryl alkynes in the presence of water is presented. The radicals are readily generated by anodic oxidation of sodium triflinate, an inexpensive and readily available CF3 source, with concomitant reduction of water. Two competitive pathways, i.e. aryl trifluoromethylation vs. oxytrifluoromethylation of the alkyne, which ultimately lead to the generation of α-trifluoromethyl ketones, have been observed. The influence of several reaction parameters on the reaction selectivity, including solvent effects, electrode materials and substitution patterns on the aromatic ring of the substrate, has been investigated. A mechanistic rationale for the generation α-trifluoromethyl ketones based on cyclic voltammetry data and radical trapping experiments is also presented. DFT calculations carried out at the M06-2X/6-311+G(d,p) level on the two competing pathways account for the observed selectivity.
Introduction
Incorporation of perfluoroalkyl moieties into organic molecules typically enhances some of their biological properties such as metabolic stability, lipophilicity and bioavailability,1 and hence represents an important type of modification in the production of agrochemicals, materials and pharmaceuticals (Fig. 1).2 Over the past two decades, a plethora of synthetic methods for the generation of C–C bonds between perfluoroalkyl groups and organic scaffolds have been reported.3 In particular, research on the introduction of trifluoromethyl groups (CF3) has gained significant attention.4 To this end, a variety of different trifluoromethyl donors have been developed, ranging from simple and inexpensive compounds, such as triflyl chloride,5 trifluoroiodomethane,6 trifluoromethyltrialkylsilane7 and sodium triflinate,8 to more complex and expensive chemicals like Umemoto's or Togni's reagents.9 In particular, the generation of α-trifluoromethyl ketones, which may serve as versatile building blocks for a diverse set of CF3-containing molecules, has been subjected to intense research and is considered to be an especially challenging task.10 Common synthetic protocols involve electrophilic or radical trifluoromethylations of silyl enol ethers,10–12 or enamines,13 which are generated as reactive intermediates from the parent ketone. Ideally, isolation of these intermediates is avoided, affording a one-pot, two-step procedure.12 Alternatively, α-trifluoromethyl ketones can be obtained by direct radical trifluoromethylation of alkenes14 or alkynes15 in the presence of an oxidizing agent (e.g. molecular oxygen) or a hydroxyl source, respectively.
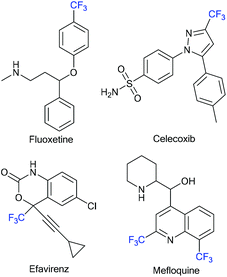 |
| Fig. 1 Examples of CF3-containing active pharmaceutical ingredients. | |
Radical trifluoromethylations are typically promoted by chemical oxidation of a CF3 source or photochemical methods (e.g. photoredox catalysis).4 Generation of CF3 radicals can also be achieved by electrochemical methods, enabling the aforementioned redox process without the need for metal- or photocatalysts or stoichiometric amounts of hazardous oxidizing or reducing agents, giving rise to highly sustainable processes.16 In fact, owing to the “inherently green” character of electroorganic synthesis,17 this technology has seen a considerable resurgence over the past few years. Generation of CF3 radicals by anodic oxidation of several CF3 sources, including CF3COOH,18 CF3SO2Na,19 or (CF3SO2)2Zn,20 and its application in the trifluoromethylation of arenes and alkenes have been reported. Recently, we have developed a novel route for the oxytrifluroromethylation of alkenes, enabled by the electrochemical oxidation of CF3SO2Na in the presence of water.21
Herein, the reactivity of anodically generated CF3 radicals in organic/aqueous media is extended to more challenging aryl alkyne substrates. A rather similar nucleophilic character of the alkyne and the aromatic moiety results in a competition between two reaction pathways: oxytrifluoromethylation of the alkyne, which leads to the corresponding α-trifluoromethyl ketone after tautomerization of the ensuing enol, and arene trifluoromethylation (Fig. 2). In an attempt to harness reaction selectivity, the effect of several reaction parameters on product distribution has been carefully studied. Formation of α-trifluoromethyl ketones was favored in most cases, although mixtures with trifluoromethyl-aryl products were always observed. DFT calculations at the M06-2X/6-311+G(d,p) level on the two competing pathways have been carried out to explain the observed selectivity.
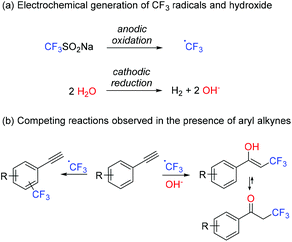 |
| Fig. 2 (a) Generation of CF3 radicals by anodic oxidation of sodium triflinate with concomitant reduction of water and (b) competition between oxytrifluoromethylation and aryl trifluoromethylation in the presence of aryl alkynes. | |
Results and discussion
Aryl vs. alkyne trifluoromethylation: effect of the solvent composition and electrode materials on reaction selectivity
Our investigation was carried out using 4-tert-butylphenylacetylene (1a) as a model aryl alkyne. All experiments were carried out in a standardized electrochemical reactor (IKA ElectraSyn) at constant current under a nitrogen atmosphere. An initial set of experiments was performed to evaluate the influence of the organic solvent, electrode material and electrolyte on the reaction outcome. All experiments were carried out on a 0.5 mmol scale, using a 0.2 M concentration for the alkyne and 1.2 equiv. CF3SO2Na. A constant current of 30 mA and a total charge of 2.2 F mol−1 (10% excess over the theoretical charge required) were applied. Notably, good to excellent conversions were obtained in all cases and, as anticipated, mixtures of the α-trifluoromethyl ketone 2a and trifluoromethyl-aryl derivatives 3a–4a were detected by GC analysis. Thus, in addition to the expected trifluoromethylaryl alkyne 3a, aryl trifluoromethylation of the α-trifluoromethyl ketone 2a (compound 4a) was also observed. Formation of 4a is expected to take place in the later stage of the reaction, when high concentrations of 2a are present in the mixture (vide infra). Minor amounts (<5%) of trifluoromethylsulfonylated aromatics were also observed in some cases (for a GC-FID chromatogram of an example of the crude reaction, see Fig. S1 in the ESI†).
Using acetone as the solvent, a series of anode/cathode material combinations were evaluated (Table 1, entries 1–4). While maintaining graphite as the anode, altering the cathode material (graphite, Pt, Ni, stainless steel) only had a minor influence on the reaction outcome, with 2a being formed with highest selectivity (Table 1, entries 1–4). In contrast, complex mixtures of products were obtained with both Pt and RVC as anodes (Table 1, entries 5 and 6). This could be attributed to lower overpotentials for some undesired oxidations on Pt, or the fact that its surface may be catalytically active for such side-reactions, and a poor mixing of the reaction mixture within the pores in the case of the RVC material.
Table 1 Influence of the electrode type, solvent and electrolyte on the outcome of the reaction of 1a with CF3SO2Na under constant current electrolysis in organic/aqueous mediaa
Next, a series of solvents covering a wide range of dielectric constants were screened (ε = 35.7 for MeCN and ε = 7.5 and 7.0 for MeTHF and THF, respectively). The solvent choice may influence both the electrolysis efficiency, as the maximum amount of current that can be passed to the reagent solution while keeping the voltage in a 3–4 V range depends on the dielectric constant of the solvent, and the selectivity of the reaction itself. Best conversions were typically obtained in acetone. In addition, acetone favored the formation of 2a, with a selectivity of up to 67% (Table 1, entry 3). Notably, the selectivity could be inverted in THF and MeTHF (entries 7 and 8). A selectivity of 65% toward aryl trifluoromethylation was observed using MeTHF as the solvent (entry 8), although the reaction conversion was significantly lower than that for acetone. Using MeOH as the solvent, an α-trifluoromethyl methyl-enol ether was formed as a product with 48% selectivity, resulting from methoxide acting as a nucleophile instead of water (Table 1, entry 10) (see Fig. S2 in the ESI†). Although full conversion was obtained in DCM, ca. 30% of an undesired, unidentified side-product was detected by GC. The type of electrolyte used had little influence on the product distribution (Table 1, entries 12 and 13). An additional experiment under potentiostatic conditions (constant voltage of 4 V) showed no noticeable difference to the reaction at constant current (Table 1, entry 14 vs. entry 4). As expected, the reaction did not proceed in the absence of electricity, proving that an electrochemical redox process was involved in the generation of the CF3 radicals (Table 1, entry 15).
An additional set of reaction parameters, namely the amount of water and CF3SO2Na, temperature, concentration, current and total charge, was next investigated (Fig. 3). Conversion increased when low amounts of water were utilized (Fig. 3a). Notably, the 2a
:
3a–4a ratio decreased with increasing amounts of water, and the favored product was inverted when the amount of water increased from 47 to 97 equiv. The increase of temperature, substrate concentration, amount of CF3SO2Na and charge had positive effects on the reaction conversion, but a less important influence on the 2a
:
3a–4a product ratio (Fig. 3b–e). Low currents (Fig. 3f) favored higher 2a
:
3a–4a ratios, as well as higher substrate conversion. In most cases, α-trifluoromethyl ketone 2a was the favored product of the reaction. As mentioned above, an excess of CF3SO2Na was required to achieve high conversions. 19F-NMR monitoring of the reaction mixture revealed consumption of more than one equivalent of sodium triflinate at conversions below 90%. This effect could be ascribed to trapping of some of the CF3 radicals by e.g. water and formation of volatile species.
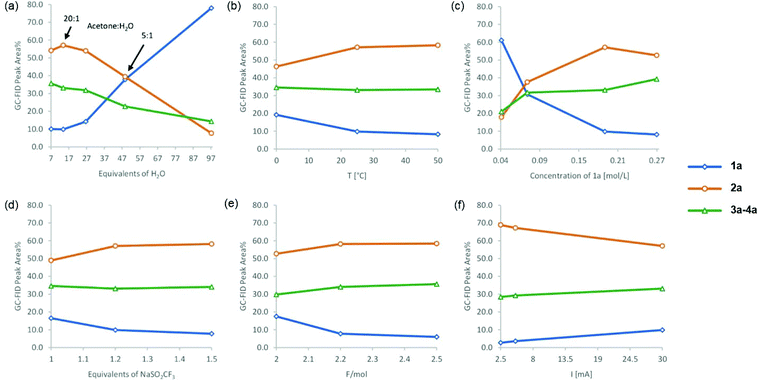 |
| Fig. 3 Influence of the amount of water (a), temperature (b), concentration (c), equivalents of CF3SO2Na (d), charge (e) and current (f) on the electrochemical transformation of 1a. Typical conditions: 0.5 mmol substrate, 1.2 equiv. CF3SO2Na, acetone : H2O 20 : 1 (v/v), 0.1 M Et4NBF4, 2.2 F mol−1, constant current (30 mA), anode: graphite, cathode: stainless steel, and 2.625 mL reaction volume. For a more detailed representation, including the amounts of all side products, see Fig. S3 in the ESI†. | |
Effect of substrate substituents. Reaction scope
To evaluate the functional group tolerance under the electrolysis conditions and assess their effect on the reaction selectivity, a diverse set of aryl alkynes was subjected to the electrochemical trifluoromethylation (Scheme 1). α-Trifluoromethyl ketones 2 were favored in most cases. For that reason, derivatives 2 were isolated and characterized. Yields for 2 and the trifluoromethyl-aryl adducts 3–4 were also determined by 19F NMR for comparison. Since the reactions were carried out in acetone, minor amounts (<10%) of the acetone aldol condensation products were detected in some reaction mixtures, which were likely formed under the basic conditions generated during the reaction. These impurities could easily be removed by evaporation.
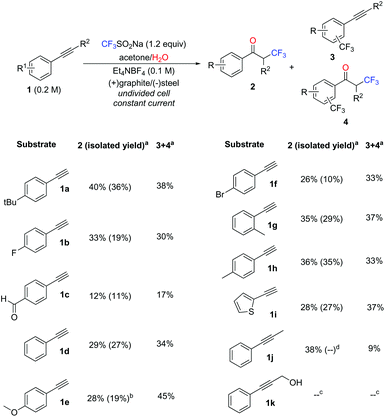 |
| Scheme 1 Reaction scope for the electrochemical transformation of alkynes in the presence of NaSO2CF3. Conditions: 1.0 mmol substrate, 1.2 equiv. NaSO2CF3, acetone : H2O 20 : 1 (v/v), 0.1 M Et4NBF4, 2.2 F mol−1, constant current (15 mA), anode: graphite, cathode: stainless steel, and 5 mL reaction volume a Determined by 19F-NMR. b Essay corrected; the isolated material (32%) contained 3e. c See Scheme 2. d Ketone 2j could not be separated from the side products by column chromatography. | |
Aromatic alkynes bearing both electron withdrawing and electron donating substituents, as well as a heterocyclic aryl alkyne, were reacted with NaSO2CF3 under electrolytic conditions (see the Experimental section for details). Surprisingly, despite the presence of deactivating functional groups on the arene in substrates 1b and 1f, the ratio of products did not shift in favor of the α-trifluoromethyl ketone 2. In contrast, during the reaction of the electron-rich 4-methoxyphenyl acetylene (1e), trifluoromethylation of the ring was clearly favored (Scheme 1). The CF3 radical addition reaction proved to be tolerant to steric effects, as both para methyl (1h) and ortho methyl (1g) phenylacetylene could be functionalized with similar yields. A non-terminal alkyne (1j) was also successfully derivatized under the standard reactions conditions. Notably, even the oxidatively labile substrate 1c was converted into the α-trifluoromethyl ketone 2c in modest yield. Isolation of pure α-trifluoromethyl ketones from the other reaction products was possible by simple column chromatography. Separation of 2e from 3e and 2j from 3j was problematic due to the very similar polarity of the products.
In an attempt to improve the reaction of oxidatively labile substances (e.g.1c and 1k), three common oxidation mediators [triphenylamine, TEMPO and manganese(III) acetate dihydrate] were evaluated for the trifluoromethylation of these substrates as well as the model alkyne 1a. Although a decrease of the current from 30 mA to 5 mA had a positive effect on the conversion of 1a and 1c, the presence of mediators did not significantly change the reaction outcome (see Table S1 in the ESI†). Notably, the reaction of 1k did not afford the expected ketone 2, but trifluoromethylacrylophenone 5 (Scheme 2). This compound is likely formed by intramolecular trapping of the carbocation intermediate 6 by the OH group (instead of water, which leads to the expected product 2). The ensuing unstable oxetane 7 rearranges to compound 5. Although 5 was observed in good yields (54%) by GC, its high reactivity did not allow its isolation in pure form (see Fig. S4 in the ESI†).
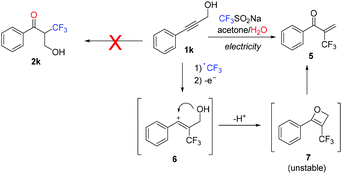 |
| Scheme 2 Reaction outcome for alkyne 1j utilizing the typical electrolysis conditions (Scheme 1). | |
Proposed mechanism for the generation of α-trifluoromethyl ketones
Cyclic voltammograms of the starting materials (Fig. 4a) revealed that oxidation of sodium triflinate occurs at 1.1–1.3 V (vs. Ag/AgCl), and is followed by an irreversible reaction, while the model aryl alkyne substrate 1a and even oxidatively labile alkynes require much higher oxidation potentials (2.2–2.5 V) (see Fig. 4a and S5 in the ESI† for other alkynes). Thus, oxidation at the anode should involve the CF3 source with good selectivity.
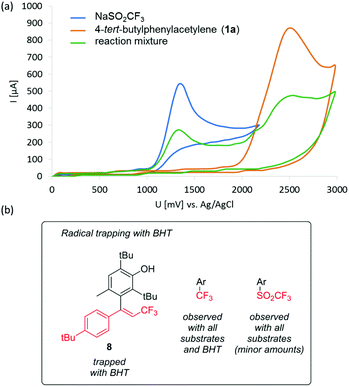 |
| Fig. 4 (a) Cyclic voltammograms of CF3SO2Na, 4-tert-butylphenylacetylene (1a) and a typical reaction mixture (see the ESI† for details). (b) Species detected by GC-MS that point to the presence of the radical species highlighted in red. | |
Oxidation of triflinate is known to generate CF3 radicals,8,19 likely by decomposition of the initially formed CF3SO2 radical, as pointed out by the side products observed in the reaction (see Fig. S6 in the ESI†). To provide evidence for a radical reaction mechanism, we carried out a control experiment using the typical electrolysis conditions (Scheme 1) for the model substrate 1a and using 1 equiv. of butylated hydroxytoluene (2,6-di-tert-butyl-4-methylphenol, BHT) as the additive. Cyclic voltammetry confirmed that BHT is oxidized at higher potential than CF3SO2Na, and therefore it is a suitable radical trapping agent for this reaction (see Fig. S5 in the ESI†). GC-MS analysis of the mixture after electrolysis revealed (see Fig. S7 in the ESI†) the presence of a large amount of unreacted 1a, confirming that BHT quenched the reaction, as well as trifluoromethylated BHT and small amounts of α-trifluoromethyl ketones 2a and 3a. To our delight, compound 8 (Fig. 3b), corresponding to the trapping of a plausible trifluoromethylalkenyl radical, could also be detected by GC-MS analysis.
Thus, the proposed mechanism for the formation of α-trifluoromethyl ketones (Fig. 5) starts with the anodic oxidation of the triflinate anion, producing a trifluoromethylsulfonyl radical which decomposes into a CF3 radical and SO2. Subsequently, the CF3 radical adds to the triple bond of the substrate in an anti-Markovnikov fashion, giving rise to the secondary alkyl radical 9 stabilized by the adjacent aromatic ring. Further oxidation of 9 produces carbocation 10. The carbocationic species is trapped by water or the hydroxide generated at the cathode, affording an enol ether 6, which tautomerizes to the ketone 2. The proposed mechanism represents an example of a paired electrochemical reaction: the hydroxide ions generated at the cathode during the electrolysis are also involved in the reaction, as one equivalent of hydroxide is incorporated into the final molecule.
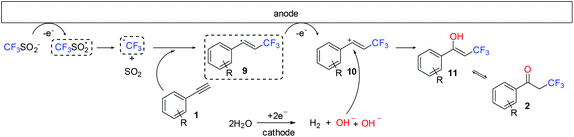 |
| Fig. 5 Proposed reaction mechanism for the formation of α-trifluoromethyl ketones 2 from alkynes and electrochemically generated CF3 radicals in the presence of water. | |
Differential selectivity analysis and computational evaluation
As described above, reactions of aryl alkynes with electrochemically generated CF3 radicals in the presence of water produced mixtures of α-trifluoromethyl ketones 2 and products derived from trifluoromethylation of the aromatic ring (3a–4a). This occurred under all reaction conditions evaluated (Table 1 and Fig. 2) and with all aryl alkynes tested (Scheme 1). Motivated by these somewhat surprising results–the reaction could not be directed to one of the two expected products, we decided to have a more detailed look into the reaction kinetics and assess the two competing pathways by DFT calculations, with the aim of providing an explanation to the observed product distribution.
HPLC monitoring of the model reaction (Fig. S8†) revealed the expected formation of compounds 2a and 3a from the initial reaction stage, and a gradual increase in the amount of 4a starting after 0.5 F mol−1. This is due to the fact that 4a is formed from 2a. The reaction selectivity can be more clearly visualized by analyzing the corresponding differential selectivity plots (Fig. 6), which represent the relative amount of the reaction products at different reaction stages. Thus, the linear differential selectivity plot obtained for 2avs. 3a (Fig. 6a) showed that the generation of the two compounds occurs simultaneously during the whole process, with a constant relative rate. The slope of the linear plot (ca. 0.32) indicated that the formation of 2a is approximately 3 times faster than that of 3a. Selectivity values for 2a did not match this relative rate (cf.Table 1) due to the formation of other side products (e.g.4a), which take place at later stages of the reaction as shown in Fig. 6b. Thus, the differential selectivity of 2avs. the sum of all trifluoromethyl-aryl products (3a, 4a and minor amounts of trifluoromethylsulfonyl derivatives) has a curved concave shape, indicating that the rate of formation of trifluoromethyl arenes increases with respect to the formation of 2a with the reaction progress. These results were expected, as the rate of formation of 4a is proportional to the amount of 2a in the reaction mixture.
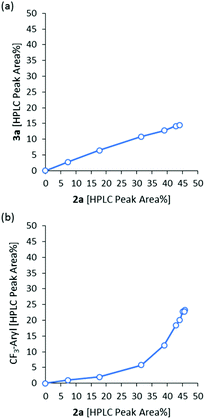 |
| Fig. 6 Differential selectivity plots for the formation of 2avs. 3a (a) and 2avs. aryl-trifluoromethyl species (b), obtained by HPLC monitoring of the reaction mixture. | |
The two reaction pathways, leading to the generation of compounds 2 and 3, were then assessed by DFT calculations to ascertain the origin of the product distribution experimentally observed. Thus, the stationary points involved in the addition of the CF3 radical to the alkyne and aromatic ring were modelled using the M06-2X functional22 and the 6-311+G(d,p) basis set. All calculations incorporated solvent effects with the SMD model,23 both for geometry optimization and frequency analyses, using acetone as the solvent. To reduce the computational cost of the calculations, the reaction of phenyl acetylene (1d) was selected as a model. The results were compared with the reaction of the trifluoromethyl radical with styrene (1d′) (Fig. 7a), which in previous studies had shown a clear preference for the radical addition to the olefin over the addition to the aromatic ring.21 While the reaction of the CF3 radical with the alkyne and the alkene was simply modelled for the C-2 addition, matching experimental observations, the radical addition to the aromatic ring was calculated for the ortho-, meta-, and para-positions. In the case of styrene, the two possible isomers for the ortho- and meta-addition of the CF3 radical (depending on the alkene orientation) were taken into account. The energy of the most stable isomer is presented.
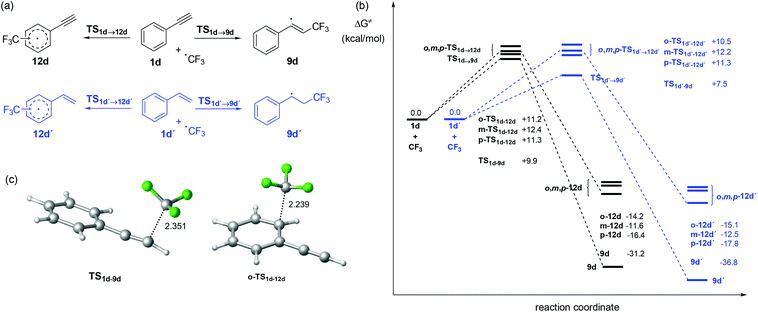 |
| Fig. 7 (a) Computed radical additions to the aromatic ring and the alkyne (1d) or alkene (1d'); (b) energy profiles calculated at the M06-2X/6-311+G(d,p) level. The similar energy barriers obtained for the radical additions to the alkyne and arene of 1d explain the mixtures of products observed experimentally; (c) structures of selected transition states. | |
The energy profile obtained for the reaction of phenyl acetylene (1d) with the CF3 radical (Fig. 7b, black color) revealed analogous energy barriers for all possible radical addition pathways, being all within a range of ca. 2 kcal mol−1. The difference in energy between the transition state for the radical addition to the triple bond (TS1d–9d) and the most favored of the arene additions (o-TS1d–12d) was only 1.3 kcal mol−1. Importantly, the similar energetics for the competing reactions satisfactorily explains the mixtures of products observed in all cases (cf.Table 1 and Fig. 2). As expected, the generation of adducts 9d and 12d was exergonic. 31.2 kcal mol−1 and 11.6–16.4 kcal mol−1 are released during the formation of 9d and 12d, respectively.
On the other hand, the energy profile for the reaction of the CF3 radical with styrene 1d′ (Fig. 7b, blue color) presented analogous energy barriers for the addition of the radical to the arene (10.5–12.2 kcal mol−1). However, the barrier for the addition to the alkene moiety resulted in significantly smaller values (7.5 kcal mol−1). This difference (3 kcal mol−1) accounts for the selective oxytrifluoromethylations achieved when styrene derivatives are reacted with CF3 radicals in organic aqueous media.21 The fate of the trifluoromethylsulfonyl (CF3SO2) radical that is initially formed and triggers the reaction mechanism (Fig. 5) was also modelled at the same level of theory. Notably, the calculations also predicted rapid decomposition of the radical with extrusion of SO2, with an energy barrier of only 5.9 kcal mol−1. This low barrier explained that only minor amounts of products containing the trifluoromethylsulfonyl moiety, via trapping of this radical, were observed experimentally.
Conclusions
In summary, the present work provides a detailed experimental and theoretical study on the reactivity of electrochemically generated CF3 radicals with aryl alkynes in the presence of water. The radicals were generated by anodic oxidation of CF3SO2Na in an undivided cell. Similar reactivity toward radicals of the alkyne and arene moieties led to two major reaction pathways: trifluoromethylation of the aromatic ring and oxytrifluoromethylation of the alkyne. The oxytrifluoromethylation process produced a 2-trifluoromethyl enol that upon tautomerization resulted in the formation of an α-trifluoromethyl ketone. The effect on the product distribution of several reaction parameters was evaluated. Formation of α-trifluoromethyl ketones was favored in most cases, except when THF or MeTHF was used as the solvent. Several aryl alkynes decorated with electron-withdrawing and donating groups were tested. The reaction performed well in all cases, although it always led to mixtures of products from the two competing pathways. A computational study of the radical additions to the arene and alkyne, leading to the two competing mechanisms, was carried out at the M06-2X/6-311+G(d,p) level. The analogous energy barriers calculated for all the radical additions successfully explained the selectivity observed experimentally.
Experimental section
General
1H NMR spectra were recorded on a 300 MHz instrument. 13C NMR and 19F NMR spectra were recorded on the same instrument at 75 MHz and 282 MHz, respectively. Routine 19F NMR monitoring was carried out in a Magritek Spinsolve 43 benchtop NMR instrument. Chemical shifts (δ) are expressed in ppm downfield from TMS as the internal standard. The letters s, d, t, q, and m are used to indicate singlet, doublet, triplet, quadruplet, and multiplet, respectively. GC-FID analysis was performed on a ThermoFisher Focus GC with a flame ionization detector, using a TR-5MS column (30 m × 0.25 mm ID × 0.25 μm) and helium as the carrier gas (1 mL min−1 constant flow). The injector temperature was set to 280 °C. After 1 min at 50 °C, the temperature was increased by 25 °C min−1 to 300 °C and kept constant at 300 °C for 4 min. The detector gases used for flame ionization were hydrogen and synthetic air (5.0 quality). GC-MS spectra were recorded using a ThermoFisher Focus GC coupled with a DSQ II (EI, 70 eV). A TR-5MS column (30 m × 0.25 mm × 0.25 μm) was used, with helium as the carrier gas (1 mL min−1 constant flow). The injector temperature was set to 280 °C. After 1 min at 50 °C, the temperature was increased by 25 °C min−1 to 300 °C and kept at 300 °C for 3 min. Analytical HPLC analysis was carried out on a C18 reversed-phase (RP) analytical column (150 × 4.6 mm, particle size 5 mm) at 37 °C by using mobile phases A [water/acetonitrile 90
:
10 (v/v) + 0.1% TFA] and B (acetonitrile + 0.1% TFA) at a flow rate of 1.5 mL min−1. The following gradient was applied: a linear increase from solution 30% B to 100% B in 8 min, and hold at 100% solution B for 2 min. Flash chromatography purifications were carried out on an automated flash chromatography system using cartridges packed with KP-SIL, 60 Å (32–63 μm particle size). Sodium trifluoromethanesulfinate (Code: 743232, Lot: BCBX4470), tetrabutylammonium tetrafluoroborate (Code: 217964, Lot: BCBV1430), tetraethylammonium tetrafluoroborate (Code: 242144, Lot: BCBV4670) and lithium perchlorate (Code: 634565, Lot: 0000011388) were purchased from Aldrich. All other chemicals were obtained from standard commercial vendors and were used without any further purification. Electrochemical reactions and cyclic voltammetry experiments were carried out in an IKA ElectraSyn 2.0.
Computational details
All calculations were carried out with the Gaussian 09 package.24 The M06-2X density functional method22 in conjunction with the 6-311+G(d,p) basis set was selected for all the geometry optimizations and frequency analysis. The geometries were optimized with the inclusion of solvation effects. For this purpose, the SMD solvation method23 was employed using acetone as the solvent. Frequency calculations at 298.15 K on all stationary points were carried out at the same level of theory as the geometry optimizations to ascertain the nature of the stationary points. Ground and transition states were characterized by none and one imaginary frequency, respectively. All of the presented relative energies are free energies at 298.15 K.
Experimental procedure for the electrochemical transformation of aromatic alkynes on the preparative scale
All reactions were carried out in an IKA ElectraSyn 2.0 using an IKA Graphite SK-50 anode and an IKA Stainless steel cathode. In a 5 mL IKA ElectraSyn vial, equipped with a stir bar, 0.5 mmol electrolyte (tetraethylammonium tetrafluoroborate, Et4NBF4), 1.2 mmol NaSO2CF3 and 1 mmol alkyne were mixed with 5 mL acetone and 250 μL water. After assembly of the electrochemical cell, the reaction mixtures were purged with N2 for five minutes prior to switching on electricity to ensure an oxygen- and CO2-free atmosphere. The instrument was operated in a constant current mode (15 mA) and 2.2 F mol−1 of charge were passed. After completion of the reaction, the crude product mixture was concentrated under reduced pressure and the residue was purified by flash column chromatography using petroleum ether/ethyl acetate as the eluent.
1-(4-tert-Butyl)phenyl-3,3,3-trifluoropropan-1-one (2a).
86 mg (36%); yellow oil; 1H NMR (300 MHz, CDCl3) δ 7.88 (d, J = 8.1 Hz, 2H), 7.52 (d, J = 8.1 Hz, 2H), 3.77 (q, J = 10.0 Hz, 2H), 1.35 (s, 9H). 13C NMR (75 MHz, CDCl3) δ 189.4, 158.3, 133.4 (d, J = 1.8 Hz), 128.4, 126.0, 124.0 (q, J = 244.5 Hz), 42.1 (q, J = 28.1 Hz), 35.4, 31.1. 19F NMR (282 MHz, CDCl3) δ −61.98 (t, J = 10.1 Hz). MS-EI: m/z 229 (75%), 201 (24%), 161 (30%), 110 (100%).
3,3,3-Trifluoro-1-(4-fluorophenyl)propan-1-one (2b).
35 mg (19%); yellow oil; 1H NMR (300 MHz, CDCl3) δ 8.02–7.92 (m, 2H), 7.25–7.11 (m, 2H), 3.77 (q, J = 9.9 Hz, 2H). 13C NMR (75 MHz, CDCl3) δ 188.3, 168.2, 164.8, 132.4, 131.4, 131.2, 124.0 (q, J = 277.1 Hz), 116.5, 116.2, 42.3 (q, J = 28.3 Hz). 19F NMR (282 MHz, CDCl3) δ −62.03 (t, J = 10.0 Hz, 3F), −102.86 (m, 1F). MS-EI: m/z 206 (6%), 123 (100%), 95 (76%).
4-(3,3,3-Trifluoropropanoyl)benzaldehyde (2c).
23 mg (11%); yellow oil; 1H NMR (300 MHz, CDCl3) δ 10.13 (s, 1H), 8.09 (d, J = 8.4 Hz, 2H), 8.02 (d, J = 8.4 Hz, 2H), 3.85 (q, J = 9.8 Hz, 2H). 13C NMR (75 MHz, CDCl3) δ 191.4, 189.3, 139.8, 130.1, 129.1, 123.8 (q, J = 276.0 Hz), 42.7 (q, J = 28.6 Hz). 19F NMR (282 MHz, CDCl3) δ −62.01 (t, J = 9.8 Hz). MS-EI: m/z 216 (7%), 133 (80%), 105 (35%).
3,3,3-Trifluoro-1-phenylpropan-1-one (2d).
47 mg (27%); yellow oil; 1H NMR (300 MHz, CDCl3) δ 7.99–7.91 (m, 2H), 7.68–7.60 (m, 1H), 7.55–7.48 (m, 2H), 3.80 (q, J = 10.0 Hz, 2H). 13C NMR (75 MHz, CDCl3) δ 189.8, 135.9, 134.4, 129.1, 128.5, 124.1 (q, J = 277.0 Hz), 42.2 (q, J = 28.2 Hz). 19F NMR (282 MHz, CDCl3) δ −62.04 (t, J = 9.9 Hz). MS-EI: m/z 188 (8%), 105 (100%), 77 (86%).
1-(4-Bromophenyl)-3,3,3-trifluoropropan-1-one (2f).
26 mg (10%); yellow oil; 1H NMR (300 MHz, CDCl3) δ 7.85–7.75 (m, 2H), 7.70–7.61 (m, 2H), 3.76 (q, J = 9.9 Hz, 2H). 13C NMR (75 MHz, CDCl3) δ 188.9, 134.6, 132.5, 130.0, 129.8, 123.9 (q, J = 277.1 Hz), 42.3 (q, J = 28.5 Hz). 19F NMR (282 MHz, CDCl3) δ −61.99 (t, J = 9.9 Hz). MS-EI: m/z 185 (93%), 183 (100%), 157 (64%), 155 (26%), 76 (98%), 74 (81%).
3,3,3-Trifluoro-1-(o-tolyl)propan-1-one (2g).
54 mg (29%); yellow oil; 1H NMR (300 MHz, CDCl3) δ 7.65–7.59 (m, 1H), 7.42–7.47 (m, 1H), 7.31 (t, J = 7.3 Hz, 2H), 3.75 (q, J = 10.1 Hz, 2H), 2.54 (s, 3H). 13C NMR (75 MHz, CDCl3) δ 192.9 (d, J = 2.4 Hz), 139.7, 136.0, 132.7, 132.6, 129.6, 129.1, 126.2, 124.1 (q, J = 277.1 Hz), 44.5 (q, J = 27.7 Hz), 21.7. 19F NMR (282 MHz, CDCl3) δ −62.09 (t, J = 10.0 Hz). MS-EI: m/z 202 (10%), 119 (74%), 91 (100%).
3,3,3-Trifluoro-1-(p-tolyl)propan-1-one (2h).
66 mg (35%); yellow oil; 1H NMR (300 MHz, CDCl3) δ 7.83 (d, J = 8.3 Hz, 2H), 7.30 (d, J = 8.3 Hz, 2H), 3.77 (q, J = 10.0 Hz, 2H), 2.43 (s, 3H). 13C NMR (75 MHz, CDCl3) δ 189.4 (d, J = 2.4 Hz), 145.5, 133.5 (d, J = 1.6 Hz), 129.8, 128.6, 124.2 (q, J = 275.3 Hz), 42.12 (q, J = 28.1 Hz), 21.9. 19F NMR (282 MHz, CDCl3) δ −62.02 (t, J = 10.0 Hz). MS-EI: m/z 202 (11%), 119 (99%), 91 (100%).
3,3,3-Trifluoro-1-(thiophen-2-yl)propan-1-one (2i).
47 mg (27%); yellow oil; 1H NMR (300 MHz, CDCl3) δ 7.76–7.72 (m, 2H), 7.18 (dd, J = 4.9, 3.9 Hz, 1H), 3.71 (q, J = 10.1 Hz, 2H). 13C NMR (75 MHz, CDCl3) δ 182.3 (d, J = 2.8 Hz), 143.3 (d, J = 2.0 Hz), 135.9, 133.6, 128.6, 123.8 (q, J = 277.3 Hz), 43.2 (q, J = 28.7 Hz). 19F NMR (282 MHz, CDCl3) δ −61.94 (t, J = 10.1 Hz). MS-EI: m/z 194 (9%), 111 (100%), 83 (23%).
Conflicts of interest
There are no conflicts to declare.
Acknowledgements
The CC FLOW Project (Austrian Research Promotion Agency FFG No. 862766) is funded through the Austrian COMET Program by the Austrian Federal Ministry of Transport, Innovation and Technology (BMVIT), the Austrian Federal Ministry of Science, Research and Economy (BMWFW), and by the State of Styria (Styrian Funding Agency SFG).
References
-
(a)
J.-P. Bégué and D. Bonnet-Delphon, Bioorganic and Medicinal Chemistry of Fluorine, John Wiley & Sons, Hoboken, 2008 CrossRef
;
(b)
Fluorine in Medicinal Chemistry and Chemical Biology, ed. I. Ojima, Wiley-VCH, Chichester, 2009 Search PubMed
.
-
(a) D. O'Hagan, Chem. Soc. Rev., 2008, 37, 308–319 RSC
;
(b) K. Müller, C. Faeh and F. Diederich, Science, 2007, 317, 1881–1886 CrossRef PubMed
;
(c) H.-J. Böhm, D. Banner, S. Bendels, M. Kansy, B. Kuhn, K. Müller, U. Obst-Sander and M. Stahl, ChemBioChem, 2004, 5, 634–643 CrossRef PubMed
.
-
(a) H.-X. Song, Q.-Y. Han, C.-L. Zhao and C.-P. Zhang, Green Chem., 2018, 20, 1662–1731 RSC
;
(b) C. Alonso, E. Martínez de Marigorta, G. Rubiales and F. Palacios, Chem. Rev., 2015, 115, 1847–1935 CrossRef CAS PubMed
.
- For selected recent reviews on trifluoromethylation chemistry see:
(a) G.-b. Li, C. Zhang, C. Song and Y.-d. Ma, Beilstein J. Org. Chem., 2018, 14, 155–181 CrossRef CAS PubMed
;
(b) E. H. Oh, H. J. Kim and S. B. Han, Synthesis, 2018, 50, 3346–3358 CrossRef CAS
;
(c) W. Wu and Z. Weng, Synthesis, 2018, 50, 1958–1964 CrossRef CAS
;
(d) Q. Lefebvre, Synlett, 2017, 28, 19–23 CrossRef CAS
;
(e) A. Prieto, O. Baudoin, D. Bouyssi and N. Monteiro, Chem. Commun., 2016, 52, 869–881 RSC
;
(f) M. Akita and T. Koike, C. R. Chim., 2015, 18, 742–751 CrossRef CAS
;
(g) P. Gao, X.-R. Song, X.-Y. Liu and Y.-M. Liang, Chem. – Eur. J., 2015, 21, 7648–7661 CrossRef CAS PubMed
;
(h) J. Charpentier, N. Früh and A. Togni, Chem. Rev., 2015, 115, 650–682 CrossRef CAS PubMed
.
- D. A. Nagib and D. W. C. MacMillan, Nature, 2011, 480, 224–228 CrossRef CAS PubMed
.
-
(a) Y. Itoh and K. Mikami, Org. Lett., 2005, 7, 4883–4885 CrossRef CAS PubMed
;
(b) P. V. Pham, D. A. Nagib and D. W. C. MacMillan, Angew. Chem., Int. Ed., 2011, 50, 6119–6122 CrossRef CAS PubMed
.
-
(a) Y.-b. Wu, G.-p. Lu, T. Yuan, Z.-b. Xu, L. Wan and C. Cai, Chem. Commun., 2016, 52, 13668–13670 RSC
;
(b) M. Oishi, H. Kondo and H. Amii, Chem. Commun., 2009, 1909–1911 RSC
.
- H. Guyon, H. Chachignon and D. Cahard, Beilstein J. Org. Chem., 2017, 13, 2764–2799 CrossRef CAS PubMed
.
- N. Shibata, A. Matsnev and D. Cahard, Beilstein J. Org. Chem., 2010, 6(65) DOI:10.3762/bjoc.6.65
.
- K. Sato, T. Yuki, R. Yamaguchi, T. Hamano, A. Tarui, M. Omote, I. Kumadaki and A. Ando, J. Org. Chem., 2009, 74, 3815–3819 CrossRef CAS PubMed
.
-
(a) L. Li, Q.-Y. Chen and Y. Guo, J. Org. Chem., 2014, 79, 5145–5152 CrossRef CAS PubMed
;
(b) K. Mikami, Y. Tomita, Y. Ichikawa, K. Amikura and Y. Itoh, Org. Lett., 2006, 8, 4671–4673 CrossRef CAS PubMed
.
-
(a) P. V. Pham, D. A. Nagib and D. W. C. MacMillan, Angew. Chem., Int. Ed., 2011, 50, 6119–6122 CrossRef CAS PubMed
;
(b) D. Cantillo, O. de Frutos, J. A. Rincón, C. Mateos and C. O. Kappe, Org. Lett., 2014, 16, 896–899 CrossRef CAS PubMed
.
- T. Kitazume and N. Ishikawa, J. Am. Chem. Soc., 1985, 107, 5186–5191 CrossRef CAS
.
- For selected examples, see:
(a) Y. Yang, Y. Liu, Y. Jiang, Y. Zhang and D. A. Vicic, J. Org. Chem., 2015, 80, 6639–6648 CrossRef CAS PubMed
;
(b) E. Yamaguchi, Y. Kamito, K. Matsuo, J. Ishihara and A. Itoh, Synthesis, 2018, 50, 3161–3168 CrossRef CAS
;
(c) Y.-b. Wu, G.-p. Lu, T. Yuan, Z.-b. Xu, L. Wan and C. Cai, Chem. Commun., 2016, 52, 13668–13670 RSC
;
(d) A. Deb, S. Manna, A. Modak, T. Patra, S. Maity and D. Maiti, Angew. Chem., Int. Ed., 2013, 52, 9747–9750 CrossRef CAS PubMed
.
-
(a) A. Maji, A. Hazra and D. Maiti, Org. Lett., 2014, 16, 4524–4527 CrossRef CAS PubMed
;
(b) Y. R. Malpani, B. K. Biswas, H. S. Han, Y.-S. Jung and S. B. Han, Org. Lett., 2018, 20, 1693–1697 CrossRef CAS PubMed
.
- For recent reviews on the applications of electrochemistry to organic synthesis, see:
(a) M. Yan, Y. Kawamata and P. S. Baran, Chem. Rev., 2017, 117, 13230–13319 CrossRef CAS PubMed
;
(b) P.-G. Echeverria, D. Delbrayelle, A. Letort, F. Nomertin, M. Perez and L. Petit, Aldrichimica Acta, 2018, 51, 3–19 Search PubMed
;
(c) S. R. Waldvogel, A. Wiebe, T. Gieshoff, S. Möhle, E. Rodrigo and M. Zirbes, Angew. Chem., Int. Ed., 2018, 57, 5594–5619 CrossRef PubMed
;
(d) S. R. Waldvogel and B. Janza, Angew. Chem., Int. Ed., 2014, 53, 7122–7123 CrossRef CAS PubMed
.
- E. J. Horn, B. R. Rosen and P. S. Baran, ACS Cent. Sci., 2016, 2, 302–308 CrossRef CAS PubMed
.
-
(a) R. N. Renaud and P. J. Champagne, Can. J. Chem., 1975, 53, 529–534 CrossRef CAS
;
(b) K. Arai, K. Watts and T. Wirth, ChemistryOpen, 2014, 3, 23–28 CrossRef CAS PubMed
.
- Many applications of electrochemical oxidation of CF3SO2Na for trifluoromethylation reactions have been recently reported, a strategy originally described by Langlois:
(a) B. R. Langlois, E. Laurent and N. Roidot, Tetrahedron Lett., 1991, 32, 7525–7528 CrossRef CAS
;
(b) J.-B. Tommasino, A. Brondes, M. Médebielle, M. Thomalla, B. R. Langlois and T. Billard, Synlett, 2002, 1697–1699 CrossRef CAS
.
- A. G. ÓBrien, A. Maruyama, Y. Inokuma, M. Fujita, P. S. Baran and D. G. Blackmond, Angew. Chem., Int. Ed., 2014, 53, 11868–11871 CrossRef PubMed
.
- W. Jud, C. O. Kappe and D. Cantillo, Chem. – Eur. J., 2018, 24, 17234–17238 CrossRef CAS PubMed
.
- Y. Zhao and D. G. Truhlar, Theor. Chem. Acc., 2008, 120, 215–241 Search PubMed
.
- A. V. Marenich, C. J. Cramer and D. G. Truhlar, J. Phys. Chem. B, 2009, 113, 6378–6396 CrossRef CAS PubMed
.
-
M. J. Frisch, G. W. Trucks, H. B. Schlegel, G. E. Scuseria, M. A. Robb, J. R. Cheeseman, G. Scalmani, V. Barone, B. Mennucci, G. A. Petersson, H. Nakatsuji, M. Caricato, X. Li, H. P. Hratchian, A. F. Izmaylov, J. Bloino, G. Zheng, J. L. Sonnenberg, M. Hada, M. Ehara, K. Toyota, R. Fukuda, J. Hasegawa, M. Ishida, T. Nakajima, Y. Honda, O. Kitao, H. Nakai, T. Vreven, J. A. Montgomery Jr., J. E. Peralta, F. Ogliaro, M. Bearpark, J. J. Heyd, E. Brothers, K. N. Kudin, V. N. Staroverov, R. Kobayashi, J. Normand, K. Raghavachari, A. Rendell, J. C. Burant, S. S. Iyengar, J. Tomasi, M. Cossi, N. Rega, J. M. Millam, M. Klene, J. E. Knox, J. B. Cross, V. Bakken, C. Adamo, J. Jaramillo, R. Gomperts, R. E. Stratmann, O. Yazyev, A. J. Austin, R. Cammi, C. Pomelli, J. W. Ochterski, R. L. Martin, K. Morokuma, V. G. Zakrzewski, G. A. Voth, P. Salvador, J. J. Dannenberg, S. Dapprich, A. D. Daniels, Ö. Farkas, J. B. Foresman, J. V. Ortiz, J. Cioslowski and D. J. Fox, Gaussian 09, Revision A.1, Gaussian, Inc., Wallingford CT, 2009 Search PubMed
.
Footnote |
† Electronic supplementary information (ESI) available: Supplementary figures and tables, copies of NMR spectra and Cartesian coordinates and energies of all calculated structures. See DOI: 10.1039/c9ob00456d |
|
This journal is © The Royal Society of Chemistry 2019 |