DOI:
10.1039/C9NR07190C
(Paper)
Nanoscale, 2019,
11, 20221-20227
The synergistic effect of Au-COF nanosheets and artificial peroxidase Au@ZIF-8(NiPd) rhombic dodecahedra for signal amplification for biomarker detection†
Received
21st August 2019
, Accepted 3rd October 2019
First published on 5th October 2019
Abstract
Here, a new type of signal amplification strategy is proposed employing Au nanoparticle (AuNP)-functionalized covalent organic framework (Au-COF) nanosheets and AuNP functionalized ZIF-8(NiPd) (Au@ZIF-8(NiPd)) rhombic dodecahedra nanocomposites for sandwich electrochemical sensor construction. The peroxidase mimics Au@ZIF-8(NiPd) took the place of natural enzymes in enzyme-assisted amplification strategies, both acting as catalysts for H2O2 reduction for signal amplification, and serving as ideal nanocarriers for signal probe anchoring. The cancer biomarker thrombin (TB) was selected as the target. Thrombin binding aptamers (TBA 2) were fixed on Au@ZIF-8(NiPd), and the obtained TBA 2–Au@ZIF-8(NiPd) bioconjugates were employed as tracer labels, and TB was sandwiched between the tracer labels and capture probe TBA 1 which were immobilized on the Au-COF nanosheet modified electrode. Au-COFs with a high specific area, super electroconductivity, and uniformly distributed AuNPs were utilized as the electrode substrate to fix TBA 1. Exploiting the sandwich method, the proposed TB aptasensor exhibited a wide linear range of 0.1 pM to 20 nM with a low detection limit of 15 fM (S/N = 3). The ingenious sensing strategy enriched the application diversity of the artificial enzyme and showed promise in research and development of point-of-care diagnostics.
Introduction
Thrombin (TB), as a serine protease, plays a decisive part in the blood coagulation process. It catalyzes the formation of insoluble fibrin from fibrinogen,1 and elicits essential cellular responses in inflammation, hemostasis and thrombosis, and blood vessel development.2 Since it relates closely to multiple diseases including thrombosis, central nervous system injury, Alzheimer's and cancer, TB is classified as a biomarker3,4 for early disease diagnosis. Thus, exploiting accurate detection methods to trace TB is of high priority.
Aptamers combined with various techniques such as colorimetry,5 surface-enhanced Raman scattering,6 and fluorescence7 have been extensively explored for TB determination. In contrast to conventional recognition element antibodies, aptamers enjoy obvious advantages in large scale production, as they are cost effective, and there are no restrictions of the detected targets.8 TBA can fold into an antiparallel chair-like G-quadruplex structure to bind with TB strongly and selectively.9 Particularly, the electrochemical method integrated with aptamers stands out as the most promising method due to its inherent advantages including high sensitivity, excellent selectivity, low cost, simple operation, fast response, and easy miniaturization.10–12 Accordingly, many efforts have been made to exploit electrochemical aptasensors for TB detection. To construct electrochemical aptasensors, two significant factors deserve consideration: (1) the high conductivity of the electrode materials, and (2) the sensitive and identifiable signal labels.
As for conductive electrode materials, covalent organic frameworks (COFs) have drawn our attention. COFs are a class of crystalline organic porous materials, constructed from organic molecular building units via covalent bonds.13,14 COFs have been extensively applied in gas adsorption and catalysis owing to their low mass densities, tunable pore sizes and shapes, high surface area, good thermal stability, and easy functionalization.15,16 Furthermore, the ordered crystalline structure makes COFs prospective substrate materials to immobilize nanoparticles (NPs),17 and the lamellar structure could accelerate the transport of charge carriers on account of periodic arrays of π clouds.18 Thus, COFs are appropriate for fabricating electrochemical biosensors. Nevertheless, despite enormous COFs being synthesized lately, the exploration of COFs on sensors is still in its infancy. Hence, it is intriguing to utilize NP modified COFs as electrode matrices, which would endow the sensors with high electroconductivity and favorable sensing performance.
Another central factor is the need for sensitive and identifiable signal labels to improve the sensitivity of electrochemical aptasensors. Typically enzyme-assisted amplification strategies are adopted such as enzyme-catalyzed reactions19 and enzyme-assisted nucleic acid amplification technologies.20 However, these techniques involve complex operation procedures, extra exogenous reagents and enzymes, which definitely increases the complexity of the experiment, detection time and cost. Moreover, the enzymes have intrinsic disadvantages of vulnerable catalytic activity and low operational stability,21,22 which further hinder the applications of enzyme-assisted aptasensors. To conquer these drawbacks, efforts have been devoted to replacing natural enzymes with artificial enzyme mimics for enzyme-assisted reactions. Metal–organic frameworks (MOFs), self-assembled by metal ions and organic ligands, have attracted widespread interest as enzyme mimics thanks to their uniform and tunable pore sizes, high stability, and huge and accessible specific surface areas.23–25 Diverse MOFs have been reported to be valid enzyme mimics with biological functions, which extends their applications in biocatalysis, bioanalysis, and biomedical engineering.26,27 Specifically, a zeolitic imidazolate framework 8 (ZIF-8) encapsulated within NiPd hollow NPs (NiPdhNPs) was found to have intrinsic peroxidase-like activity with superb stability and a high specific surface area.28 ZIF-8 can stabilize the incorporated NPs to retain high catalytic activity. More importantly, the uniform pore size also provided a molecular sieving effect to achieve selectivity of the reactant. Until now, reports on utilizing MOF-based nanozymes as nanocarriers for sandwich electrochemical aptasensors are rare. Employing a ZIF-8 matrix to assemble NPs as peroxidase mimic catalysts for signal amplification would be a fascinating research subject.
Herein, a simple and ultrasensitive sandwich electrochemical TB aptasensor is ingeniously designed and fabricated. The peroxidase mimic Au@ZIF-8(NiPd) with superb catalytic performance and a highly porous structure was synthesized for the first time. Using H2O2 as the signal molecule, Au@ZIF-8(NiPd) offered the microenvironment for H2O2 preconcentration and promoted H2O2 reduction, leading to signal amplification. Besides, Au@ZIF-8(NiPd) with a large surface area provided numerous active sites for TBA 2 immobilization, enhancing the sensing sensitivity and selectivity. Au-COFs with a high specific area, super electroconductivity, and uniformly distributed AuNPs were employed as the substrate to assemble TBA 1, and this was the first time that Au-COFs were exploited to fabricate an electrochemical aptasensor. With the synergetic effect of the nanocarrier Au@ZIF-8(NiPd) and electrode matrix Au-COFs, the aptasensor exhibited high sensitivity and selectivity, and it can sense TB in human serum. This strategy promoted the applications of artificial enzymes in electrochemical sensors, and holds promise in the research and development of point-of-care diagnostics.
Experimental
Synthesis of COF nanosheets
COF nanosheets were synthesized referring to the literature procedure with some modifications (Scheme S1†).29 0.5 g BDBA and 20 g of a 1,4-dioxane–mesitylene mixture (1
:
1, v/v) were homogeneously mixed by stirring for 1 h, and the mixture was transferred to a 20 mL Teflon-lined autoclave to react at 120 °C for 72 h. Then, the pristine COFs were filtered and washed with 1,4-dioxane, and then dried under vacuum conditions. COF powders were dissolved in ethanol (2 mg mL−1) and sonicated for 1 h to prepare exfoliated COF nanosheets.
Synthesis of NiPdNPs, ZIF-8(NiPd), and Au@ZIF-8(NiPd)
NiPdNPs were prepared referring to the literature procedure with minor modifications.30 Typically, 25.8 mg trisodium citrate and 6.5 mg NiCl2 were successively dissolved in 50 mL H2O by stirring. Then, the mixture was bubbled with N2 for 30 min to remove dissolved O2. After that, 5 mL freshly prepared NaBH4 solution (2 mg mL−1) was added slowly drop-by-drop into the solution under vigorous stirring. Then, 2.5 mL H2PdCl4 (8.48 mM) was added dropwise into the mixed solution, and stirred for 10 min. Finally, NiPdNPs were washed by centrifugation, re-dispersed in 5 mL water, and preserved at 4 °C.
ZIF-8(NiPd) was constructed according to the literature procedure with some modifications.31 45 mL Zn(NO3)2 (50 mM) methanol solution and 45 mL 2-methylimidazole (50 mM) methanol solution were mixed uniformly by stirring. Next, 1 mL NiPdNP solution was added and stirred to obtain a homogeneous solution. Then, the mixed solution was kept undisturbed at room temperature for 24 h. Finally, ZIF-8(NiPd) was acquired via centrifugation with methanol, and dried under vacuum conditions.
Au@ZIF-8(NiPd) was prepared via the NaBH4 reduction method. 1 mL HAuCl4 (w/w, 1%) solution was dropped into 1 mL (1 mg mL−1) ZIF-8(NiPd) solution under stirring. Then, 1 mL freshly prepared NaBH4 solution (2 mg mL−1) was added dropwise into the mixed solution and stirred for 40 min. After that, the mixture was centrifuged with H2O and dried under vacuum conditions to obtain Au@ZIF-8(NiPd).
Preparation of TBA 2–Au@ZIF-8(NiPd) (tracer labels)
The preparation of tracer labels is displayed in Scheme 1A. First, 100 μL TBA (2.0 μM) solution was added into 2 mL (1 mg mL−1) Au@ZIF-8(NiPd) solution and gently stirred for 16 h at 4 °C. TBA was attached onto Au@ZIF-8(NiPd) by the interactions between –NH2 and AuNPs. Then, 50 μL of BSA (w/w, 1%) was dropped into the mixture and stirred for 1 h to block the remaining active sites. After centrifugation, the obtained product was suspended in 2 mL PBS (10 mM, pH 7.0) and preserved at 4 °C.
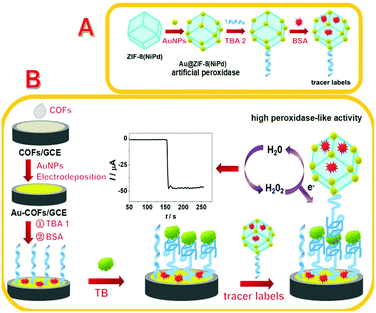 |
| Scheme 1 Schematic illustration of the process for the synthesis of tracer labels (A) and the construction of the aptasensor (B). | |
Construction of the aptasensor
The construction process of the aptasensor is shown in Scheme 1B. GCE was polished respectively using 0.1 μm and 0.05 μm alumina slurries, and then rinsed ultrasonically with nitric acid/water (1/1, v/v), absolute alcohol, and ultrapure water separately to achieve a mirror-like surface and dried under N2. 10 μL of COF nanosheet solution was dropped onto the GCE surface and dried at room temperature to obtain COFs/GCE. Then, the COFs/GCE was soaked in HAuCl4 solution (w/w, 1%) for electro-deposition at −0.2 V for 40 s, hence, Au-COFs/GCE was prepared. After that, 20 μL TBA 1 (2.0 μM) was dropped onto the Au-COFs/GCE and incubated at room temperature for 14 h to acquire TBA 1/Au-COFs/GCE. Subsequently, the electrode was further incubated with 10 μL BSA solution (w/w, 1%) for 40 min to block the remaining active sites, and then rinsed thoroughly with water. Finally, the BSA/TBA 1/Au-COFs/GCE was prepared. As for TB detection, the prepared electrode was incubated with different concentrations of TB for 60 min at room temperature, and then 20 μL of tracer labels was cast onto the prepared electrode and incubated for another 60 min. After rinsing, the electrode was used for electrochemical measurements.
Peroxidase-like activity of Au@ZIF-8(NiPd)
The peroxidase-like activity of Au@ZIF-8(NiPd) was investigated employing TMB, ABTS, and OPD as the chromogenic substrates. 10 μL nanohybrids (1 mg mL−1) were dropped into 3.0 mL PBS (10 mM, pH = 5.0) consisting of 0.25 mM chromogenic substrate and 2 mM H2O2. The mixture was incubated at 50 °C for 20 min, and then utilized for UV–vis detection.
Results and discussion
Characterization of the COFs and Au-COFs
SEM and TEM measurements were carried out to explore the morphology and structure of the synthesized COFs and Au-COFs. As shown in Fig. 1, pristine COFs showed a typical lamellar structure with terraced texture at the edge. After sonication treatment, the pristine COFs were transformed into flat COF nanosheets with a monolayer or a few layers. The evidence of the formation of COFs was also provided by FTIR and XRD. The FTIR spectra of pristine COFs and COF nanosheets demonstrated almost the same spectra with the formation of respective boroxine and boronate ester rings,32 indicating that there was no degradation of the skeletal structure of COFs after the sonication treatment. And the characteristic peaks of the COF nanosheets in the XRD patterns were consistent with the reported data,32,33 indicating the successful formation of COF nanosheets. Fig. 1f shows the TEM image of Au-COF nanosheets, and as is shown, AuNPs were uniformly anchored onto the COF nanosheets, and no agglomeration was observed. Thus, the Au-COF nanosheets were successfully formed.
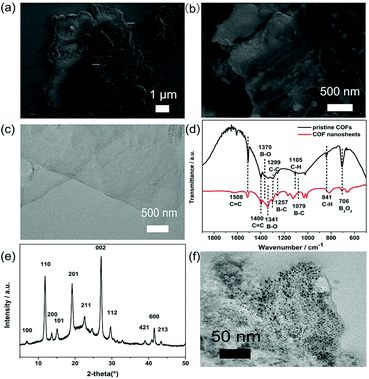 |
| Fig. 1 (a) and (b) SEM images of pristine COFs; (c) TEM image of COF nanosheets; (d) FTIR spectra of pristine COF and COF nanosheets; (e) XRD patterns of COF nanosheets; (f) TEM image of Au-COF nanosheets. | |
Characterization of Au@ZIF-8(NiPd) and tracer labels
SEM and TEM characterized the morphology of the samples. As displayed in Fig. 2a, homogeneous NiPdNPs were well dispersed with an average size of 2.67 nm (Fig. S1a†). The high-resolution TEM (HRTEM) image of NiPdNPs exhibited crystalline nature with a lattice spacing of 0.22 nm, which was ascribed to the (111) planes of face-centered cubic (fcc)-type Ni (0.203 nm)34 and Pd (0.224 nm).35,36
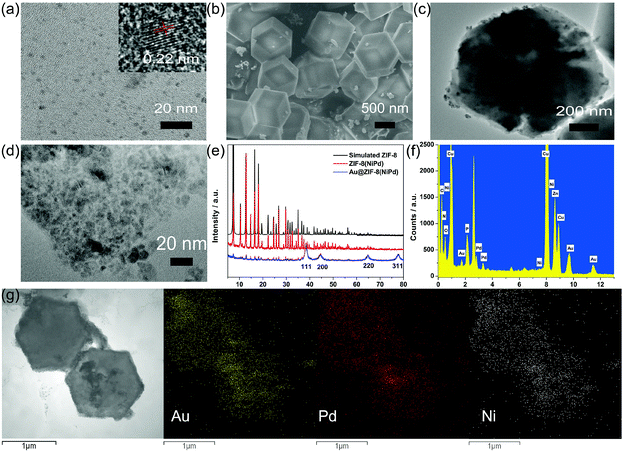 |
| Fig. 2 (a) TEM image of NiPdNPs (inset: HRTEM image of NiPdNPs); (b) SEM image, and (c) and (d) TEM images of Au@ZIF-8(NiPd); (e) XRD patterns of simulated ZIF-8, ZIF-8(NiPd), and Au@ZIF-8(NiPd); (f) EDX spectra of tracer labels; (g) STEM-EDS elemental mapping images of the tracer labels. | |
The SEM image of ZIF-8(NiPd) (Fig. S1†) shows a smooth and uniform rhombic dodecahedral shape, and the TEM image further proved that NiPdNPs were uniformly distributed inside the ZIF-8. After the in situ reduction of AuNPs, the obtained Au@ZIF-8(NiPd) maintained the rhombic dodecahedral structure with a relatively unsmooth surface. Fig. 2d shows the amplified TEM image of the Au@ZIF-8(NiPd) surface, and as is shown, a large amount of AuNPs were observed, providing a massive number of active sites for TBA anchoring.
XRD was also applied to confirm the synthesis of Au@ZIF-8(NiPd). As shown in Fig. 2e, ZIF-8(NiPd) exhibited identical diffraction peaks of simulated ZIF-8, revealing that the encapsulation of NiPdNPs had an insignificant influence on the phase of ZIF-8 hosts. Besides, the predominant XRD patterns of ZIF-8 were preserved in Au@ZIF-8(NiPd) with the appearance of four intense diffraction peaks located at 38.4°, 44.8°, 64.9°, and 77.9°, which were attributed to the (111), (200), (220), and (311) planes of fcc-type Au.34 Thus, Au@ZIF-8(NiPd) was successfully prepared.
In addition, STEM-EDS elemental mapping images of rhombic dodecahedral TBA 2–Au@ZIF-8(NiPd) (tracer labels) further confirmed the uniform distribution of NiPdNPs inside the ZIF-8 and AuNPs over ZIF-8. The EDX spectra (Fig. 2f) of the tracer labels affirmed the existence of element P that originated from the surface-immobilized aptamers, leading to the fabrication of tracer labels.
The peroxidase-like activity of Au@ZIF-8(NiPd) was investigated by catalysis of the chromogenic peroxidase substrate TMB, ABTS, and OPD. As shown in Fig. 3a, Au@ZIF-8(NiPd) catalytically oxidized TMB, ABTS, and OPD to produce different colors in the presence of H2O2, indicating its peroxidase-like activity. Further comparison of the peroxidase-like activity of different catalysts was conducted using OPD as the chromogenic substrate. As shown in Fig. 3b, OPD was catalytically oxidized by NiPdNPs, ZIF-8(NiPd) and Au@ZIF-8(NiPd) in the presence of H2O2 with the change in color to orange. In contrast, ZIF-8 displayed negligible color change with a poor peroxidase-like activity. Compared with NiPdNPs, ZIF-8(NiPd) showed relatively inferior catalytic activity because as the concentrations of the catalysts were the same, the content of NiPdNPs in ZIF-8(NiPd) was less. Interestingly, Au@ZIF-8(NiPd) demonstrated much enhanced enzymatic activity of the mimic with high absorption at 425 nm, which is attributed to the synergistic effect between the AuNPs and ZIF-8(NiPd). In addition, the OPD solution and the OPD + H2O2 solution presented an insignificant color change, suggesting that the color change was relevant to the high mimetic peroxidase activity of Au@ZIF-8(NiPd). The enhanced catalytic activity can contribute to the detection of the target at a low concentration.
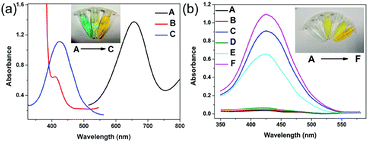 |
| Fig. 3 (a) Au@ZIF-8(NiPd) catalyzed oxidation of various substrates to produce different colors in the presence of H2O2: (A) TMB, (B) ABTS, and (C) OPD, respectively. Inset: The corresponding photograph of different substances. (b) The absorption spectra and digital photos of different reaction systems: (A) OPD; (B) OPD + H2O2; (C) OPD + H2O2 + NiPdNPs; (D) OPD + H2O2 + ZIF-8; (E) OPD + H2O2 + ZIF-8(NiPd); (F) OPD + H2O2 + Au@ZIF-8(NiPd). | |
Electrochemical characterization of the stepwise-modified electrodes
The fabrication process of the aptasensor was observed by electrochemical impedance spectroscopy (EIS) and cyclic voltammetry (CV) measurements. As shown in Fig. 4a, the results of EIS were fitted with the Randles electronic equivalent circuit. The semicircle portion at higher frequencies is related to an electron-transfer limited process, while the linear portion at lower frequencies is related to a diffusion limited process.37 The diameter of the semicircle represents the charge transfer resistance (Rct). In comparison with a bare GCE, the COFs/GCE showed a distinctly increased Rct due to the relatively inferior electroconductivity of COF nanosheets. After the electrodeposition of AuNPs, the Rct of the Au-COFs/GCE decreased evidently. COF nanosheets provided an ideal platform for the anchoring of AuNPs, and thus accelerated the electron transfer and reduced the resistance. When TBA 1 was introduced, the Rct increased because the negatively charged phosphate skeletons in TBA 1 repulsed the [Fe(CN)6]3−/4− capture probe. Afterwards, a successive increase of Rct was observed when BSA was dropped onto the electrode since BSA can hinder electron transfer. Eventually, when the electrode was incubated with TB, the Rct was further improved, suggesting that TB was successfully captured by TBA 1. The related CV curves were in accord with the EIS results, further proving the successful construction of the proposed aptasensor.
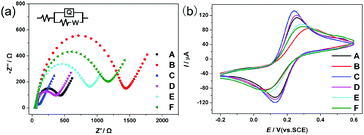 |
| Fig. 4 (a) EIS and (b) CV curves of different electrodes: (A) GCE; (B) COFs/GCE; (C) Au-COFs/GCE; (D) TBA 1/Au-COFs/GCE; (E) BSA/TBA 1/Au-COFs/GCE; (F) TB/BSA/TBA 1/Au-COFs/GCE in PBS (10 mM, pH 7.0) containing 5 mM [Fe(CN)6]3−/4− and 0.1 M KCl. The electrode was incubated with 10 nM TB for 40 min. The frequency ranged from 105 to 0.01 Hz with 5 mV amplitude. The equivalent circuit was inserted. | |
Optimization of the detection conditions
The experimental conditions including the volume of the COF nanosheet suspension (2 mg mL−1), the deposition time of AuNPs, the incubation time of TB, the volume of tracer labels, and the concentration of H2O2 were investigated to achieve high electrochemical performance of the aptasensor, and 10 μL of COF suspension, 40 s of electrodeposition time, 60 min of TB incubation time, 20 μL of tracer labels, and 5 mM H2O2 were selected as the optimum conditions.
Performance of the aptasensor
The analytical performance of the proposed aptasensor was appraised by amperometric measurement under the optimal conditions, and the results are shown in Fig. 5. As is shown, the current responses increased with the increasing concentrations of TB, and the current changes varied linearly with the logarithm of TB concentrations in the range of 0.1 pM to 20 nM with a low detection limit of 15 fM (S/N = 3). Moreover, a comparison with previously reported aptasensors is summarized in Table S2.† The proposed aptasensor had a lower detection limit and a wider linear range. The exceptional performance was attributed to the following factors: (1) Au-COFs with a high specific area, super electroconductivity, and uniformly distributed AuNPs provided numerous active sites for TBA 1 anchoring to amplify the electrochemical signal; (2) Au@ZIF-8(NiPd) possessed a porous structure and high peroxidase catalytic activity, which contributed to the preconcentration of the signal molecule H2O2 and promoted the reduction of H2O2, leading to signal amplification; (3) the nanocarrier Au@ZIF-8(NiPd) provided a large specific surface area to which large amounts of TBA 2 can be fixed, which further promoted the electrochemical performance and improved sensing sensitivity and selectivity.
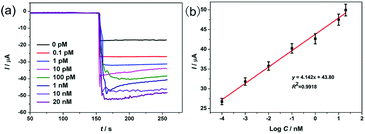 |
| Fig. 5 (a) Amperometric i–t curves of the aptasensor at −0.40 V at different concentrations of TB. (b) Calibration curve of current versus logarithm of TB concentrations. | |
Selectivity, reproducibility, and stability of the aptasensor
The selectivity of the aptasensor was assessed and is shown in Fig. 6. As is shown, the electrochemical response caused by 100 nM bovine serum albumin (BSA), lysozyme (Lys), and human IgG did not change much compared with the blank signal (0 pM TB). When the electrode was incubated with a mixture containing 10 nM BSA, Lys, IgG, and TB, the current response was approximate to the response caused by only 10 nM TB, indicating good selectivity of the aptasensor.
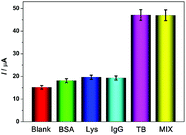 |
| Fig. 6 Selectivity of the proposed TB aptasensor. | |
The reproducibility of the aptasensor was evaluated using five independently prepared aptasensors; the relative standard derivation (RSD) of current responses was 4.96%, demonstrating that the aptasensor shows good reproducibility.
To explore the stability of the aptasensor, the as-prepared aptasensors were stored at 4 °C for 10 days and tested every day, and it was found that 86.6% of the original electrochemical signal was maintained. Thus, the stability of the aptasensor was satisfactory.
Application in real samples
Different concentrations of TB were injected into 10-fold diluted human serum samples to test the applicability of the aptasensor. A standard addition method was employed. As shown in Table 1, the recovery was from 91.4 to 104.9%, suggesting the potential application prospect of the aptasensor.
Table 1 Detection of TB in serum samples
Sample |
Added (nM) |
Founda (nM) |
RSD (%) |
Recovery (%) |
Average of five determinations.
|
Serum 1 |
0.01 |
0.0103 |
4.17 |
103.0 |
Serum 2 |
0.1 |
0.0914 |
3.22 |
91.4 |
Serum 3 |
1.0 |
1.049 |
5.69 |
104.9 |
Conclusions
In summary, a sensitive electrochemical sandwich aptasensor for TB detection was designed and constructed utilizing Au-COFs as the conductive matrix and Au@ZIF-8(NiPd) as nanocarriers. The Au-COFs could achieve signal amplification due to their super electroconductivity and high amount of TBA 1 anchoring. And the high peroxidase catalysis activity and loading capacity of Au@ZIF-8(NiPd) further promoted sensing sensitivity and selectivity. Based on this, the aptasensor showed huge potential applications in the research and development of point-of-care diagnostics. More importantly, this work expanded the application of a nanozyme to electrochemical sensors.
Conflicts of interest
There are no conflicts to declare.
Acknowledgements
This work acknowledges the support from the Natural Science Foundation of Jilin Province (No. 20180101195JC).
Notes and references
- D. M. Tasset, M. F. Kubik and W. Steiner, J. Mol. Biol., 1997, 272, 688–698 CrossRef CAS PubMed.
- S. R. Coughlin, Nature, 2000, 407, 258 CrossRef CAS PubMed.
- M. Franchini and P. M. Mannucci, Semin. Thromb. Hemostasis, 2012, 38, 95–101 CrossRef CAS PubMed.
- A. Nishino, M. Suzuki, H. Ohtani, O. Motohashi, K. Umezawa, H. Nagura and T. Yoshimoto, J. Neurotrauma, 1993, 10, 167–179 CrossRef CAS PubMed.
- G. Liang, S. Cai, P. Zhang, Y. Peng, H. Chen, S. Zhang and J. Kong, Anal. Chim. Acta, 2011, 689, 243–249 CrossRef CAS PubMed.
- A. R. Bizzarri and S. Cannistraro, Anal. Biochem., 2009, 393, 149–154 CrossRef CAS PubMed.
- H. Chang, L. Tang, Y. Wang, J. Jiang and J. Li, Anal. Chem., 2010, 82, 2341–2346 CrossRef CAS PubMed.
- Z. Zhang, Z. Wang, X. Wang and X. Yang, Sens. Actuators, B, 2010, 147, 428–433 CrossRef CAS.
- A. Merlino, A. Randazzo, E. Novellino, I. Russo Krauss, L. Mazzarella and F. Sica, Nucleic Acids Res., 2012, 40, 8119–8128 CrossRef PubMed.
- F. Gao, L. Du, Y. Zhang, F. Zhou and D. Tang, Biosens. Bioelectron., 2016, 86, 185–193 CrossRef CAS.
- J. Yang, B. Dou, R. Yuan and Y. Xiang, Anal. Chem., 2016, 88, 8218–8223 CrossRef CAS PubMed.
- A. B. Hashkavayi and J. B. Raoof, Biosens. Bioelectron., 2017, 91, 650–657 CrossRef CAS PubMed.
- J. W. Colson, A. R. Woll, A. Mukherjee, M. P. Levendorf, E. L. Spitler, V. B. Shields, M. G. Spencer, J. Park and W. R. Dichtel, Science, 2011, 332, 228–231 CrossRef CAS PubMed.
- X. Feng, X. Ding and D. Jiang, Chem. Soc. Rev., 2012, 41, 6010–6022 RSC.
- S. S. Han, J. L. Mendoza-Cortés and W. A. Goddard III, Chem. Soc. Rev., 2009, 38, 1460–1476 RSC.
- H. Xu, J. Gao and D. Jiang, Nat. Chem., 2015, 7, 905 CrossRef CAS PubMed.
- S.-Y. Ding, J. Gao, Q. Wang, Y. Zhang, W.-G. Song, C.-Y. Su and W. Wang, J. Am. Chem. Soc., 2011, 133, 19816–19822 CrossRef CAS PubMed.
- Q. Fang, Z. Zhuang, S. Gu, R. B. Kaspar, J. Zheng, J. Wang, S. Qiu and Y. Yan, Nat. Commun., 2014, 5, 4503 CrossRef PubMed.
- N. Xia, Y. Zhang, X. Wei, Y. Huang and L. Liu, Anal. Chim. Acta, 2015, 878, 95–101 CrossRef CAS PubMed.
- M. Liu, J. Song, S. Shuang, C. Dong, J. D. Brennan and Y. Li, ACS Nano, 2014, 8, 5564–5573 CrossRef CAS PubMed.
- F. Meng, X. Yan, J. Liu, J. Gu and Z. Zou, Electrochim. Acta, 2011, 56, 4657–4662 CrossRef CAS.
- Y. Li, Q. Lu, S. Wu, L. Wang and X. Shi, Biosens. Bioelectron., 2013, 41, 576–581 CrossRef CAS PubMed.
- H. Furukawa, K. E. Cordova, M. O'Keeffe and O. M. Yaghi, Science, 2013, 341, 1230444 CrossRef PubMed.
- J.-D. Xiao, Q. Shang, Y. Xiong, Q. Zhang, Y. Luo, S.-H. Yu and H.-L. Jiang, Angew. Chem., Int. Ed., 2016, 55, 9389–9393 CrossRef CAS PubMed.
- M. Maes, L. Alaerts, F. Vermoortele, R. Ameloot, S. Couck, V. Finsy, J. F. M. Denayer and D. E. De Vos, J. Am. Chem. Soc., 2010, 132, 2284–2292 CrossRef CAS PubMed.
- Y. Hu, H. Cheng, X. Zhao, J. Wu, F. Muhammad, S. Lin, J. He, L. Zhou, C. Zhang, Y. Deng, P. Wang, Z. Zhou, S. Nie and H. Wei, ACS Nano, 2017, 11, 5558–5566 CrossRef CAS PubMed.
- J. S. Kahn, L. Freage, N. Enkin, M. A. A. Garcia and I. Willner, Adv. Mater., 2017, 29, 1602782 CrossRef PubMed.
- Q. Wang, X. Zhang, L. Huang, Z. Zhang and S. Dong, Angew. Chem., Int. Ed., 2017, 56, 16082–16085 CrossRef CAS PubMed.
- G. Li, K. Zhang and T. Tsuru, ACS Appl. Mater. Interfaces, 2017, 9, 8433–8436 CrossRef CAS PubMed.
- Q. Wang, L. Zhang, C. Shang, Z. Zhang and S. Dong, Chem. Commun., 2016, 52, 5410–5413 RSC.
- F. Meng, S. Zhang, L. Ma, W. Zhang, M. Li, T. Wu, H. Li, T. Zhang, X. Lu, F. Huo and J. Lu, Adv. Mater., 2018, 30, 1803263 CrossRef PubMed.
- A. P. Côté, A. I. Benin, N. W. Ockwig, M. O'Keeffe, A. J. Matzger and O. M. Yaghi, Science, 2005, 310, 1166–1170 CrossRef PubMed.
- J. Sun, A. Klechikov, C. Moise, M. Prodana, M. Enachescu and A. V. Talyzin, Angew. Chem., Int. Ed., 2018, 57, 1034–1038 CrossRef CAS PubMed.
- D. Wang and Y. Li, J. Am. Chem. Soc., 2010, 132, 6280–6281 CrossRef CAS PubMed.
- Z.-L. Wang, H.-L. Wang, J.-M. Yan, Y. Ping, S.-I. O, S.-J. Li and Q. Jiang, Chem. Commun., 2014, 50, 2732–2734 RSC.
- J.-M. Yan, S.-J. Li, S.-S. Yi, B.-R. Wulan, W.-T. Zheng and Q. Jiang, Adv. Mater., 2018, 30, 1703038 CrossRef PubMed.
- M. Ciureanu and H. Wang, J. Electrochem. Soc., 1999, 146, 4031–4040 CrossRef CAS.
Footnote |
† Electronic supplementary information (ESI) available. See DOI: 10.1039/c9nr07190c |
|
This journal is © The Royal Society of Chemistry 2019 |
Click here to see how this site uses Cookies. View our privacy policy here.