DOI:
10.1039/C9NR05367K
(Review Article)
Nanoscale, 2019,
11, 19636-19657
Surface coatings for solid-state nanopores
Received
25th June 2019
, Accepted 15th September 2019
First published on 11th October 2019
Abstract
Since their introduction in 2001, solid-state nanopores have been increasingly exploited for the detection and characterization of biomolecules ranging from single DNA strands to protein complexes. A major factor that enables the application of nanopores to the analysis and characterization of a broad range of macromolecules is the preparation of coatings on the pore wall to either prevent non-specific adhesion of molecules or to facilitate specific interactions of molecules of interest within the pore. Surface coatings can therefore be useful to minimize clogging of nanopores or to increase the residence time of target analytes in the pore. This review article describes various coatings and their utility for changing pore diameters, increasing the stability of nanopores, reducing non-specific interactions, manipulating surface charges, enabling interactions with specific target molecules, and reducing the noise of current recordings through nanopores. We compare the coating methods with respect to the ease of preparing the coating, the stability of the coating and the requirement for specialized equipment to prepare the coating.
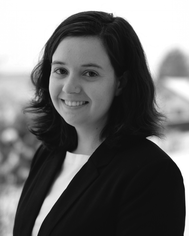 Olivia M. Eggenberger | Olivia Eggenberger is a Ph.D. student at the Adolphe Merkle Institute at the University of Fribourg in the Biophysics group of Prof. Michael Mayer. She received her undergraduate degree from the Department of Physics at Albion College in 2013 and a Master of Science from the Department of Biomedical Engineering at the University of Michigan in 2015. Her research focuses on single protein characterization using lipid-coated synthetic nanopores. |
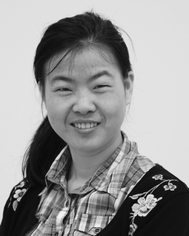 Cuifeng Ying | Cuifeng Ying received her Ph.D in Physics in 2013 from Nankai University in the group of Prof. Jianguo Tian. She is currently working as a postdoctoral researcher at the Adolphe Merkle Institute at the University of Fribourg in the Biophysics group of Prof. Michael Mayer. Her research focuses on characterizing single proteins using nanoplasmonic optical measurements and nanopore technology. |
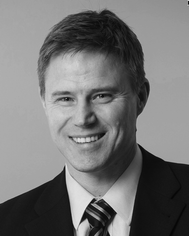 Michael Mayer | Michael Mayer obtained a Ph.D. in biophysical chemistry with Horst Vogel at the Swiss Federal Institute of Technology in Lausanne (EPFL), followed by postdoctoral research with George M. Whitesides at Harvard University. In 2004, he started a faculty position in Biomedical Engineering at the University of Michigan. In 2015 his group moved to the Adolphe Merkle Institute at the University of Fribourg, where he holds the chair of Biophysics. His research takes inspiration from nature to solve problems in biophysics ranging from understanding signaling and transport processes in biological membranes to characterizing protein complexes relevant for neurodegenerative diseases and to engineering biocompatible electrical power sources. |
Introduction
In the past two decades, nanopore-based analysis of single biomolecules or nanoparticles has undergone rapid development for the detection and characterization of DNA, proteins, viruses and synthetic nanoparticles.1–13 Recent advancements include the development of the portable MinION device for DNA sequencing with protein nanopores,14,15 the combination of nanopore recordings with additional modalities for sensing, characterizing, or manipulating molecules such as detecting fluorescent molecules based on plasmonic effects,16–22 recording changes in the local voltage of a graphene nanoribbon transistor,23 or pulling on or holding molecules in a nanopore with optical tweezers.24,25
In most cases, the basic experimental setup to detect and characterize single molecules in nanopores comprises two compartments of electrolyte solution, a thin insulating membrane that separates these compartments, and a single pore with a diameter ranging from 1–50 nm that constitutes the only connection between the two compartments (Fig. 1A). When an electric potential difference is applied across the membrane, molecules move through the electrolyte-filled pore and cause a change in the resistance of the pore by displacement of ions (Fig. 1B). The resulting resistive pulses that coincide with the translocation of individual particles reveal characteristics of that molecule. For instance, the most probable dwell time of the resistive pulse is inversely proportional to the charge of the molecule, while the amplitude of the resistive pulse is related to the volume, shape, and orientation of the molecule in the electric field (Fig. 1B).26
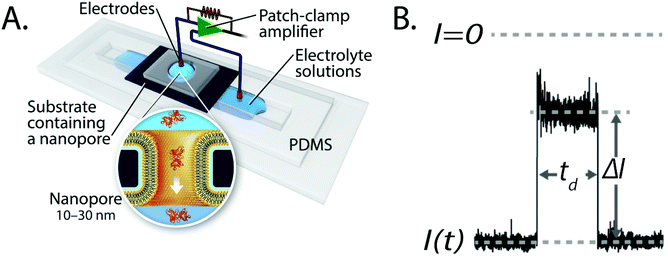 |
| Fig. 1 Basic principles of resistive pulse recordings through nanopores. A. Experimental set-up for resistive pulse recordings using an electrolyte-filled nanopore; the inset shows the translocation of a single protein through a nanopore with a lipid–bilayer coating.27 Figure from Yusko et al.27 B. Example of a resistive pulse; the change in current, ΔI, is proportional to particle volume and the dwell time, td, is inversely proportional to particle charge. Time-dependent modulations of the current during a resistive pulse can reflect the orientation of non-spherical particles and reveal their shape, rotational diffusion coefficient and dipole moment.27 | |
There are two major types of nanopores: biological nanopores28–32 and synthetic, solid-state nanopores.3,8,33 Biological nanopores consist of transmembrane proteins that enable the translocation of molecules through their lumen.34,35 The most widely used example of this type of nanopore is the α-hemolysin protein that is expressed by Staphylococcus aureus and self-incorporates into lipid membranes.28,36 The narrowest constriction of protein pores is relatively small; their diameters typically range from 0.4 nm–3.4 nm.5,6,28,37,38 These constrictions enable resistive-pulse recordings with high signal-to-noise ratio, they make protein pores attractive for the detection of analytes with at least one small dimension such as ions,39 organic molecules,40 peptides and unfolded proteins,41 as well as for sequencing of DNA and RNA.42–46 Other attractive features of these pores for biophysics and biosensing applications are the availability of crystal structures of several of these pore proteins with atomic resolution,36,47–52 their evolved resistance to clogging,29 their amenability to site-specific chemical modifications,53,54 and the excellent reproducibility of producing these pores by established protein expression and purification methods.28 Biological nanopores have, however, three main limitations: first, their small diameters prevent the ability to characterize large molecules. Second, their intrinsic fragility can lead to fluctuations of the baseline current through these pores under certain conditions such as elevated applied potential differences or elevated temperature.6,29 And third, the requirement to reconstitute these proteins into a lipid bilayer or polymer membrane poses the challenge to prepare a stable lipid or polymer membrane for each experiment, and to reconstitute protein pores into these membranes efficiently55 before each experiment.
The lack of large-scale tunability of the diameter of biological nanopore provided one of the motivations for the development of synthetic nanopores. Synthetic nanopores can be fabricated in virtually any size from below 1 nm in diameter56 to the sub—micrometer range.57,58 These sizes allow for the analysis of a large range of biomolecules including proteins, viruses, and nanoparticles.42,59,60 Nanoscale pores or channels in solid state materials are fabricated by various techniques61–66 including ion beam sculpting,56 focused ion beam fabrication,67 transmission electron microscopy,68 electron beam fabrication,69–73 track-etching,74–78 dielectric break down,79,80 laser-assisted dielectric breakdown,80 laser-assisted etching,80–82 and layer-by-layer removal.83 These techniques make it possible to create nanopores with varying shapes84 and surface chemistries85 in a range of materials including silicon nitride,86,87 silicon dioxide,68 hafnium oxide,88 aluminum oxide,89 graphene,90,91 glass92–94 and polymer films.95,96
One major limitation of solid-state nanopores is the tendency of their walls to interact non-specifically with many analytes. These interactions can lead to clogging of the pores and to the inability to translocate additional molecules. In this context, proteins, which constitute an increasingly common analyte in nanopore-based biophysics studies, are particularly prone to interact with the walls of synthetic nanopores.97 Factors contributing to these interactions include electrostatic attraction,98–100 van der Waals forces,101–103 and hydrophobic interactions.97,104–106 Surface coatings such as those shown in Fig. 2 can help to reduce the strength of these interactions and thereby allow for unperturbed translocation.107
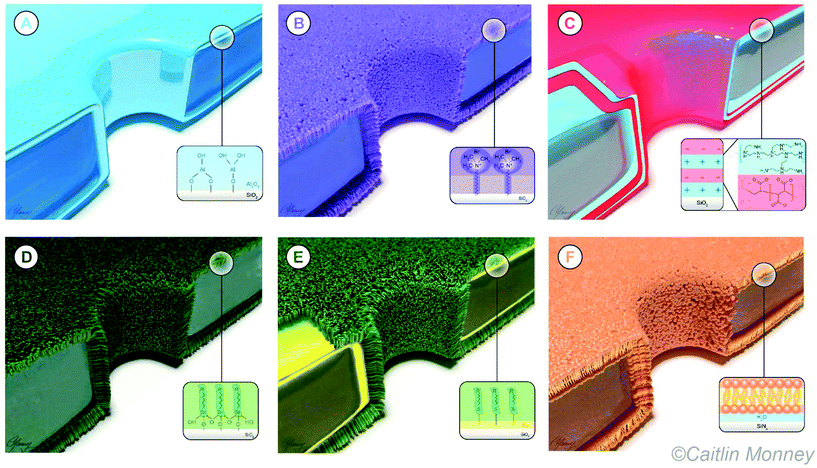 |
| Fig. 2 Idealized cartoon representations of the most commonly employed nanopore coatings. A. Cross-section through a SiO2 membrane with a coating of Al2O3 deposited on the membrane and on the walls of the nanopore. B. Coating of a nanopore prepared by physisorption of a surfactant. C. Coating prepared by layer-by-layer self-assembly of negatively and positively charge polymers. D. Coating prepared by silanization. E. Coating of a self-assembled monolayer of alkanethiols on gold. F. Coating of a nanopore with a fluid lipid bilayer. | |
The negatively charged surfaces on the walls of most synthetic nanopores also lead to two phenomena that are relevant for nanopore-based analyses: electroosmotic flow (EOF) and ion current rectification (ICR). Charged surfaces in contact with a liquid electrolyte accumulate a layer of counter-ions forming an electrical double layer (EDL).108 When a potential is applied that drops along the length of a nanopore, it drives electrophoretic movement of ions in the EDL creating EOF whereby the liquid moves with the ions.109–111 Electroosmotic flow provides an additional force on molecules inside the nanopore, which can either add to the electrophoretic force or point in the opposite direction, depending on the net charge of the molecule and on the polarity of the charges on the nanopore wall.112,113 In the context of nanopore sensing, it is important to either minimize EOF as much as possible or to keep it constant at a well-defined level in order to analyze and interpret translocation time distributions from the motion of particles or macromolecules through the pore.27,107,114
The second phenomenon that originates from charges at the nanopore wall is ICR, which requires either an asymmetry of the pore geometry or of the distribution of surface charges along the pore's long axis.115–117 The resulting asymmetric ion distribution leads to a preferential current flow towards one polarity of the applied electric potential difference compared to the other polarity, leading to non-linear curves of current as a function of applied voltage similar to an electric diode.115,118–123 This phenomenon can be exploited for sensing purposes,121,124–126 however for applications that benefit from a uniform electric field along the nanopore, ICR should typically be eliminated.80 Surface coatings provide a way to increase, reduce or invert surface charges on the walls of nanopores.127–130 Tuning the charge density on the nanopore wall or the ionic strength of the electrolyte solution adjusts the screening length of the EDL and can hence be used to manipulate the velocity of EOF in the nanopore channel.119
To address problems such as limited stability of synthetic nanopores by slow “etching” in electrolyte solutions131,132 or non-specific interaction of analytes with pore walls of synthetic nanopores, various coating methods have been developed (Fig. 2). These methods range from metal oxide deposition to self-assembled monolayers of thiols on gold (Table 1) and have been discussed previously for use in nanopore experiments in several excellent review articles.133–139 This review provides an update as well as a comprehensive exploration of the current status of the use of surface coatings in the nanopore field. With this goal in mind, Table 1 presents an overview of the eight most common coating methods together with their suitability for various applications. Following the organization in this table, this review discusses these coatings as well as their benefits and limitations for the analysis of single macromolecules and particles. While the primary motivation and rationale for nanopore coatings is often to avoid adhesion to the pore wall, once applied, these coatings provide additional advantages, which we will discuss throughout this review.
Table 1 Comparison of the main benefits and characteristics of different methods for coating nanopore walls
Method |
Application |
Characteristics |
Reduce non-specific interactions |
Manipulate surface charges |
Engineer specific interactions |
Change pore diameter |
Reduce noise |
Ease of coating |
Stability of coating |
Specialized equipment |
Coatings which provide a better than average positive outcome are marked with (++), coatings which provide a positive influence on nanopore sensing are marked with (+), those with a neutral influence are marked with (•), and coatings that may incur a negative effect with regard to a certain property are marked with (−). For a self-assembled monolayer of high quality involving a gold surface with the application of thiols, a fresh, unoxidized layer of gold is necessary, typically requiring a set up for sputtering or otherwise depositing thin films of gold. |
Depositions from the vapor phase82,130,140–154 |
• |
+ |
• |
++ |
+ |
++ |
++ |
Yes |
Surfactants128,155,156 |
+ |
+ |
• |
• |
• |
++ |
− |
No |
Other physisorbed surface modification157–160 |
+ |
+ |
+ |
• |
• |
++ |
− |
No |
Layer-by-layer self assembly161–170 |
+ |
++ |
+ |
++ |
• |
• |
+ |
No |
Silanization129,171–191 |
+ |
+ |
++ |
+ |
• |
+ |
+ |
No |
Self-assembled monolayers of thiols on gold188,192–209 |
+ |
+ |
++ |
+ |
• |
+ |
+ |
Yesa |
Other covalent surface modifications123,127,210–254 |
+ |
+ |
++ |
+ |
• |
+ |
+ |
No |
Fluid lipid coatings27,107,114,255–261 |
++ |
++ |
++ |
+ |
• |
− |
• |
No |
Types of surface coatings for synthetic nanopores
Depositions of coatings from the gas phase
Vapor depositions by atomic layer deposition (ALD)262 and chemical vapor deposition (CVD)263 allow for the application of material in a well-controlled manner.142 The precision, in terms of layer thickness, especially of ALD, which cycles through the deposition of individual single-molecule layers, makes gas-phase depositions an attractive technique for coating nanopore walls.264 Alternatively, electron beam-induced deposition (EBID) is a technique for spatially localized deposition that involves physisorption of precursor molecules on the surface followed by deposition mediated by electrons.265,266 Potential benefits of depositions on membranes containing a nanopore, besides the obvious change in pore size and shape,140,142,143,146,147,150,151,154 include reduction of recording noise,142,149 modification of surface properties such as charge or hydrophobicity,130,140,142,147,148 control of current rectification,152 and manipulation of surface interactions with analytes of interest or with other molecules in a sample.141 While depositions of coatings from the gas phase make it possible to reduce the pore diameter, the same process leads to a concomitant, and often undesired increase in nanopore length. Long pores, on the one hand, increase the dwell times of resistive pulses, thereby improving the time resolution; on the other hand, long nanopores have an increased sensing volume and thereby result in a decreased signal to noise ratio compared to shorter pores with the same diameter.
Due to the limited accessibility to recessed nanoscale features, depositions of a continuous film can be difficult to achieve inside nanopores. Elam et al. explored this limitation on pores with high aspect ratios (length/diameter up to 5000) by assessing the uniformity of the coatings at various locations in the pores.144 These authors utilized Monte Carlo simulations to predict the necessary exposure times in a general form that could be applied to any porous substrate.144,145 In order to further increase the quality of depositions in nanopores Fan et al. developed a dual-stage ALD process. This process led to coatings with high levels of homogeneity and conformity within the pores.153
Chen et al. demonstrated that controlled deposition of Al2O3 by ALD is a potential strategy to reduce 1/f noise, control the diameter, and neutralize the surface charge of nanopores prepared by ion beam sculpting in silicon nitride membranes.142 The authors showed that this coating increased the throughput of DNA translocations through the pore compared to a pore in uncoated SiNx membranes (Fig. 3). They attributed low throughput before deposition to a variable surface charge distribution within the nanopore and hypothesized that the uniform surface properties after ALD coating enabled DNA analysis without clogging and long-lived or permanent blockages.142
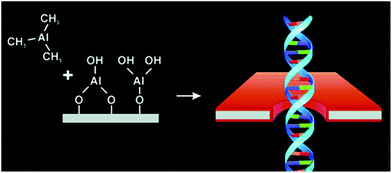 |
| Fig. 3 Representation of the deposition of Al2O3 on a solid-state chip with a nanopore as an example of a coating prepared by ALD. The coating is shown in red. This Al2O3 coating allowed Chen et al. to analyze DNA strands without clogging.142 Reprinted with permission from ref. 142. Copyright 2004 American Chemical Society. | |
In an effort to discriminate between single- and double-stranded DNA, Thangaraj et al. performed ALD of Al2O3 on track-etched nanopores in poly(ethylene terephthalate) (PET) films to reduce the surface charge as well as to control the shape and size of these pores.130 Specifically, the deposition reduced the diameter by ∼25% and reduced current fluctuations resulting from free polymer chains on the surface after the track-etching process. The resulting change in pore diameter and increase in aspect ratio, lowered the strength of the electric field and prolonged dwell-time in the pore, enabling the detection of single-stranded DNA (ssDNA) and double-stranded DNA (dsDNA).130
In order to reduce the diameter of nanopores, Kox et al. used EBID for applying a coating of a hydrocarbon compound.146 The deposition shrunk the nanopore size from around 100 nm to a diameter of 20 nm and the resulting elimination of ICR suggested that the shape of the pore changed from conical to symmetric. In addition, using a SiO2 precursor in EBID led to a chemically stable and constant surface charge, facilitating the detection of biological macromolecules.146,147
One important aspect of deposition techniques on membranes containing nanopores is that they can increase the stability of the membranes, and in particular, of the pore diameter against slow etching in electrolyte solution during recordings. The long-term stability of the nanopore diameter is essential for quantitative and reproducible experiments, since a small change in nanopore diameter can induce a relatively large difference in the sensing volume, the resistance and the thermal noise of nanopores. During nanopore recordings, pores are immersed in an electrolyte solution with an applied potential difference and are often cleaned aggressively by a hot solution of concentrated sulfuric acid with hydrogen peroxide (so called Piranha solution) or by an O2 plasma between experiments. While SiNx and SiO2 are considered chemically and physically robust materials, there is nonetheless a problem with slow etching on the nanometer scale.267 Specifically, SiO2 on the nanopore walls, which originates either from membranes composed entirely of SiO2 or from oxidation of the surface of SiNx membranes, hydrolyzes slowly to silicic acid and dissolves in the electrolyte solution during recordings.131,268
The etch rate of SiNx or SiO2 during nanopore experiments varies as a function of temperature, pH, salt concentration, applied voltage, nanopore shape and nanopore fabrication methods132,267,269,270 and can sometimes be sufficiently fast to lead to a noticeable increase in conductivity through the growing pore during the experiment. The resulting uncertainty in pore diameter, shape, volume, and electric field inside the pore leads to uncertainty in quantitative resistive pulse experiments that aim to characterize translocating particles or molecules.
One promising coating that can be applied by gas phase deposition is hafnium oxide (HfO2). This coating has shown high chemical stability during extended nanopore experiments.71,88 For instance, by depositing a thin layer of HfO2 using ALD on the walls of a nanopore formed in a SiNx membrane, Yamazaki et al. have effectively inhibited SiNx dissolution in a photothermal etching environment (Fig. 4).82 Coating nanopores with a protective self-assembled monolayer (SAM) has also been shown to prevent nanopores from slow etching in aqueous electrolyte solution and enabled measurements for several days.172,271 Nonetheless, slow etching of nanopores leading to increasing pore diameters continues to be one of the major challenges in recordings with solid-state nanopores, especially when very thin insulating membranes (<30 nm) are required.
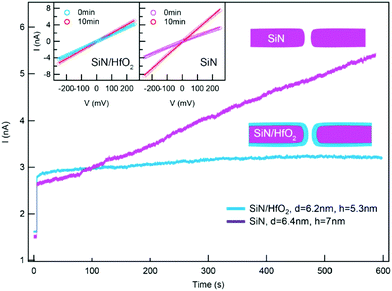 |
| Fig. 4 Coating a nanopore in a SiNx membrane with a layer of HfO2 effectively inhibited the growth of the nanopore diameter.82 Graph showing a time-dependent recording of baseline current through an uncoated nanopore in a SiNx membrane (purple) and through a nanopore coated with HfO2 (blue) while a voltage of 100 mV was applied and while the membrane was simultaneously illuminated by a laser (532 nm). Insets: Current versus voltage curves showing the difference in resistance of two nanopores before and after measurements that lasted for 10 min. Reprinted with permission from ref. 82. Copyright 2018 American Chemical Society. | |
Surfactant-based nanopore coatings
Surfactants (surface-active agents) can adsorb on surfaces and alter the surface chemistry of that surface.128,272–274 These amphiphilic agents consist of both hydrophobic and hydrophilic residues, allowing, in some cases, for their unaided adhesion to surfaces.274 Surfactants typically lower surface tension and may perform additional functions such as foaming, inhibiting corrosion, and killing bacteria.272,273 Nanopore coatings with surfactants such as Tween 20 and cetyl trimethyl ammonium bromide (CTAB) are primarily employed to reduce interactions of biomolecules with the pore wall;155,156 they can, however, also provide the ability to alter surface charge density and therefore current rectification.128
Hu et al. showed that the commercially-available surfactant Tween 20 prevented irreversible clogging of nanopores as a result of minimized protein adsorption to the pore wall;155Fig. 5 illustrates the proposed mode of action.155 Specifically, the authors suggested that Tween 20 application to silicon nitride nanopores rendered the surface more hydrophilic (as confirmed by contact angle experiments), minimizing adhesion of the protein alpha-synuclein that is implicated in Parkinson's disease.275 This modification allowed for the identification of four types of alpha-synuclein oligomers155 as well as the differentiation between ssDNA and dsDNA.156
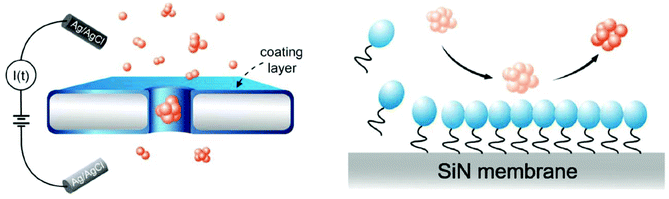 |
| Fig. 5 Cartoon representation of the putative effect of Tween 20 (shown in blue) on non-specific adsorption of proteins to the pore wall.155 The circles represent the hydrophilic moiety while the tails represent the hydrophobic moiety of the surfactant molecules. Figure from ref. 155. | |
Xie et al. tested CTAB for generating a coating that inverted the negative surface charge of track-etched nanopores in PET foils to a surface with a positive charge.128 Through the adjustment of the CTAB concentration in the solution used for recording, the authors changed the surface charge from −9 mC m−2 to +8 mC m−2, and thereby tuned the properties of current rectification.128
Other coating methods by physisorption
The physisorption of molecules to generate coatings on a membrane with a nanopore constitutes one of the most straightforward to use surface modifications. To this end, one of the most commonly employed approaches is adsorption of positively-charged poly-L-lysine (PLL) onto negatively charged surfaces. These PLL coatings were reported to block the adhesion of molecules on the pore wall,157 to allow for the manipulation of surface charges,157,159 as well as to engineer specific interactions. One example of a specific interaction was that of mycotoxins160 and the protease thrombin158 using a cross-linker that attached to the amino groups in PLL and to cysteine residues on antibodies specific to the molecule of interest.
In the case of nanopores in graphene, Schneider et al. showed that the non-covalent self-assembly of a monolayer of amphiphilic molecules, which exposed hydrophilic end groups blocked hydrophobic interactions between DNA and the graphene walls of the pore.157 This coating was composed of a molecule that combined a hydrophobic aminopyrene residue with a hydrophilic tetrameric ethylene glycol moiety. The pyrene moiety putatively interacted with the graphene and the ethylene glycol protruded out from the pore wall, rendering the surface hydrophilic. This modification enabled the detection of dsDNA and ssDNA with improved reproducibility,157 illustrating the potential of coated nanopores in graphene sheets.
Umehara et al. examined the effect of PLL coatings on the mobility of ions within nanopipette electrodes.159 Uncoated pipettes exhibited ICR as expected from their conical shape, while PLL-coated pipettes displayed increased rectification at the opposite polarity compared to the uncoated pipettes.119 This change occurred as the positively charged PLL coating inverted the polarity of the negative surface charge of the bare glass wall of the nanopipette.
Coatings made from PLL were also used in a so-called signal transduction by ion “nanogating” (STING) sensor using a quartz nanopipette.158,160 Actis et al. introduced this concept for the detection of the mycotoxin HT-2 by taking advantage of immunoglobulin (IgG) molecules crosslinked to the PLL coating (Fig. 6).160 Immobilization of thrombin aptamers to a layer of PLL and polyacrylic acid (PAA) allowed for the detection of thrombin using the same sensing platform.158
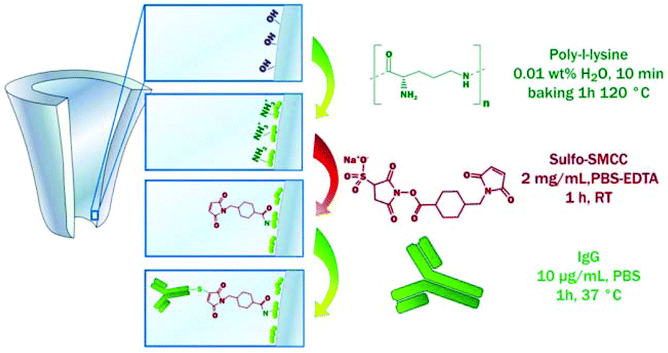 |
| Fig. 6 The functionalization of a quartz nanopipette by the physisorption of PLL and subsequent immobilization of IgG molecules. This coating allowed for the detection of the mycotoxin HT-2, a small toxin that is difficult to detect.160 Reprinted from ref. 160 with permission from Elsevier. | |
Coatings formed using layer-by-layer self assembly
The coating technique layer-by-layer self-assembly (LBL) employs a cycle of alternating deposition of oppositely charged polyions to create thin films.276–279 These depositions typically begin with a positively charged layer to capitalize on the negative charges present on most surfaces, including glass, silicon, and metals.280,281 Layer-by-layer self-assembly allows for nano-scale precision when adjusting the diameter of a nanopore since each bilayer usually contributes an increase in thickness of less than 1 nm. While the compositions of the layers and the deposition techniques vary, LBL coatings are most commonly used to manipulate nanopore size,164 tailor surface chemistry,165–169 or allow for the incorporation of other molecules for specific detection of certain analytes.161–163,166,167,170
In order to adjust the diameter and surface charge density of a nanopore, Lepoitevin et al. deposited alternating layers of PLL and poly(styrene sulfonate) onto track-etched, conically-shaped nanopores in PET.167 This approach modified the pore's ICR characteristics, while the addition of PLL grafted with poly(ethyleneglycol) (N-hydroxysuccinimide 5-pentanoate) ether 2-(biotinylamino) ethane (NHS-mPEG-biotin) made it possible to attach or recognize biotin-binding proteins.167 To design a nanopore that could be gated by the variation of pH and that responded to differences in ion concentration, Zhao et al. performed LBL with polyethylenimine (PEI) and chondroitin-4-sulfate (ChS) on track-etched pores (Fig. 7).168
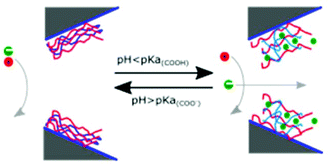 |
| Fig. 7 The mechanism of a nanopore gate formed by layer-by-layer self-assembly of PEI and ChS and its response to changes in pH value of the aqueous recording electrolyte as proposed by Zhao et al.168 At low ionic strength, the ionic current through the pore responded to changes in pH value: at pH values smaller than 4, the gate opened for flux of anions, while at pH values above 4, anion flux was significantly reduced.168 Reprinted with permission from ref. 168. Copyright 2017 American Chemical Society. | |
Blundell et al. used layer-by-layer assembly to functionalize conical nanopores prepared in thin polyurethane membranes.166 The coating made it possible to control the ionic conductance through the nanopore by changing the pH value and ionic strength of the recording electrolyte.166 The authors demonstrated that layers composed of PEI and polyacrylic acid-maleic acid (PAAMA) with the incorporation of an aptamer enabled the detection 5 pM concentrations of the cancer biomarker vascular endothelial growth factor (VEGF).166
Nanopore coatings by silanization
Silanization involves the reaction of organosilanes with surface hydroxyl groups, in a process that can be associated with molecular self-assembly.282,283 Silanes comprise both organic and inorganic moieties and can form covalent bonds with varying levels of stability282 on surfaces of a variety of substrates including quartz,284 aluminum oxide,285 and iron oxide.286 In the absence of polymerization, silanization forms thin coatings with low surface density, which may be used to increase hydrophobicity185,190 or to reduce non-specific surface adhesion.283 In the context of nanopores, silanization allows for the functionalization of pore walls by enabling the attachment of DNA,173,175,178,183 dendrimers,174 nucleoporins,176 aldehydes,172,177 spiropyran moieties,181 cysteines,187 carboxylic acid,172 EDTA,188 peptides,189,191 and polymer brushes182 to chemical groups that are attached to the silane molecule. Apart from the possibility of such attachments, silanization can generate a coating with antifouling properties184 and can be used to manipulate ICR185 and other charge-based properties,179 including the modulation of surface charge by changing the pH value of the recording electrolyte129,180,186 and the regulation of transport through the conformational change of ligands in response to light or heat.171
Tan et al. performed silanization with 3-aminopropyltriethoxysilane (APTES) on silicon nitride nanopores (which typically have a thin layer of SiO2 on their surface287) to render the net charge of the pore surface positive due to protonated terminal amine groups.179 The authors chose a functionalization with amine groups to reduce EOF-related drag as well as to attract negatively charged nanoparticles to the pore entrance for detection of translocation events at increased frequency.179
Wanunu and Meller created nanopores that responded to changes in pH or to the presence of certain proteins by attaching carboxylic acid or aldehyde groups to silane coatings on the pore. To this end, they coated the pores with a variety of organosilane reagents containing epoxy, methoxyethylene glycol and amine moieties before attaching molecules that either displayed carboxylic acid groups or displayed aldehyde groups upon conjugation.172Fig. 8 shows the pH sensitivity exhibited by such a system. The coatings were formed in two ways: through (i) immersion of nanopore chips in the silane solution and (ii) voltage-driven mass transport to promote uniform coating of small pores (5 nm in diameter) without clogging.172 To understand the pH dependence of selective transport of certain ions through nanopores, Wang et al. applied two different alkylsilanes to conical glass nanopores with a platinum disk electrode embedded at the bottom of the pore.180 Specifically, a monolayer terminated in –CN groups modified the exterior surface while an amine-terminated monolayer modified the interior surface of the pore. Protonation and deprotonation of the –NH2 groups affected the flux of charged species.180
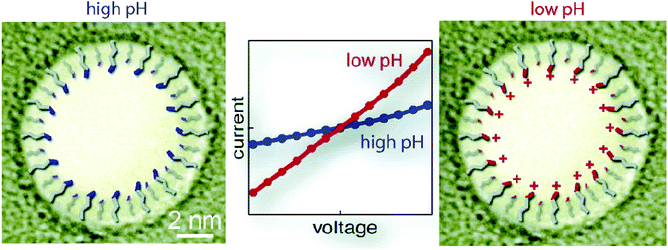 |
| Fig. 8 Effect of pH-dependent surface charge of nanopores coated with a silane with protonatable end groups proposed by Wanunu and Meller.172 Uncoated pores did not show the same pH-dependence as those coated with 3-(aminopropyl)trimethoxysilane (APTMS). At relatively low ionic strength (0.14 M KCl), the conductance through coated pores varied with the pH value in the recording electrolyte, while uncoated pores displayed no sensitivity to pH at either low ionic strength or at 1.0 M KCl.172 Reprinted with permission from ref. 172. Copyright 2007 American Chemical Society. | |
To allow selective detection and sequencing of short strands of DNA through specific interactions with a binding partner in a nanopore, Iqbal et al. attached a hairpin loop of DNA via a silane layer (APTES) and a homo-bifunctional crosslinker (1,4-phenylene diisothiocyanate). The silanization of these pores also decreased their effective diameter to increase the amplitude of resistive pulses generated by DNA translocation.175 Also in the pursuit of DNA sequencing, Anderson et al. silanized solid-state nanopores to form a ‘polymeric cushion’ between the DNA and the pore walls.129 This cushion, composed of APTMS, prevented DNA from sticking to the nanopore walls and slowed its translocation time through the modification of the surface charge. By varying the solution's pH, the authors were able to vary the translocation times of unfolded DNA.129
Nilsson et al. functionalized nanopores in a silicon nitride membrane that had been prepared by focused-ion-beam drilling through a three step process.178 They first grew a silicon oxide ring locally through ion-beam-assisted deposition. The oxide surface then reacted with mercaptopropyltrimethoxysilane to anchor thiol-terminated linkers. Finally, acrylamide-terminated ssDNA strands reacted with the thiol groups on the linkers, enabling detection of specific biological materials (anything from viruses to cells) through reactions with these DNA probes.178 In another example, Ding et al. immobilized aptamers on the silanized wall of nanopores in order to render glass nanopores specific for detection of proteins.173 Interaction of immunoglobulin E (IgE) and ricin molecules with aptamers in the narrow sensing zone of the pore enabled their detection.173
Tang et al. coated solid-state nanopores in silicon nitride membranes with polyethylene glycol (PEG200) to improve the detection of ssDNA and dsDNA.190 This PEG layer lowered hydrophilicity,190 1/f noise, and the pH-dependent surface charge.
Coatings from self-assembled monolayers of thiols on gold
Self-assembled monolayers288 are a well-studied and commonly employed approach to modify or functionalize surfaces for a variety of applications ranging from prevention of corrosion, formation of protein-repellant surfaces,289 to employment as active or passive elements in transistors or switches.290,291 The SAMs discussed here are composed of molecules with terminal thiol groups that allow for covalent conjugation to a freshly prepared gold layer on the nanopore surface. Apart from gold deposition, SAM preparation does not require specialized equipment, the monolayers can form over large surfaces and they can provide surface groups that repel molecules, interact with, or covalently link to molecules of interest.288,292 In the context of nanopore sensing, SAMs are predominantly used for sensing specific analytes,193,201,203,204 minimizing non-specific interactions,188,197,200,202,208 manipulating surface charge,192,194,196–198 and adding functionality such as gating of the pore,192,205,206 preferential transport,194,196–199 or enhancing the signal of plasmonic nanopores.207,209
Charles R. Martin's group was among the first to take advantage of SAMs in nanopores by chemisorbing thiols to gold surfaces deposited onto track etched nanotubes.195 The same group has since explored other modifications involving SAMs.198 For example, they varied the hydrophobicity of gold nanotubules by choice of the R group in the alkane thiol molecules that they chemisorbed to the tubule walls in order to explore its influence on transport of molecules with varying hydrophobicity.196 This research showed that membranes made from functionalized gold nanotubules separated hydrophobic molecules from hydrophilic species. In another study, Lee and Martin chemisorbed cysteine to gold nanotubule membranes to introduce pH-switchable selectivity for ion-transport.197 At a low pH (when the cysteine's carboxyl and amino groups were protonated), the membranes allowed the passage of anions and rejected cations (Fig. 9). The opposite was true at a high pH. At the isoelectric point of cysteine (pH = 6.0), no transport selectivity was observed.
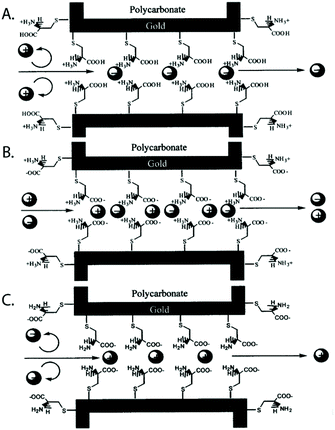 |
| Fig. 9 A representation of the three protonation states of cysteine chemisorbed to a gold-coated nanopore wall as proposed by Lee and Martin.197 A. At a low pH, the cysteines were protonated resulting in a state that permitted transport of anions and rejected cations. B. Close to the isoelectric point of cysteine, a pH of 6, no significant selectivity for cations or anions was observed. C. At a high pH, the cysteines were deprotonated resulting in a state that permitted transport of cations and rejected anions.197 Reprinted with permission from ref. 197. Copyright 2001 American Chemical Society. | |
In another example of engineering specific interactions on the pore surface, He et al. formed a gold film on glass nanopores with ∼30 nm diameters and decorated the film by self-assembly of 2-thiouracil (2-TU).193 Hydrogen bonding between the amide moieties of uric acid and the 2-TU surface molecules allowed for the specific detection of uric acid, a biomarker used in the diagnosis of diseases like gout, arthritis and renal disease.293 As the authors increased the concentration of uric acid in the recording buffer, the ionic current increased to a stable value, indicating the binding of uric acid to the SAM coating.193
A SAM of nitrilotriacetic acid (NTA) groups on the gold-coated walls of a nanopore enabled specific detection of His-tagged proteins.204 This SAM also shrunk the diameter by ∼6 nm and prevented nonspecific interactions between proteins and pore walls. Wei et al. demonstrated the specificity for binding of His-tagged proteins with control experiments using imidazole as a competitive binder (Fig. 10).204 In another example of using nanopores in the context of protein biophysics, Jovanovic-Talisman et al. sought inspiration from the nuclear pore complex (NPC) and rendered the walls of nanopores in a polycarbonate film specific for transport of proteins of interest.203 To recreate the NPC, the authors applied a gold layer on synthetic nanopores, attached FG-nucleoporins through a C-terminal cysteine, and attached small PEG-thiol molecules to passivate remaining areas of exposed gold. This artificial NPC effectively behaved as a filter, allowing the preferred passage of cargo in complex with transport factors specified to bind to multiple repeats of Phy-Gly (FG) motifs in the FG-nucleoporins.203
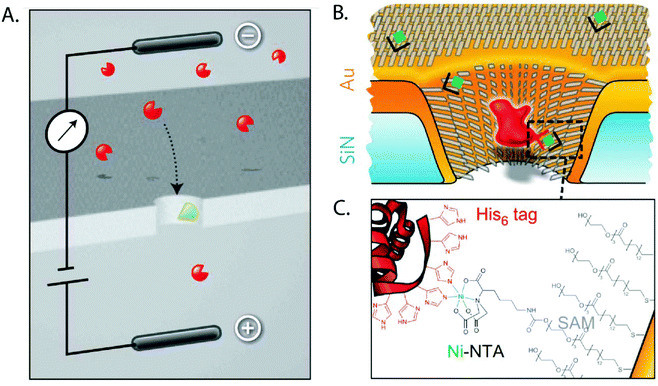 |
| Fig. 10 Cartoon depictions of the SAM-coated nanopore used for sensing specific proteins as proposed by Wei et al.204 A. Schematic of the principle of specific analyte sensing. The proteins of interest are shown in red while the receptor that is specific to the molecule of interest is shown in green. B. The same concept shown in detail on a gold-coated nanopore with the His6-tagged proteins shown in red. C. The binding between the His-tagged protein and the SAM coating the nanopore.204 Reprinted with permission from ref. 204. Copyright 2012 Springer Nature. | |
Sexton et al. attached PEG-thiol molecules to a gold layer prepared on the surface of track etched conical nanopores in PET membranes for the prevention of protein adsorption.202 With these PEG-functionalized nanopores, the authors distinguished translocation of bovine serum albumin (BSA) in complex with anti-BSA Fab fragments from translocations of BSA alone.202 This work followed a study from the same group, which exploited the advantages provided by a PEG-thiol coating to separate proteins as a function of their size by adjusting the size of the nanotubules.199
Siwy et al. deposited gold on conical nanopores in PET membranes to form gold nanotubes and functionalized the tubes with three different molecules for molecular-recognition.201 Specifically, the authors exploited the strong interaction between biotin and streptavidin, protein G and IgG, and ricin and its antibody to increase the selectivity and sensitivity of the pores for these three analytes. The result was a simple Boolean sensor: binding of the molecule of interest near the nanotube orifice led to a current blockage indicating the presence of the molecule.200,201
Other covalent surface modifications
Nanopore surfaces that expose carboxyl groups are often coated by reaction with carbodiimide moieties for coupling molecules of interest.123,127,212–234,237,238,240–247,254 This technique works well on the walls of nanopores in polymer films and is robust and versatile, particularly for imparting specificity for detection of specific DNA strands or other biomolecules.217,218,223,224,227,229,232,244,254 The same coupling chemistry has been employed to engineer nanopore systems that can be gated210,212,214–216,219,221,235,238,241,246 or to generate nanopore diodes.123,219,232,233,239,242 Other covalent modification techniques include spin-coating,252 hydrosilylation,253 plasma-induced graft polymerization210,211 and crosslinking other functional groups such as DNA strands,235,236 spiropyrans,239 or 4-carboxyl benzyl phosphonic acid248 directly to the surface of the pore wall.
To create a pH- and voltage-sensitive mesh within a nanopore, Buchsbaum et al. attached ssDNA probes to the walls of conical pores in a PET film by reacting the amino groups at the 5′ end of DNA oligomers with the carboxyl groups on the pore wall.212 At a low pH, the DNA strands bound to each other through electrostatic interactions (AC-rich strands became protonated and GT-rich strands did not) and increased the resistance through the pore by approximately 60-fold to several tens of gigaohms. At a neutral pH, switching the polarity of the applied voltage controlled the ‘gating’ mechanism: with the application of a negative potential, the authors proposed that the DNA strands preferentially deflected towards the smaller pore opening, causing a partial blockade, while the opposite polarity presumably caused the end of the DNA strands to move preferentially towards the larger opening, “opening” the pore.212 This research group also created diodes and transistors from a nanopore in a polymer film.123,241,242 To realize a similar strategy for gating the ion flux through a nanopore, Lepoitevin et al. performed ALD of thin Al2O3/ZnO films on track-etched nanopores in a PET film followed by exposure of the nanopore chip to N-[3-(trimethoxysilyl)propyl] ethylenediamine (AEAPTMS) vapor. This treatment generated –NH2 groups on the surface. Finally, they linked biotin-PEG molecules to the pore walls through AEAPTMS grafting to the surface –NH2 groups.213,214 Changes in pH resulted in changes in the resistance of the nanopore or, after functionalization of the biotin-PEG layer with the proteins avidin or streptavidin, this system detected biotinylated IgG, and biotinylated BSA.213 Finally, the same group applied a PEG layer on the walls of nanopores in a PET film through linking to carboxylate groups on the pore surface to enable the detection of amyloids without clogging247 or they attached PEG-spiropyrans to the same pores to generate a light- and pH-responsive nanopore.246
Inspired by biological ion channels, Brunsen et al. functionalized a mesoporous thin film of silica with polymer brushes composed of poly(methacryloyl ethylene phosphate) (PMEP) to modulate ion transport by changing pH. The polymer brushes either interacted with or repelled each other depending on the pH.251 Yameen et al. explored this concept on conical nanopores by influencing ion flow based on thermally-controlled gating (Fig. 11).215,216,249,250 Briefly, at room temperature the polymer brushes were in a swollen state while an increase in temperature past the critical solubility level caused the brushes to switch to the collapsed state, increasing the nanopore's effective diameter.
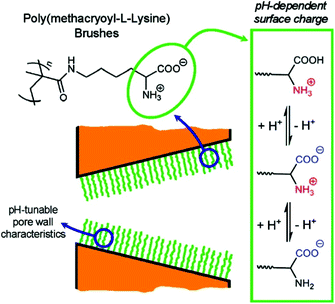 |
| Fig. 11 Cartoon depiction of nanopores with a coating of polymer brushes whose ion transport properties could be tuned by changes in pH.249 Reprinted with permission from ref. 249. Copyright 2009 American Chemical Society. | |
Ali et al. constructed a device for the detection of ssDNA oligonucleotides through carbodiimide-mediated coupling of specific peptide nucleic acid (PNA) probes to the surface of track-etched nanochannels in polyimide membranes.217 These uncharged PNA probes also decreased the pore's ICR by about 70% compared to the ICR before modification.217 Using the same technique to immobilize aptamers designed to selectively bind the enzyme lysozyme, this group locally anchored lysozyme onto the pore surface to accumulate charge and increase ICR. Lysozyme has a high isoelectric point of 11.4,294 therefore the molecules were positively charged under the experimental conditions.218 Kececi et al. modified PET membranes by reacting surface-exposed –COO− groups with ethanolamine through (1-ethyl-3-[3-dimethylaminopropyl] carbodiimide hydrochloride, EDC) coupling chemistry to reduce surface charge.127 The authors used these nanopores to detect short DNA strands and to distinguish between strands of different lengths.
Fluid lipid coatings
Coatings from fluid layers of lipids are attractive because they solve several of the most common problems in the context of nanopore recordings of proteins and other macromolecules.107,114,295 Inspired by the lipid-coated nanopores present in the antennae of silk moths296,297 (Fig. 12A), our group demonstrated, for instance, that lipid coatings efficiently prevent or minimize non-specific adsorption of proteins to the pore wall, eliminating clogging.27,80,107,114,258,259 In addition, lipid coatings make it possible to imbue the coating with the capability to engage in specific interactions with target analytes by the incorporation of lipid-anchored ligand or receptor molecules. Binding of proteins of interest to these ligands or receptors tethers them to the bilayer (Fig. 12B) but due to the fluid nature of this lipid coating, lipid anchored target proteins can still move and translocate through lipid-coated nanopores. Depending on the strength of the interaction, these lipid anchors can concentrate molecules of interest onto the fluid coating, increasing the sensitivity of detection.107 Alternatively, proteins can be cross-linked covalently to lipid anchors27 or proteins such as GPI-anchored proteins, which are intrinsically lipidated, can be examined.107 Lipid anchors provide the advantage to slow down the speed of translocation of the anchored target molecules by two orders of magnitude as a result of the drag of the anchor in the viscous fluid lipid coating. Finally the zwitterionic nature of lipids with phosphatidylcholine head groups in the coating almost completely eliminates electroosmotic flow107,114,255,256 and eliminates or minimizes non-specific interactions with many proteins.27
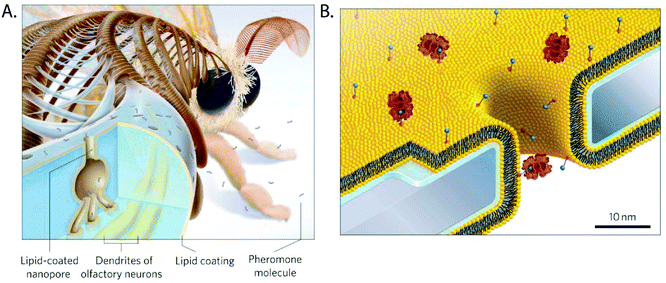 |
| Fig. 12 Lipid coated nanopores and their inspiration.107 A. The nanopores through the exoskeleton of the sensilla in the antennae of the silk moth are lipid-coated to facilitate diffusion of pheromone molecules towards their receptors on the dendrites of olfactory neurons.299 B. Cartoon of a lipid coated (yellow) nanopore with proteins (red) anchored to lipids presenting a ligand (blue) on their headgroup. The water layer with a thickness of ∼1 nm between the supported lipid bilayer and the chip surface is indicated in blue.107 Figure from ref. 107. | |
Fig. 12 shows a schematic of a nanopore coated with a phospholipid bilayer. In one example, our group took advantage of lipid coatings to investigate the aggregation of amyloid-β oligomers, which are associated with neurodegenerative diseases such as Alzheimer's disease.259,275 Without the fluid lipid coating these experiments typically ended within seconds because amyloid-β samples clogged uncoated nanopores in silicon nitride membranes298 while lipid coatings enabled recordings for more than 40 min. More recently, we took advantage of the reduced translocation speed of lipid-anchored proteins to characterize them on the single molecule level by determining their shape, rotational diffusion coefficient, dipole moment, and charge.27 For these applications, the lipid coating is essential because, on the one hand, it slows down the rotation and translocation of lipid-anchored proteins sufficiently to time-resolve their rotational motion in the pore27,295 and, on the other hand, the coating provides a non-stick surface that enables translational and rotational motion of the protein without artifacts from non-specific adsorption.295 Artifact-free rotation is required to quantify a protein's rotational diffusion coefficient as well as its bias towards certain orientations in the electric field inside the nanopore; it is this bias that we used to estimate the dipole moment of individual proteins.27,295 We compared other nanopore coatings such as silanization and surfactant coatings with Tween 20
295 to lipid coatings but in our hands these alternative coatings did not eliminate wall interactions and led to artifacts.
Our group explored a variety of lipid compositions with regard to their benefits in fluid lipid coatings.114 To this end, we evaluated the lipid coatings based on four major characteristics: stability of the recorded current baseline, current noise, ability of the coating to slow down the speed of translocation, and ease of preparing a stable nanopore coating. We concluded (Table 2) that the best coatings were either composed of 1-palmitoyl-2-oleoyl-sn-glycero-3-phosphocholine (POPC) with 0 to 20 mol% cholesterol or of monolayer-forming lipids inspired from Archaea with 0 to 40 mol% POPC. These tethered lipids provide the advantage that they result in fluid lipid coatings with approximately 10-fold increased viscosity compared to POPC coatings; these viscous coatings resulted in the longest translocation times of lipid-anchored proteins that we could achieve to date. One limitation is that Archaea-inspired, monolayer-forming lipids are not readily available.
Table 2 Comparison of different lipid coatings with regard to their ability to form stable coatings with low current noise during nanopore recordings as well as their ability to slow down the speed of translocation of lipid-anchored analytes. Table from Eggenberger et al.114
Lipid composition of coating |
Stable baseline |
Low noise |
Slow translocation |
Straightforward to coat |
100% POPC |
+ |
+ |
+ |
+ |
25, 50, 80, 90, 100% Archaea lipids + 75, 50, 20, 10, 0% POPC |
+ |
+ |
++ |
+ |
100% DiPhyPC |
− − |
− − |
+ |
+ |
100% Di-O-PhyPC |
− − |
− − |
+ |
+ |
60% DOPC + 20% DOPE + 20% LysoPC |
+ |
− |
+ |
+ |
10, 20, 30, 40% cholesterol + 90, 80, 70, 60% POPC |
+ |
+ |
+ |
+ |
50% cholesterol + 50% POPC |
+ |
+ |
+ |
− |
Venkatesen et al. applied the concept of coating nanopores in SiNx membranes with lipid bilayers to coating nanopores in free-standing membranes of aluminum oxide (Al2O3).257 Specifically, the authors used the liposome rupture technique with high osmotic pressure in the presence of Ca2+ ions to coat Al2O3 that had been deposited by ALD. The deposition of lipid bilayers composed of 1,2-di-(9Z-octadecenoyl)-sn-glycero-3-phosphocholine onto membranes with single nanopores increased the impedance from less or equal to 1 MΩ to more than 1 GΩ and allowed for the integration of a biological nanopore into the lipid membrane for the formation of a hybrid nanopore.257
Hernández-Ainsa et al. showed that lipid coatings can also be applied onto the walls of nanopores in quartz nanocapillaries.258 In this case, the lipid bilayers increased the ratio of λ-DNA detection from 13% to 40% presumably due to the reduction of surface charges and minimization of non-specific adsorption of DNA to the coated capillary walls.258
Galla et al. demonstrated that applying a zwitterionic supported lipid bilayer to nanopores prepared with a helium-ion beam in silicon nitride membranes almost completely eliminated EOF.255 The authors threaded a single molecule of dsDNA through the pore with the help of optical tweezers and measured the effect of the lipid coating on the threading force. They found that the lipid coating almost completely eliminated EOF leading to an increase in threading force by 85%.255 Sischka et al. compared lipid-coated nanopores to carbon nano membranes (CNMs) and to uncoated silicon nitride membranes.256 These CNMs increased threading forces of DNA by 15% compared to uncoated membranes, showing a slight reduction in EOF, but this reduction was significantly smaller compared to the one enabled by lipid coatings.
When using nanopores in graphene for protein and nanoparticle translocation, Shan et al. detected gold nanoparticles after oxygen plasma treatment but they were not able to detect ferritin proteins after treatment of their graphene membranes with either oxygen plasma or mercaptohexadecanoic acid.260 In order to prevent ferritin adhesion to the pore walls, they modified their graphene membranes with the nanopore by immersion in an aqueous solution of the phospholipid-PEG amphiphile DPPE-PEG750; this treatment facilitated translocation and detection of ferritin but not BSA.260
To facilitate the free movement of proteins held within a nanopore by attaching them to a DNA-origami scaffold,261 Schmid et al. coated solid-state nanopores in SiNx membranes with a lipid bilayer. In this set up the fluid lipid bilayer made it possible to observe a single molecule over extended times inside a nanopore while minimizing non-specific interactions with the pore wall.261
Outlook
The application of a coating to the walls of nanopores makes it possible to address many of limitations that come along with approaches for the detection and characterization of single molecules in synthetic nanopores. For instance, artifacts as a result of adhesion to the pore wall, ICR and EOF can be minimized or enhanced by choice of the appropriate coating. This review outlined the spectrum of approaches to nanopore coatings as well as the resulting benefits and opportunities. While no single coating technique solves all of the problems associated with solid-state nanopores – clogging, instability, unresolved translocation events, or success rate of preparing stable coatings of high quality still present challenges for many nanopore experiments – the coatings reviewed here increased the specificity, sensitivity, versatility, and information content from nanopore-based single molecule experiments. We hope that this overview will be helpful for solving or minimizing some of the problems that hamper the usefulness of nanopore-based analytics of complex, real world samples.275 We predict that the nanopore field will continue to expand the strategies for increasing the functionality of nanopores300 and nanocapillaries301–303 and that coatings will play an essential role in this development. We expect to witness an increase, both in the number of ways how coatings will be applied, and in the fine-tuning of their molecular composition. Another development of interest may be coatings that enable and stabilize, hybrid biological-synthetic nanopores in which at least a selection of protein pores may be tightly embedded into a coated solid-state nanopore while maintaining their full functionality.304–311 Nanopores with coated walls will likely be useful for studies that manipulate or measure the forces acting on molecules during their translocating through pores, including studies that employ a combination of pressure and voltage312 or laser-based trapping.313 Coatings may also become increasingly important for experiments that explore nanopores in membranes made from novel materials or for nanopore studies with unconventional or non-aqueous recording electrolytes or solutions.23,314–316 We are convinced that nanopore coatings will not only continue to improve the functionality of pores but will also provide a means to characterize the pores themselves, for instance with regard to their size and geometry.80,317 With improved coatings, we hope that nanopore-based biophysics and analytics will continue to make a growing contribution to our understanding of biological macromolecules and their interactions as well as to the detection of clinically-relevant biomarkers.318,319
Conflicts of interest
Michael Mayer is an inventor on a patent application about fluid lipid coatings for nanopore experiments.
Acknowledgements
This work was supported by Oxford Nanopore Technologies (Grant No. 350509-N016133) and the Swiss National Science Foundation (SNSF Grant No. 200021_169304).
References
- S. Howorka and Z. Siwy, Nanopore analytics: sensing of single molecules, Chem. Soc. Rev., 2009, 38, 2360–2384 RSC
.
- T. Ito, L. Sun, R. R. Henriquez and R. M. Crooks, A Carbon Nanotube-Based Coulter Nanoparticle Counter, Acc. Chem. Res., 2004, 37, 937–945 CrossRef CAS PubMed
.
- C. Dekker, Solid-state nanopores, Nat. Nanotechnol., 2007, 2, 209–215 CrossRef CAS PubMed
.
- U. F. Keyser, Controlling molecular transport through nanopores, J. R. Soc., Interface, 2011, 8, 1369–1378 CrossRef CAS PubMed
.
- W. Shi, A. K. Friedman and L. A. Baker, Nanopore Sensing, Anal. Chem., 2016, 89, 157–188 CrossRef PubMed
.
- D. Branton,
et al., The potential and challenges of nanopore sequencing, Nat. Biotechnol., 2008, 26, 1146–1153 CrossRef CAS PubMed
.
- D. Anselmetti, Nanopores: Tiny holes with great promise, Nat. Nanotechnol., 2012, 7, 81–82 CrossRef CAS PubMed
.
- B. N. Miles,
et al., Single molecule sensing with solid-state nanopores: novel materials, methods, and applications, Chem. Soc. Rev., 2012, 42, 15–28 RSC
.
- L. Movileanu, Interrogating single proteins through nanopores: challenges and opportunities, Trends Biotechnol., 2009, 27, 333–341 CrossRef CAS PubMed
.
- L.-Q. Gu and J. W. Shim, Single molecule sensing by nanopores and nanopore devices, Analyst, 2010, 135, 441–451 RSC
.
- B. M. Venkatesan and R. Bashir, Nanopore sensors for nucleic acid analysis, Nat. Nanotechnol., 2011, 6, 615–624 CrossRef CAS PubMed
.
- M. Davenport,
et al., The Role of Pore Geometry in Single Nanoparticle Detection, ACS Nano, 2012, 6, 8366–8380 CrossRef CAS PubMed
.
- W.-J. Lan, D. A. Holden, B. Zhang and H. S. White, Nanoparticle Transport in Conical-Shaped Nanopores, Anal. Chem., 2011, 83, 3840–3847 CrossRef CAS PubMed
.
- M. Jain, H. E. Olsen, B. Paten and M. Akeson, The Oxford Nanopore MinION: delivery of nanopore sequencing to the genomics community, Genome Biol., 2016, 17, 239 CrossRef PubMed
.
- M. Jain,
et al., Nanopore sequencing and assembly of a human genome with ultra-long reads, Nat. Biotechnol., 2018, 36, 338–345 CrossRef CAS PubMed
.
- F. Nicoli, D. Verschueren, M. Klein, C. Dekker and M. P. Jonsson, DNA Translocations through Solid-State Plasmonic Nanopores, Nano Lett., 2014, 14, 6917–6925 CrossRef CAS PubMed
.
- J. D. Spitzberg, A. Zrehen, X. F. van Kooten and A. Meller, Plasmonic-Nanopore Biosensors for Superior Single-Molecule Detection, Adv. Mater., 2019, 1900422 CrossRef PubMed
.
- H. Im, J. Wittenberg, N. Lesuffleur, A. C, N. Lindquist and S.-H. Oh, Membrane protein biosensing with plasmonic nanopore arrays and pore -spanning lipid membranes, Chem. Sci., 2010, 1, 688–696 RSC
.
- M. P. Jonsson and C. Dekker, Plasmonic Nanopore for Electrical Profiling of Optical Intensity Landscapes, Nano Lett., 2013, 13, 1029–1033 CrossRef CAS PubMed
.
- M. Belkin, S.-H. Chao, M. P. Jonsson, C. Dekker and A. Aksimentiev, Plasmonic Nanopores for Trapping, Controlling Displacement, and Sequencing of DNA, ACS Nano, 2015, 9, 10598–10611 CrossRef CAS PubMed
.
- O. N. Assad,
et al., Light-Enhancing Plasmonic-Nanopore Biosensor for Superior Single-Molecule Detection, Adv. Mater., 2017, 29, 1605442 CrossRef PubMed
.
- C. R. Crick,
et al., Low-Noise Plasmonic Nanopore Biosensors for Single Molecule Detection at Elevated Temperatures, ACS Photonics, 2017, 4, 2835–2842 CrossRef CAS
.
- F. Traversi,
et al., Detecting the translocation of DNA through a nanopore using graphene nanoribbons, Nat. Nanotechnol., 2013, 8, 939–945 CrossRef CAS PubMed
.
- U. F. Keyser,
et al., Direct force measurements on DNA in a solid-state nanopore, Nat. Phys., 2006, 2, 473–477 Search PubMed
.
- N. Hacohen, C. J. X. Ip and R. Gordon, Analysis of Egg White Protein
Composition with Double Nanohole Optical Tweezers, ACS Omega, 2018, 3, 5266–5272 Search PubMed
.
- J. S. Daniels and N. Pourmand, Label-Free Impedance Biosensors: Opportunities and Challenges, Electroanalysis, 2007, 19, 1239–1257 CrossRef CAS PubMed
.
- E. C. Yusko,
et al., Real-time shape approximation and fingerprinting of single proteins using a nanopore, Nat. Nanotechnol., 2017, 12, 360–367 CrossRef CAS PubMed
.
- L. Ma and S. L. Cockroft, Biological Nanopores for Single-Molecule Biophysics, ChemBioChem, 2010, 11, 25–34 CrossRef CAS PubMed
.
- S. Majd,
et al., Applications of biological pores in nanomedicine, sensing, and nanoelectronics, Curr. Opin. Biotechnol., 2010, 21, 439–476 CrossRef CAS PubMed
.
- Y.-L. Ying, C. Cao and Y.-T. Long, Single molecule analysis by biological nanopore sensors, Analyst, 2014, 139, 3826–3835 RSC
.
- S. Majd, E. C. Yusko, A. D. MacBriar, J. Yang and M. Mayer, Gramicidin Pores Report the Activity of Membrane-Active Enzymes, J. Am. Chem. Soc., 2009, 131, 16119–16126 CrossRef CAS PubMed
.
- S. Blake, T. Mayer, M. Mayer and J. Yang, Monitoring Chemical Reactions by Using Ion-Channel-Forming Peptides, ChemBioChem, 2006, 7, 433–435 CrossRef CAS PubMed
.
- K. Lee,
et al., Recent Progress in Solid-State Nanopores, Adv. Mater., 2018, 30, 1704680 CrossRef PubMed
.
- M. Mayer and J. Yang, Engineered Ion Channels as Emerging Tools for Chemical Biology, Acc. Chem. Res., 2013, 46, 2998–3008 CrossRef CAS PubMed
.
- M. X. Macrae,
et al., A Semi-Synthetic Ion Channel Platform for Detection of Phosphatase and Protease Activity, ACS Nano, 2009, 3, 3567–3580 CrossRef CAS PubMed
.
- L. Song,
et al., Structure of staphylococcal alpha-hemolysin, a heptameric transmembrane pore, Science, 1996, 274, 1859–1866 CrossRef CAS PubMed
.
- G. Huang, A. Voet and G. Maglia, FraC nanopores with adjustable diameter identify the mass of opposite-charge peptides with 44 dalton resolution, Nat. Commun., 2019, 10, 835 CrossRef PubMed
.
- S. K. Nomidis, J. Hooyberghs, G. Maglia and E. Carlon, DNA capture into the ClyA nanopore: diffusion-limited versus reaction-limited processes, J. Phys.: Condens. Matter, 2018, 30, 304001 CrossRef PubMed
.
- O. Braha,
et al., Simultaneous stochastic sensing of divalent metal ions, Nat. Biotechnol., 2000, 18, 1005–1007 CrossRef CAS PubMed
.
- G. Baaken,
et al., High-Resolution Size-Discrimination of Single Nonionic Synthetic Polymers with a Highly Charged Biological Nanopore, ACS Nano, 2015, 9, 6443–6449 CrossRef CAS PubMed
.
- R. Stefureac, Y. Long, H.-B. Kraatz, P. Howard and J. S. Lee, Transport of α-Helical Peptides through α-Hemolysin and Aerolysin Pores, Biochemistry, 2006, 45, 9172–9179 CrossRef CAS PubMed
.
- F. Haque, J. Li, H.-C. Wu, X.-J. Liang and P. Guo, Solid-state and biological nanopore for real-time sensing of single chemical and sequencing of DNA, Nano Today, 2013, 8, 56–74 CrossRef CAS
.
-
G. Maglia, A. J. Heron, D. Stoddart, D. Japrung and H. Bayley, Chapter 22 - Analysis of Single Nucleic Acid Molecules with Protein Nanopores, in Methods in Enzymology, ed. N. G. Walter, Academic Press, 2010, vol. 475, pp. 591–623 Search PubMed
.
- G. F. Schneider and C. Dekker, DNA sequencing with nanopores, Nat. Biotechnol., 2012, 30, 326–328 CrossRef CAS PubMed
.
- M. Wanunu, Nanopores: A journey towards DNA sequencing, Phys. Life Rev., 2012, 9, 125–158 CrossRef PubMed
.
- Y. Wang, D. Zheng, Q. Tan, M. X. Wang and L.-Q. Gu, Nanopore-based detection of circulating microRNAs in lung cancer patients, Nat. Nanotechnol., 2011, 6, 668–674 CrossRef CAS PubMed
.
- M. Mueller, U. Grauschopf, T. Maier, R. Glockshuber and N. Ban, The structure of a cytolytic α-helical toxin pore reveals its assembly mechanism, Nature, 2009, 459, 726–730 CrossRef CAS PubMed
.
- I. Iacovache,
et al., Cryo-EM structure of aerolysin variants reveals a novel protein fold and the pore-formation process, Nat. Commun., 2016, 7, 12062 CrossRef CAS PubMed
.
- A. A. Simpson,
et al., Structure determination of the head-tail connector of bacteriophage phi29, Acta Crystallogr., Sect. D: Biol. Crystallogr., 2001, 57, 1260–1269 CrossRef CAS PubMed
.
- P. Goyal,
et al., Structural and mechanistic insights into the bacterial amyloid secretion channel CsgG, Nature, 2014, 516, 250–253 CrossRef CAS PubMed
.
- M. Faller, M. Niederweis and G. E. Schulz, The Structure of a Mycobacterial Outer-Membrane Channel, Science, 2004, 303, 1189–1192 CrossRef CAS
.
- K. Tanaka, J. M. M. Caaveiro, K. Morante, J. M. González-Mañas and K. Tsumoto, Structural basis for self-assembly of a cytolytic pore lined by protein and lipid, Nat. Commun., 2015, 6, 6337 CrossRef PubMed
.
- L.-Q. Gu, S. Cheley and H. Bayley, Prolonged Residence Time of a Noncovalent Molecular Adapter, β-Cyclodextrin, within the Lumen of Mutant α-Hemolysin Pores, J. Gen. Physiol., 2001, 118, 481–494 CrossRef CAS PubMed
.
- B. Walker and H. Bayley, Key Residues for Membrane Binding, Oligomerization, and Pore Forming Activity of Staphylococcal α-Hemolysin Identified by Cysteine Scanning Mutagenesis and Targeted Chemical Modification, J. Biol. Chem., 1995, 270, 23065–23071 CrossRef CAS PubMed
.
-
C. Miller, Ion Channel Reconstitution, 1986 Search PubMed
.
- J. Li,
et al., Ion-beam sculpting at nanometre length scales, Nature, 2001, 412, 166–169 CrossRef CAS PubMed
.
- J. D. Uram, K. Ke, A. J. Hunt and M. Mayer, Label-Free Affinity Assays by Rapid Detection of Immune Complexes in Submicrometer Pores, Angew. Chem., Int. Ed., 2006, 45, 2281–2285 CrossRef CAS PubMed
.
- J. D. Uram, K. Ke, A. J. Hunt and M. Mayer, Submicrometer Pore-Based Characterization and Quantification of Antibody–Virus Interactions, Small, 2006, 2, 967–972 CrossRef CAS PubMed
.
- L. J. de Vreede,
et al., Wafer-scale fabrication of fused silica chips for low-noise recording of resistive pulses through nanopores, Nanotechnology, 2019, 30, 265301 CrossRef CAS PubMed
.
- J. D. Uram and M. Mayer, Estimation of solid phase affinity constants using resistive-pulses from functionalized nanoparticles, Biosens. Bioelectron., 2007, 22, 1556–1560 CrossRef CAS
.
- F. Bian,
et al., Ultrasmall Silver Nanopores Fabricated by Femtosecond Laser Pulses, Nano Lett., 2011, 11, 3251–3257 CrossRef CAS PubMed
.
- I. Perez,
et al., TEM-Based Metrology for HfO2 Layers and Nanotubes Formed in Anodic Aluminum Oxide Nanopore Structures, Small, 2008, 4, 1223–1232 CrossRef CAS PubMed
.
- J. Feng,
et al., Electrochemical Reaction in Single Layer MoS2: Nanopores Opened Atom by Atom, Nano Lett., 2015, 15, 3431–3438 CrossRef CAS PubMed
.
- P. Actis, A. C. Mak and N. Pourmand, Functionalized nanopipettes: toward label-free, single cell biosensors, Bioanal. Rev., 2010, 1, 177–185 CrossRef PubMed
.
- B. M. Venkatesan,
et al., Highly Sensitive, Mechanically Stable Nanopore Sensors for DNA Analysis, Adv. Mater., 2009, 21, 2771–2776 CrossRef CAS PubMed
.
- R. An,
et al., Ultrafast laser fabrication of submicrometer pores in borosilicate glass, Opt. Lett., 2008, 33, 1153–1155 CrossRef PubMed
.
- R. Torre, J. dela Larkin, A. Singer and A. Meller, Fabrication and characterization of solid-state nanopore arrays for high-throughput DNA sequencing, Nanotechnology, 2012, 23, 385308 CrossRef PubMed
.
- A. J. Storm, J. H. Chen, X. S. Ling, H. W. Zandbergen and C. Dekker, Fabrication of solid-state nanopores with single-nanometre precision, Nat. Mater., 2003, 2, 537–540 CrossRef CAS PubMed
.
- X. Hou, W. Guo and L. Jiang, Biomimetic smart nanopores and nanochannels, Chem. Soc. Rev., 2011, 40, 2385–2401 RSC
.
- C. J. Lo, T. Aref and A. Bezryadin, Fabrication of symmetric sub-5 nm nanopores using focused ion and electron beams, Nanotechnology, 2006, 17, 3264 CrossRef CAS
.
- J. Shim, J. A. Rivera and R. Bashir, Electron beam induced local crystallization of HfO2 nanopores for biosensing applications, Nanoscale, 2013, 5, 10887–10893 RSC
.
- M.-Y. Wu, D. Krapf, M. Zandbergen, H. Zandbergen and P. E. Batson, Formation of nanopores in a SiN/SiO2 membrane with an electron beam, Appl. Phys. Lett., 2005, 87, 113106 CrossRef
.
- B. M. Venkatesan, A. B. Shah, J.-M. Zuo and R. Bashir, DNA Sensing Using Nanocrystalline Surface-Enhanced Al2O3 Nanopore Sensors, Adv. Funct. Mater., 2010, 20, 1266–1275 CrossRef CAS PubMed
.
- L. T. Sexton, L. P. Horne and C. R. Martin, Developing synthetic conical nanopores for biosensing applications, Mol. Biosyst., 2007, 3, 667–685 RSC
.
- J. E. Wharton,
et al., A Method for Reproducibly Preparing Synthetic Nanopores for Resistive-Pulse Biosensors, Small, 2007, 3, 1424–1430 CrossRef CAS PubMed
.
- C. C. Harrell, S. B. Lee and C. R. Martin, Synthetic Single-Nanopore and Nanotube Membranes, Anal. Chem., 2003, 75, 6861–6867 CrossRef CAS PubMed
.
- P. Y. Apel,
et al., Effect of nanosized surfactant molecules on the etching of ion tracks: New degrees of freedom in design of pore shape, Nucl. Instrum. Methods Phys. Res., Sect. B, 2003, 209, 329–334 CrossRef CAS
.
- P. Scopece, L. A. Baker, P. Ugo and C. R. Martin, Conical nanopore membranes: solvent shaping of nanopores, Nanotechnology, 2006, 17, 3951 CrossRef CAS
.
- H. Kwok, K. Briggs and V. Tabard-Cossa, Nanopore Fabrication by Controlled Dielectric Breakdown, PLoS One, 2014, 9, e92880 CrossRef PubMed
.
- C. Ying,
et al., Formation of Single Nanopores with Diameters of 20–50 nm in Silicon Nitride Membranes Using Laser-Assisted Controlled Breakdown, ACS Nano, 2018, 12, 11458–11470 CrossRef CAS PubMed
.
- T. Gilboa, E. Zvuloni, A. Zrehen, A. H. Squires and A. Meller, Automated, Ultra-Fast Laser-Drilling of Nanometer Scale Pores and Nanopore Arrays in Aqueous Solutions, Adv. Funct. Mater., 2019, 1900642 CrossRef
.
- H. Yamazaki, R. Hu, Q. Zhao and M. Wanunu, Photothermally Assisted Thinning of Silicon Nitride Membranes for Ultrathin Asymmetric Nanopores, ACS Nano, 2018, 12, 12472–12481 CrossRef CAS
.
- J. Gao,
et al., Layer-by-layer removal of insulating few-layer mica flakes for asymmetric ultra-thin nanopore fabrication, Nano Res., 2011, 5, 99–108 CrossRef
.
- N. Li, S. Yu, C. C. Harrell and C. R. Martin, Conical Nanopore Membranes, Preparation and Transport Properties, Anal. Chem., 2004, 76, 2025–2030 CrossRef CAS PubMed
.
- G. F. Schneider,
et al., DNA Translocation through Graphene Nanopores, Nano Lett., 2010, 10, 3163–3167 CrossRef CAS PubMed
.
- M. J. Kim, M. Wanunu, D. C. Bell and A. Meller, Rapid Fabrication of Uniformly Sized Nanopores and Nanopore Arrays for Parallel DNA Analysis, Adv. Mater., 2006, 18, 3149–3153 CrossRef CAS
.
- R. Wei, D. Pedone, A. Zürner, M. Döblinger and U. Rant, Fabrication of Metallized Nanopores in Silicon Nitride Membranes for Single-Molecule Sensing, Small, 2010, 6, 1406–1414 CrossRef CAS PubMed
.
- J. Larkin,
et al., Slow DNA Transport through Nanopores in Hafnium Oxide Membranes, ACS Nano, 2013, 7, 10121–10128 CrossRef CAS PubMed
.
- J. Yan,
et al., Growth of Patterned Nanopore Arrays of Anodic Aluminum Oxide, Adv. Mater., 2003, 15, 2015–2018 CrossRef CAS
.
- C. J. Russo and J. A. Golovchenko, Atom-by-atom nucleation and growth of graphene nanopores, Proc. Natl. Acad. Sci. U. S. A., 2012, 109, 5953–5957 CrossRef CAS PubMed
.
- Z. S. Siwy and M. Davenport, Nanopores: Graphene opens up to DNA, Nat. Nanotechnol., 2010, 5, 697–698 CrossRef CAS PubMed
.
- J. D. Uram, K. Ke and M. Mayer, Noise and Bandwidth of Current Recordings from Submicrometer Pores and Nanopores, ACS Nano, 2008, 2, 857–872 CrossRef CAS PubMed
.
- W. Bell and N. A,
et al., Multiplexed ionic current sensing with glass nanopores, Lab Chip, 2013, 13, 1859–1862 RSC
.
- B. Zhang,
et al., Bench-Top Method for Fabricating Glass-Sealed Nanodisk Electrodes, Glass Nanopore Electrodes, and Glass Nanopore Membranes of Controlled Size, Anal. Chem., 2007, 79, 4778–4787 CrossRef CAS PubMed
.
- A. Mara, Z. Siwy, C. Trautmann, J. Wan and F. Kamme, An Asymmetric Polymer Nanopore for Single Molecule Detection, Nano Lett., 2004, 4, 497–501 CrossRef CAS
.
- B. Schiedt, K. Healy, A. P. Morrison, R. Neumann and Z. Siwy, Transport of ions and biomolecules through single asymmetric nanopores in polymer films, Nucl. Instrum. Methods Phys. Res., Sect. B, 2005, 236, 109–116 CrossRef CAS
.
- V. Hlady and J. Buijs, Protein adsorption on solid surfaces, Curr. Opin. Biotechnol., 1996, 7, 72–77 CrossRef CAS
.
- Z. Siwy, I. D. Kosińska, A. Fuliński and C. R. Martin, Asymmetric Diffusion through Synthetic Nanopores, Phys. Rev. Lett., 2005, 94, 048102 CrossRef CAS PubMed
.
- A. J. Storm,
et al., Fast DNA translocation through a solid-state nanopore, Nano Lett., 2005, 5, 1193–1197 CrossRef CAS PubMed
.
- D. Fologea, J. Uplinger, B. Thomas, D. S. McNabb and J. Li, Slowing DNA Translocation in a Solid-State Nanopore, Nano Lett., 2005, 5, 1734–1737 CrossRef CAS PubMed
.
- W. Sparreboom, A. van den Berg and J. C. T. Eijkel, Principles and applications of nanofluidic transport, Nat. Nanotechnol., 2009, 4, 713–720 CrossRef CAS PubMed
.
- A. Ramachandran, Y. Liu, W. Asghar and S. Iqbal, Characterization of DNA-Nanopore Interactions by Molecular Dynamics, Am. J. Biomed. Sci., 2009, 344–351, DOI:10.5099/aj090400344
.
- S. Narayanan, A. G. Fedorov and Y. K. Joshi, Interfacial Transport of Evaporating Water Confined in Nanopores, Langmuir, 2011, 27, 10666–10676 CrossRef CAS PubMed
.
- M. R. Powell, L. Cleary, M. Davenport, K. J. Shea and Z. S. Siwy, Electric-field-induced wetting and dewetting in single hydrophobic nanopores, Nat. Nanotechnol., 2011, 6, 798–802 CrossRef CAS
.
- S. Vaitheeswaran and D. Thirumalai, Interactions between amino acid side chains in cylindrical hydrophobic nanopores with applications to peptide stability, Proc. Natl. Acad. Sci. U. S. A., 2008, 105, 17636–17641 CrossRef CAS PubMed
.
- A. Aksimentiev, J. B. Heng, G. Timp and K. Schulten, Microscopic Kinetics of DNA Translocation through Synthetic Nanopores, Biophys. J., 2004, 87, 2086–2097 CrossRef CAS
.
- E. C. Yusko,
et al., Controlling protein translocation through nanopores with bio-inspired fluid walls, Nat. Nanotechnol., 2011, 6, 253–260 CrossRef CAS PubMed
.
- M. R. Powell,
et al., Nanoprecipitation-assisted ion current oscillations, Nat. Nanotechnol., 2008, 3, 51–57 CrossRef CAS PubMed
.
- H. Daiguji, P. Yang and A. Majumdar, Ion Transport in Nanofluidic Channels, Nano Lett., 2004, 4, 137–142 CrossRef CAS
.
- H. Daiguji, P. Yang, A. J. Szeri and A. Majumdar, Electrochemomechanical Energy Conversion in Nanofluidic Channels, Nano Lett., 2004, 4, 2315–2321 CrossRef CAS
.
- D. V. Melnikov, Z. K. Hulings and M. E. Gracheva, Electro-osmotic flow through nanopores in thin and ultrathin membranes, Phys. Rev. E, 2017, 95, 063105 CrossRef PubMed
.
- A. Asandei,
et al., Electroosmotic Trap Against the Electrophoretic Force Near a Protein Nanopore Reveals Peptide Dynamics During Capture and Translocation, ACS Appl. Mater. Interfaces, 2016, 8, 13166–13179 CrossRef CAS PubMed
.
- E. C. Yusko, Y. N. Billeh and M. Mayer, Current oscillations generated by precipitate formation in the mixing zone between two solutions inside a nanopore, J. Phys.: Condens. Matter, 2010, 22, 454127 CrossRef PubMed
.
- O. M. Eggenberger,
et al., Fluid surface coatings for solid-state nanopores: comparison of phospholipid bilayers and archaea-Inspired lipid monolayers, Nanotechnology, 2019, 30, 325504 CrossRef PubMed
.
- Z. Siwy, E. Heins, C. C. Harrell, P. Kohli and C. R. Martin, Conical-Nanotube Ion-Current Rectifiers: The Role of Surface Charge, J. Am. Chem. Soc., 2004, 126, 10850–10851 CrossRef CAS
.
- H. S. White and A. Bund, Ion Current Rectification at Nanopores in Glass Membranes, Langmuir, 2008, 24, 2212–2218 CrossRef CAS PubMed
.
- Y. Ai, M. Zhang, S. W. Joo, M. A. Cheney and S. Qian, Effects of Electroosmotic Flow on Ionic Current Rectification in Conical Nanopores, J. Phys. Chem. C, 2010, 114, 3883–3890 CrossRef CAS
.
-
J.-F. Pietschmann
, et al., Rectification properties of conically shaped nanopores: consequences of miniaturization, 2012, ArXiv12094164 Cond-Mat Physicsphysics.
- E. C. Yusko, R. An and M. Mayer, Electroosmotic Flow Can Generate Ion Current Rectification in Nano- and Micropores, ACS Nano, 2010, 4, 477–487 CrossRef CAS PubMed
.
- M. X. Macrae, S. Blake, M. Mayer and J. Yang, Nanoscale Ionic Diodes with Tunable and Switchable Rectifying Behavior, J. Am. Chem. Soc., 2010, 132, 1766–1767 CrossRef CAS PubMed
.
- Z. S. Siwy, Ion-Current Rectification in Nanopores and Nanotubes with Broken Symmetry, Adv. Funct. Mater., 2006, 16, 735–746 CrossRef CAS
.
- P. Y. Apel, Y. E. Korchev, Z. Siwy, R. Spohr and M. Yoshida, Diode-like single-ion track membrane prepared by electro-stopping, Nucl. Instrum. Methods Phys. Res., Sect. B, 2001, 184, 337–346 CrossRef CAS
.
- I. Vlassiouk and Z. S. Siwy, Nanofluidic Diode, Nano Lett., 2007, 7, 552–556 CrossRef CAS PubMed
.
- Y. Tian, X. Hou and L. Jiang, Biomimetic ionic rectifier systems: Asymmetric modification of single nanochannels by ion sputtering technology, J. Electroanal. Chem., 2011, 656, 231–236 CrossRef CAS
.
- X. Hou, H. Dong, D. Zhu and L. Jiang, Fabrication of Stable Single Nanochannels with Controllable Ionic Rectification, Small, 2010, 6, 361–365 CrossRef CAS PubMed
.
- Z. Zhang,
et al., A Bioinspired Multifunctional Heterogeneous Membrane with Ultrahigh Ionic Rectification and Highly Efficient Selective Ionic Gating, Adv. Mater., 2016, 28, 144–150 CrossRef CAS PubMed
.
- K. Kececi, L. T. Sexton, F. Buyukserin and C. R. Martin, Resistive-pulse detection of short dsDNAs using a chemically functionalized conical nanopore sensor, Nanomedicine, 2008, 3, 787–796 CrossRef CAS PubMed
.
- Y. Xie,
et al., Surface Modification of Single Track-Etched Nanopores with Surfactant CTAB, Langmuir, 2009, 25, 8870–8874 CrossRef CAS PubMed
.
- B. N. Anderson, M. Muthukumar and A. Meller, pH Tuning of DNA Translocation Time through Organically Functionalized Nanopores, ACS Nano, 2013, 7, 1408–1414 CrossRef CAS PubMed
.
- V. Thangaraj,
et al., Detection of short ssDNA and dsDNA by current-voltage measurements using conical nanopores coated with Al2O3 by atomic layer deposition, Microchim. Acta, 2015, 183, 1011–1017 CrossRef
.
- E. Laarz, B. V. Zhmud and L. Bergström, Dissolution and Deagglomeration of Silicon Nitride in Aqueous Medium, J. Am. Ceram. Soc., 2000, 83, 2394–2400 CrossRef CAS
.
- B. V. Zhmud and L. Bergström, Dissolution Kinetics of Silicon Nitride in Aqueous Suspension, J. Colloid Interface Sci., 1999, 218, 582–584 CrossRef CAS PubMed
.
- S. W. Kowalczyk, T. R. Blosser and C. Dekker, Biomimetic nanopores: learning from and about nature, Trends Biotechnol., 2011, 29, 607–614 CrossRef CAS PubMed
.
- X. Hou, H. Zhang and L. Jiang, Building Bio-Inspired Artificial Functional Nanochannels: From Symmetric to Asymmetric Modification, Angew. Chem., Int. Ed., 2012, 51, 5296–5307 CrossRef CAS
.
- R. E. Gyurcsányi, Chemically-modified nanopores for sensing, TrAC, Trends Anal. Chem., 2008, 27, 627–639 CrossRef
.
- A. Kocer, L. Tauk and P. Déjardin, Nanopore sensors: From hybrid to abiotic systems, Biosens. Bioelectron., 2012, 38, 1–10 CrossRef CAS
.
- M. Tagliazucchi and I. Szleifer, Transport mechanisms in nanopores and nanochannels: can we mimic nature? Mater, Today, 2015, 18, 131–142 CAS
.
- M. Lepoitevin, T. Ma, M. Bechelany, J.-M. Janot and S. Balme, Functionalization of single solid state nanopores to mimic biological ion channels: A review, Adv. Colloid Interface Sci., 2017, 250, 195–213 CrossRef CAS PubMed
.
- G. Jeon, S. Y. Yang and J. K. Kim, Functional nanoporous membranes for drug delivery, J. Mater. Chem., 2012, 22, 14814–14834 RSC
.
- A. Asatekin and K. K. Gleason, Polymeric Nanopore Membranes for Hydrophobicity-Based Separations by Conformal Initiated Chemical Vapor Deposition, Nano Lett., 2011, 11, 677–686 CrossRef CAS
.
- S. Banerjee,
et al., Slowing DNA Transport Using Graphene–DNA Interactions, Adv. Funct. Mater., 2015, 25, 936–946 CrossRef CAS
.
- P. Chen,
et al., Atomic Layer Deposition to Fine-Tune the Surface Properties and Diameters of Fabricated Nanopores, Nano Lett., 2004, 4, 1333–1337 CrossRef CAS
.
- Z. Chen,
et al., DNA translocation through an array of kinked nanopores, Nat. Mater., 2010, 9, 667–675 CrossRef CAS
.
- J. W. Elam, D. Routkevitch, P. P. Mardilovich and S. M. George, Conformal Coating on Ultrahigh-Aspect-Ratio Nanopores of Anodic Alumina by Atomic Layer Deposition, Chem. Mater., 2003, 15, 3507–3517 CrossRef CAS
.
- J. W. Elam,
et al., Atomic Layer Deposition for the Conformal Coating of Nanoporous Materials, J. Nanomater., 2006, 2006, e64501 Search PubMed
.
- R. Kox, C. Chen, G. Maes, L. Lagae and G. Borghs, Shrinking solid-state nanopores using electron-beam-induced deposition, Nanotechnology, 2009, 20, 115302 CrossRef PubMed
.
- R. Kox,
et al., Local solid-state modification of nanopore surface charges, Nanotechnology, 2010, 21, 335703 CrossRef PubMed
.
- D. Coglitore,
et al., Metal alloy solid-state nanopores for single nanoparticle detection, Phys. Chem. Chem. Phys., 2018, 20, 12799–12807 RSC
.
- P. Waduge,
et al., Direct and Scalable Deposition of Atomically Thin Low-Noise MoS2 Membranes on Apertures, ACS Nano, 2015, 9, 7352–7359 CrossRef CAS PubMed
.
- C. Wang,
et al., Atomic Layer Deposition Modified Track-Etched Conical Nanochannels for Protein Sensing, Anal. Chem., 2015, 87, 8227–8233 CrossRef CAS PubMed
.
- M. Weber,
et al., Boron Nitride Nanoporous Membranes with High Surface Charge by Atomic Layer Deposition, ACS Appl. Mater. Interfaces, 2017, 9, 16669–16678 CrossRef CAS PubMed
.
- E. B. Kalman, O. Sudre, I. Vlassiouk and Z. S. Siwy, Control of ionic transport through gated single conical nanopores, Anal. Bioanal. Chem., 2008, 394, 413–419 CrossRef
.
- C. Fan,
et al., Achieving Uniform and Conformal ALD Coatings on Sub-10 nm Pores Using Dual-Stage Exposure/Purge at Optimized Growth Temperatures, MRS Adv., 2018, 3, 2833–2839 CrossRef CAS
.
- S. Zeng,
et al., Controlled size reduction and its underlying mechanism to form solid-state nanopores via electron beam induced carbon deposition, Nanotechnology, 2019, 30, 455303 CrossRef PubMed
.
- R. Hu,
et al., Intrinsic and membrane-facilitated α-synuclein oligomerization revealed by label-free detection through solid-state nanopores, Sci. Rep., 2016, 6, 20776 CrossRef CAS PubMed
.
- X. Li,
et al., Non-sticky translocation of bio-molecules through Tween 20-coated solid-state nanopores in a wide pH range, Appl. Phys. Lett., 2016, 109, 143105 CrossRef
.
- G. F. Schneider,
et al., Tailoring the hydrophobicity of graphene for its use as nanopores for DNA translocation, Nat. Commun., 2013, 4, 2619 CrossRef PubMed
.
- P. Actis,
et al., Reversible thrombin detection by aptamer functionalized STING sensors, Biosens. Bioelectron., 2011, 26, 4503–4507 CrossRef CAS PubMed
.
- S. Umehara,
et al., Current Rectification with Poly-l-Lysine-Coated Quartz Nanopipettes, Nano Lett., 2006, 6, 2486–2492 CrossRef CAS
.
- P. Actis, O. Jejelowo and N. Pourmand, UltraSensitive Mycotoxin Detection by STING Sensors, Biosens. Bioelectron., 2010, 26, 333–337 CrossRef CAS
.
- P. Actis,
et al., Voltage-Controlled Metal Binding on Polyelectrolyte-Functionalized Nanopores, Langmuir, 2011, 27, 6528–6533 CrossRef CAS
.
- B. Vilozny, P. Actis, R. A. Seger, Q. Vallmajo-Martin and N. Pourmand, Reversible Cation Response with a Protein-Modified Nanopipette, Anal. Chem., 2011, 83, 6121–6126 CrossRef CAS
.
- S. Umehara, M. Karhanek, R. W. Davis and N. Pourmand, Label-free biosensing with functionalized nanopipette probes, Proc. Natl. Acad. Sci. U. S. A., 2009, 106, 4611–4616 CrossRef CAS
.
- H. Alem, F. Blondeau, K. Glinel, S. Demoustier-Champagne and A. M. Jonas, Layer-by-Layer Assembly of Polyelectrolytes in Nanopores, Macromolecules, 2007, 40, 3366–3372 CrossRef CAS
.
- M. Ali,
et al., Layer-by-Layer Assembly of Polyelectrolytes into Ionic Current Rectifying Solid-State Nanopores: Insights from Theory and Experiment, J. Am. Chem. Soc., 2010, 132, 8338–8348 CrossRef CAS PubMed
.
- E. L. C. J. Blundell, L. Mayne, M. Lickorish, S. Christie and M. Platt, Protein detection using Tunable Pores: Resistive Pulses and Current Rectification, Faraday Discuss., 2016, 193, 487–505 RSC
.
- M. Lepoitevin,
et al., Fast and reversible functionalization of a single nanopore based on layer-by-layer polyelectrolyte self-assembly for tuning current rectification and designing sensors, RSC Adv., 2016, 6, 32228–32233 RSC
.
- Y. Zhao, J.-M. Janot, E. Balanzat and S. Balme, Mimicking pH-Gated Ionic Channels by Polyelectrolyte Complex Confinement Inside a Single Nanopore, Langmuir, 2017, 33, 3484–3490 CrossRef CAS PubMed
.
- T. Ma,
et al., Impact of Polyelectrolyte Multilayers on the Ionic Current Rectification of Conical Nanopores, Langmuir, 2018, 34, 3405–3412 CrossRef CAS PubMed
.
- N. Das, R. Ray, S. Ray and C. R. Chaudhuri, Intelligent Quantification of Picomolar Protein Concentration in Serum by Functionalized Nanopores, IEEE Sens. J., 2018, 1–1, DOI:10.1109/JSEN.2018.2872853
.
- N. Liu,
et al., Photoregulation of Mass Transport through a Photoresponsive Azobenzene-Modified Nanoporous Membrane, Nano Lett., 2004, 4, 551–554 CrossRef CAS
.
- M. Wanunu and A. Meller, Chemically Modified Solid-State Nanopores, Nano Lett., 2007, 7, 1580–1585 CrossRef CAS PubMed
.
- S. Ding, C. Gao and L.-Q. Gu, Capturing Single Molecules of Immunoglobulin and Ricin with an Aptamer-Encoded Glass Nanopore, Anal. Chem., 2009, 81, 6649–6655 CrossRef CAS PubMed
.
- Y. Fu, H. Tokuhisa and L. A. Baker, Nanopore DNA sensors based on dendrimer-modified nanopipettes, Chem. Commun., 2009, 4877–4879, 10.1039/B910511E
.
- S. M. Iqbal, D. Akin and R. Bashir, Solid-state nanopore channels with DNA selectivity, Nat. Nanotechnol., 2007, 2, 243–248 CrossRef CAS
.
- S. W. Kowalczyk,
et al., Single-molecule transport across an individual biomimetic nuclear pore complex, Nat. Nanotechnol., 2011, 6, 433–438 CrossRef CAS
.
- S. B. Lee,
et al., Antibody-Based Bio-Nanotube Membranes for Enantiomeric Drug Separations, Science, 2002, 296, 2198–2200 CrossRef CAS PubMed
.
- J. Nilsson, J. R. I. Lee, T. V. Ratto and S. E. Létant, Localized Functionalization of Single Nanopores, Adv. Mater., 2006, 18, 427–431 CrossRef CAS
.
- S. Tan, L. Wang, H. Liu, H. Wu and Q. Liu, Single Nanoparticle Translocation Through Chemically Modified Solid Nanopore, Nanoscale Res. Lett., 2016, 11, 50 CrossRef
.
- G. Wang, B. Zhang, J. R. Wayment, J. M. Harris and H. S. White, Electrostatic-Gated Transport in Chemically Modified Glass Nanopore Electrodes, J. Am. Chem. Soc., 2006, 128, 7679–7686 CrossRef CAS PubMed
.
- G. Wang, A. K. Bohaty, I. Zharov and H. S. White, Photon Gated Transport at the Glass Nanopore Electrode, J. Am. Chem. Soc., 2006, 128, 13553–13558 CrossRef CAS
.
- L.-X. Zhang, S.-L. Cai, Y.-B. Zheng, X.-H. Cao and Y.-Q. Li, Smart Homopolymer Modification to Single Glass Conical Nanopore Channels: Dual-Stimuli-Actuated Highly Efficient Ion Gating, Adv. Funct. Mater., 2011, 21, 2103–2107 CrossRef CAS
.
- V. Mussi,
et al., “DNA-Dressed NAnopore”
for complementary sequence detection, Biosens. Bioelectron., 2011, 29, 125–131 CrossRef CAS PubMed
.
- N. Giamblanco,
et al., Detection of protein aggregate morphology through single antifouling nanopore, Sens. Actuators, B, 2018, 260, 736–745 CrossRef CAS
.
- S. Balme,
et al., Unexpected ionic transport behavior in hydrophobic and uncharged conical nanopores, Faraday Discuss., 2018, 210, 69–85 RSC
.
- G.-C. Liu,
et al., Ion-current-rectification-based customizable pH response in glass nanopipettes via silanization, Electrochem. Commun., 2018, 93, 95–99 CrossRef CAS
.
- G.-C. Liu,
et al., pH-modulated ion-current rectification in a cysteine-functionalized glass nanopipette, Electrochem. Commun., 2018, 97, 6–10 CrossRef CAS
.
- A. Ananth,
et al., Reversible Immobilization of Proteins in Sensors and Solid-State Nanopores, Small, 2018, 14, 1703357 CrossRef PubMed
.
- Y. Liebes-Peer, H. Rapaport and N. Ashkenasy, Amplification of Single Molecule Translocation Signal Using β-Strand Peptide Functionalized Nanopores, ACS Nano, 2014, 8, 6822–6832 CrossRef CAS PubMed
.
- Z. Tang,
et al., Surface Modification of Solid-State Nanopores for Sticky-Free Translocation of Single-Stranded DNA, Small, 2014, 10, 4332–4339 CAS
.
- S. Zhang,
et al., Detection of alkaline phosphatase activity with a functionalized nanopipette, Electrochem. Commun., 2019, 99, 71–74 CrossRef CAS
.
- W. Guo,
et al., Current Rectification in Temperature-Responsive Single Nanopores, ChemPhysChem, 2010, 11, 859–864 CrossRef CAS PubMed
.
- H. He, X. Xu, P. Wang, L. Chen and Y. Jin, The facile surface chemical modification of a single glass nanopore and its use in the nonenzymatic detection of uric acid, Chem. Commun., 2015, 51, 1914–1917 RSC
.
- M. Nishizawa, V. P. Menon and C. R. Martin, Metal Nanotubule Membranes with Electrochemically Switchable Ion-Transport Selectivity, Science, 1995, 268, 700–702 CrossRef CAS PubMed
.
- J. C. Hulteen, K. B. Jirage and C. R. Martin, Introducing Chemical Transport Selectivity into Gold Nanotubule Membranes, J. Am. Chem. Soc., 1998, 120, 6603–6604 CrossRef CAS
.
- K. B. Jirage, J. C. Hulteen and C. R. Martin, Effect of Thiol Chemisorption on the Transport Properties of Gold Nanotubule Membranes, Anal. Chem., 1999, 71, 4913–4918 CrossRef CAS PubMed
.
- S. B. Lee and C. R. Martin, pH-Switchable, Ion-Permselective Gold Nanotubule Membrane Based on Chemisorbed Cysteine, Anal. Chem., 2001, 73, 768–775 CrossRef CAS
.
- C. R. Martin, M. Nishizawa, K. Jirage, M. Kang and S. B. Lee, Controlling Ion-Transport Selectivity in Gold Nanotubule Membranes, Adv. Mater., 2001, 13, 1351–1362 CrossRef CAS
.
- S. Yu, S. B. Lee, M. Kang and C. R. Martin, Size-Based Protein Separations in Poly(ethylene glycol)-Derivatized Gold Nanotubule Membranes, Nano Lett., 2001, 1, 495–498 CrossRef CAS
.
- P. Kohli,
et al., DNA-Functionalized Nanotube Membranes with Single-Base Mismatch Selectivity, Science, 2004, 305, 984–986 CrossRef CAS PubMed
.
- Z. Siwy,
et al., Protein Biosensors Based on Biofunctionalized Conical Gold Nanotubes, J. Am. Chem. Soc., 2005, 127, 5000–5001 CrossRef CAS PubMed
.
- L. T. Sexton,
et al., Resistive-Pulse Studies of Proteins and Protein/Antibody Complexes Using a Conical Nanotube Sensor, J. Am. Chem. Soc., 2007, 129, 13144–13152 CrossRef CAS PubMed
.
- T. Jovanovic-Talisman,
et al., Artificial nanopores that mimic the transport selectivity of the nuclear pore complex, Nature, 2009, 457, 1023–1027 CrossRef CAS PubMed
.
- R. Wei, V. Gatterdam, R. Wieneke, R. Tampé and U. Rant, Stochastic sensing of proteins with receptor-modified solid-state nanopores, Nat. Nanotechnol., 2012, 7, 257–263 CrossRef CAS PubMed
.
- G. Emilsson,
et al., Polymer brushes in solid-state nanopores form an impenetrable entropic barrier for proteins, Nanoscale, 2018, 10, 4663–4669 RSC
.
- G. Emilsson,
et al., Gating Protein Transport in Solid State Nanopores by Single Molecule Recognition, ACS Cent. Sci., 2018, 4, 1007–1014 CrossRef CAS
.
- X. Zambrana-Puyalto,
et al., Site-selective functionalization
of plasmonic nanopores for enhanced fluorescence emission rate and Förster resonance energy transfer, Nanoscale Adv., 2019, 1, 2454–2461 RSC
.
- Y. Pang and R. Gordon, Optical Trapping of a Single Protein, Nano Lett., 2012, 12, 402–406 CrossRef CAS PubMed
.
- B. Malekian,
et al., Detecting Selective Protein Binding Inside Plasmonic Nanopores: Toward a Mimic of the Nuclear Pore Complex, Front. Chem., 2018, 6, 637 CrossRef CAS
.
- X. Hou,
et al., A pH-Gating Ionic Transport Nanodevice: Asymmetric Chemical Modification of Single Nanochannels, Adv. Mater., 2010, 22, 2440–2443 CrossRef CAS PubMed
.
- X. Hou,
et al., A Biomimetic Asymmetric Responsive Single Nanochannel, J. Am. Chem. Soc., 2010, 132, 11736–11742 CrossRef CAS PubMed
.
- S. F. Buchsbaum, G. Nguyen, S. Howorka and Z. S. Siwy, DNA-Modified Polymer Pores Allow pH- and Voltage-Gated Control of Channel Flux, J. Am. Chem. Soc., 2014, 136, 9902–9905 CrossRef CAS PubMed
.
- M. Lepoitevin, M. Bechelany, E. Balanzat, J.-M. Janot and S. Balme, Non-Fluorescence label protein sensing with track-etched nanopore decorated by avidin/biotin system, Electrochim. Acta, 2016, 211, 611–618 CrossRef CAS
.
- M. Lepoitevin,
et al., Combining a sensor and a pH-gated nanopore based on an avidin–biotin system, Chem. Commun., 2015, 51, 5994–5997 RSC
.
- B. Yameen,
et al., Ionic Transport Through Single Solid-State Nanopores Controlled with Thermally Nanoactuated Macromolecular Gates, Small, 2009, 5, 1287–1291 CrossRef CAS
.
- B. Yameen,
et al., Synthetic Proton-Gated Ion Channels via Single Solid-State Nanochannels Modified with Responsive Polymer Brushes, Nano Lett., 2009, 9, 2788–2793 CrossRef CAS PubMed
.
- M. Ali, R. Neumann and W. Ensinger, Sequence-Specific Recognition of DNA Oligomer Using Peptide Nucleic Acid (PNA)-Modified Synthetic Ion Channels: PNA/DNA Hybridization in Nanoconfined Environment, ACS Nano, 2010, 4, 7267–7274 CrossRef CAS PubMed
.
- M. Ali, S. Nasir and W. Ensinger, Bioconjugation-induced ionic current rectification in aptamer-modified single cylindrical nanopores, Chem. Commun., 2015, 51, 3454–3457 RSC
.
- M. Ali, S. Mafe, P. Ramirez, R. Neumann and W. Ensinger, Logic Gates Using Nanofluidic Diodes Based on Conical Nanopores Functionalized with Polyprotic Acid Chains, Langmuir, 2009, 25, 11993–11997 CrossRef CAS PubMed
.
- M. Ali, B. Schiedt, R. Neumann and W. Ensinger, Biosensing with Functionalized Single Asymmetric Polymer Nanochannels, Macromol. Biosci., 2010, 10, 28–32 CrossRef CAS
.
- M. Ali, Q. H. Nguyen, R. Neumann and W. Ensinger, ATP-modulated ionic transport through synthetic nanochannels, Chem. Commun., 2010, 46, 6690–6692 RSC
.
- M. Ali,
et al., Biomolecular conjugation inside synthetic polymer nanopores viaglycoprotein–lectin interactions, Nanoscale, 2011, 3, 1894–1903 RSC
.
- M. Ali,
et al., Hydrogen Peroxide Sensing with Horseradish Peroxidase-Modified Polymer Single Conical Nanochannels, Anal. Chem., 2011, 83, 1673–1680 CrossRef CAS PubMed
.
- M. Ali,
et al., Metal Ion Affinity-based Biomolecular Recognition and Conjugation inside Synthetic Polymer Nanopores Modified with Iron–Terpyridine Complexes, J. Am. Chem. Soc., 2011, 133, 17307–17314 CrossRef CAS
.
- S. Nasir, M. Ali and W. Ensinger, Thermally controlled permeation of ionic molecules through synthetic nanopores functionalized with amine-terminated polymer brushes, Nanotechnology, 2012, 23, 225502 CrossRef PubMed
.
- M. Ali,
et al., Single cigar-shaped nanopores functionalized with amphoteric amino acid chains: experimental and theoretical characterization, ACS Nano, 2012, 6, 3631–3640 CrossRef CAS PubMed
.
- M. Ali,
et al., Calcium Binding and Ionic Conduction in Single Conical Nanopores with Polyacid Chains: Model and Experiments, ACS Nano, 2012, 6, 9247–9257 CrossRef CAS PubMed
.
- M. N. Tahir,
et al., Silicatein conjugation inside nanoconfined geometries through immobilized NTA–Ni(II) chelates, Chem. Commun., 2013, 49, 2210–2212 RSC
.
- M. Ali,
et al., Carbohydrate-Mediated Biomolecular Recognition and Gating of Synthetic Ion Channels, J. Phys. Chem. C, 2013, 117, 18234–18242 CrossRef CAS
.
- M. Ali, S. Nasir, I. Ahmed, L. Fruk and W. Ensinger, Tuning nanopore surface polarity and rectification properties through enzymatic hydrolysis inside nanoconfined geometries, Chem. Commun., 2013, 49, 8770–8772 RSC
.
- M. Ali, S. Nasir and W. Ensinger, Stereoselective detection of amino acids with protein-modified single asymmetric nanopores, Electrochim. Acta, 2016, 215, 231–237 CrossRef CAS
.
- M. Ali,
et al., Label-free histamine detection with nanofluidic diodes through metal ion displacement mechanism, Colloids Surf., B, 2017, 150, 201–208 CrossRef CAS
.
- M. Ali,
et al., A redox-sensitive nanofluidic diode based on nicotinamide-modified asymmetric nanopores, Sens. Actuators, B, 2017, 240, 895–902 CrossRef CAS
.
- M. Ali,
et al., Potassium-induced ionic conduction through a single nanofluidic pore modified with acyclic polyether derivative, Anal. Chim. Acta, 2018, 1039, 132–139 CrossRef CAS
.
- F. Xia,
et al., Gating of Single Synthetic Nanopores by Proton-Driven DNA Molecular Motors, J. Am. Chem. Soc., 2008, 130, 8345–8350 CrossRef CAS PubMed
.
- X. Hou,
et al., A Biomimetic Potassium Responsive Nanochannel: G-Quadruplex DNA Conformational Switching in a Synthetic Nanopore, J. Am. Chem. Soc., 2009, 131, 7800–7805 CrossRef CAS PubMed
.
- Y. Tian,
et al., A biomimetic zinc activated ion channel, Chem. Commun., 2010, 46, 1682–1684 RSC
.
- W. Guo,
et al., Integrating Ionic Gate and Rectifier Within One Solid-State Nanopore via Modification with Dual-Responsive Copolymer Brushes, Adv. Funct. Mater., 2010, 20, 3561–3567 CrossRef CAS
.
- M. Zhang,
et al., Light and pH Cooperative Nanofluidic Diode Using a Spiropyran-Functionalized Single Nanochannel, Adv. Mater., 2012, 24, 2424–2428 CrossRef CAS PubMed
.
- Z. Zhang,
et al., Asymmetric Multifunctional Heterogeneous Membranes for pH- and Temperature-Cooperative Smart Ion Transport Modulation, Adv. Mater., 2016, 28, 9613–9619 CrossRef CAS PubMed
.
- E. B. Kalman, I. Vlassiouk and Z. S. Siwy, Nanofluidic Bipolar Transistors, Adv. Mater., 2008, 20, 293–297 CrossRef CAS
.
- I. Vlassiouk, T. R. Kozel and Z. S. Siwy, Biosensing with Nanofluidic Diodes, J. Am. Chem. Soc., 2009, 131, 8211–8220 CrossRef CAS PubMed
.
- G. Nguyen, S. Howorka and Z. S. Siwy, DNA Strands Attached Inside Single Conical Nanopores: Ionic Pore Characteristics and Insight into DNA Biophysics, J. Membr. Biol., 2011, 239, 105–113 CrossRef CAS PubMed
.
- M. Ali,
et al., Biosensing and Supramolecular Bioconjugation in Single Conical Polymer Nanochannels. Facile Incorporation of Biorecognition Elements into Nanoconfined Geometries, J. Am. Chem. Soc., 2008, 130, 16351–16357 CrossRef CAS PubMed
.
- M. Tagliazucchi, O. Azzaroni and I. Szleifer, Responsive Polymers End-Tethered in Solid-State Nanochannels: When Nanoconfinement Really Matters, J. Am. Chem. Soc., 2010, 132, 12404–12411 CrossRef CAS
.
- T. Ma,
et al., Combining Light-Gated and pH-Responsive Nanopore Based on PEG-Spiropyran Functionalization, Adv. Mater. Interfaces, 2018, 5, 1701051 CrossRef
.
- N. Giamblanco,
et al., Amyloid Growth, Inhibition, and Real-Time Enzymatic Degradation Revealed with Single Conical Nanopore, Anal. Chem., 2018, 90, 12900–12908 CrossRef CAS PubMed
.
- D. Wang,
et al., Regulating the Transport of DNA through Biofriendly Nanochannels in a Thin Solid Membrane, Sci. Rep., 2014, 4, 3985 CrossRef PubMed
.
- B. Yameen,
et al., Single Conical Nanopores Displaying pH-Tunable Rectifying Characteristics. Manipulating Ionic Transport With Zwitterionic Polymer Brushes, J. Am. Chem. Soc., 2009, 131, 2070–2071 CrossRef CAS PubMed
.
- B. Yameen,
et al., Proton-regulated rectified ionic transport through solid-state conical nanopores modified with phosphate-bearing polymer brushes, Chem. Commun., 2010, 46, 1908–1910 RSC
.
- A. Brunsen,
et al., Proton and Calcium-Gated Ionic Mesochannels: Phosphate-Bearing Polymer Brushes Hosted in Mesoporous Thin Films As Biomimetic Interfacial Architectures, Langmuir, 2012, 28, 3583–3592 CrossRef CAS PubMed
.
- I. W. Leong,
et al., Back-Side Polymer-Coated Solid-State Nanopore Sensors, ACS Omega, 2019, 4, 12561–12566 CrossRef CAS PubMed
.
- Y. M. N. D. Y. Bandara, B. I. Karawdeniya, J. T. Hagan, R. B. Chevalier and J. R. Dwyer, Chemically Functionalizing Controlled Dielectric Breakdown Silicon Nitride Nanopores by Direct Photohydrosilylation, ACS Appl. Mater. Interfaces, 2019, 11, 30411–30420 CrossRef CAS PubMed
.
- Y. Youn,
et al., Selective Detection
of Single-Stranded DNA Molecules Using a Glass Nanocapillary Functionalized with DNA, Anal. Chem., 2016, 88, 688–694 CrossRef CAS PubMed
.
- L. Galla,
et al., Hydrodynamic Slip on DNA Observed by Optical Tweezers-Controlled Translocation Experiments with Solid-State and Lipid-Coated Nanopores, Nano Lett., 2014, 14, 4176–4182 CrossRef CAS PubMed
.
- A. Sischka,
et al., Controlled translocation of DNA through nanopores in carbon nano-, silicon-nitride- and lipid-coated membranes, Analyst, 2015, 140, 4843–4847 RSC
.
- B. M. Venkatesan,
et al., Lipid bilayer coated Al2O3 nanopore sensors: towards a hybrid biological solid-state nanopore, Biomed. Microdevices, 2011, 13, 671–682 CrossRef CAS PubMed
.
- S. Hernández-Ainsa,
et al., Lipid-coated nanocapillaries for DNA sensing, Analyst, 2012, 138, 104–106 RSC
.
- E. C. Yusko,
et al., Single-Particle Characterization of Aβ Oligomers in Solution, ACS Nano, 2012, 6, 5909–5919 CrossRef CAS PubMed
.
- Y. P. Shan,
et al., Surface modification of graphene nanopores for protein translocation, Nanotechnology, 2013, 24, 495102 CrossRef CAS PubMed
.
- S. Schmid, P. Stoemmer, H. Dietz and C. Dekker, High Bandwidth Sensing of Single Protein Dynamics using Nanopores and DNA Origami, Biophys. J., 2019, 116, 341a–342a CrossRef
.
- S. M. George, Atomic Layer Deposition: An Overview, Chem. Rev., 2010, 110, 111–131 CrossRef CAS PubMed
.
- M. J. Hampden-Smith and T. T. Kodas, Chemical vapor deposition of metals: Part 1. An overview of CVD processes, Chem. Vap. Deposition, 1995, 1, 8–23 CrossRef CAS
.
- C.-M. Wang, D.-L. Kong, Q. Chen and J.-M. Xue, Surface engineering of synthetic nanopores by atomic layer deposition and their applications, Front. Mater. Sci., 2013, 7, 335–349 CrossRef
.
- H. W. P. Koops, R. Weiel, D. P. Kern and T. H. Baum, High-resolution electron-beam induced deposition, J. Vac. Sci. Technol., B: Microelectron. Process. Phenom., 1988, 6, 477–481 CrossRef CAS
.
- W. F. van Dorp and C. W. Hagen, A critical literature review of focused electron beam induced deposition, J. Appl. Phys., 2008, 104, 081301 CrossRef
.
- R. Rollings,
et al., The effects of geometry and stability of solid-state nanopores on detecting single DNA molecules, Nanotechnology, 2015, 26, 044001 CrossRef CAS PubMed
.
- G. F. Eriksen and K. Dyrbye, Protective coatings in harsh environments, J. Micromech. Microeng., 1996, 6, 55 CrossRef CAS
.
- M. van den Hout,
et al., Controlling nanopore size, shape and stability, Nanotechnology, 2010, 21, 115304 CrossRef PubMed
.
- M. Vogt and R. Hauptmann, Plasma-deposited passivation layers for moisture and water protection, Surf. Coat. Technol., 1995, 74–75, 676–681 CrossRef CAS
.
- B. Yin,
et al., Covalent Modification of Silicon Nitride Nanopore by Amphoteric Polylysine for Short DNA Detection, ACS Omega, 2017, 2, 7127–7135 CrossRef CAS
.
-
M. J. Rosen and D. J. T. Kunjappu, Surfactants and Interfacial Phenomena, John Wiley & Sons, 2012 Search PubMed
.
-
J. Salager, Surfactants: Types and uses, 2002 Search PubMed
.
- S. Paria and K. C. Khilar, A review on experimental studies of surfactant adsorption at the hydrophilic solid–water interface, Adv. Colloid Interface Sci., 2004, 110, 75–95 CrossRef CAS PubMed
.
- J. Houghtaling, J. List and M. Mayer, Nanopore-Based Rapid Characterization of Individual Amyloid Particles in Solution: Concepts, Challenges, and Prospects, Small, 2018, 14, 1802412 CrossRef PubMed
.
- R. K. Iler, Multilayers of colloidal particles, J. Colloid Interface Sci., 1966, 21, 569–594 CrossRef CAS
.
- G. Decher and J.-D. Hong, Buildup of ultrathin multilayer films by a self-assembly process, 1 consecutive adsorption of anionic and cationic bipolar amphiphiles on charged surfaces, Makromol. Chem., Macromol. Symp., 1991, 46, 321–327 CrossRef CAS
.
- G. Decher and J. D. Hong, Buildup of Ultrathin Multilayer Films by a Self-Assembly Process: II. Consecutive Adsorption of Anionic and Cationic Bipolar Amphiphiles and Polyelectrolytes on Charged Surfaces, Ber. Bunsenges. Phys. Chem., 1991, 95, 1430–1434 CrossRef CAS
.
- G. Decher, J. D. Hong and J. Schmitt, Buildup of ultrathin multilayer films by a self-assembly process: III. Consecutively alternating
adsorption of anionic and cationic polyelectrolytes on charged surfaces, Thin Solid Films, 1992, 210, 831–835 CrossRef
.
- Z. Tang, Y. Wang, P. Podsiadlo and N. A. Kotov, Biomedical Applications of Layer-by-Layer Assembly: From Biomimetics to Tissue Engineering, Adv. Mater., 2006, 18, 3203–3224 CrossRef CAS
.
- J. J. Richardson,
et al., Innovation in Layer-by-Layer Assembly, Chem. Rev., 2016, 116, 14828–14867 CrossRef CAS PubMed
.
- A Silane Primer: Chemistry and Applications of AIkoxy Silanes. ResearchGate Available at: https://www.researchgate.net/publication/237302419_A_Silane_Primer_Chemistry_and_Applications_of_AIkoxy_Silanes. (Accessed: 29th May 2017).
- J. B. Brzoska, I. B. Azouz and F. Rondelez, Silanization of Solid Substrates: A Step Toward Reproducibility, Langmuir, 1994, 10, 4367–4373 CrossRef CAS
.
- D. Kowalczyk, S. Slomkowski, M. M. Chehimi and M. Delamar, Adsorption of aminopropyltriethoxy silane on quartz: an XPS and contact angle measurements study, Int. J. Adhes. Adhes., 1996, 16, 227–232 CrossRef CAS
.
- I. Lee and R. P. Wool, Controlling amine receptor group density on aluminum oxide surfaces by mixed silane self assembly, Thin Solid Films, 2000, 379, 94–100 CrossRef CAS
.
- E. K. U. Larsen,
et al., Size-Dependent Accumulation of PEGylated Silane-Coated Magnetic Iron Oxide Nanoparticles in Murine Tumors, ACS Nano, 2009, 3, 1947–1951 CrossRef CAS PubMed
.
- S. I. Raider, R. Flitsch, J. A. Aboaf and W. A. Pliskin, Surface Oxidation of Silicon Nitride Films, J. Electrochem. Soc., 1976, 123, 560–565 CrossRef CAS
.
- J. C. Love, L. A. Estroff, J. K. Kriebel, R. G. Nuzzo and G. M. Whitesides, Self-Assembled Monolayers of Thiolates on Metals as a Form of Nanotechnology, Chem. Rev., 2005, 105, 1103–1170 CrossRef CAS PubMed
.
- E. Ostuni, R. G. Chapman, R. E. Holmlin, S. Takayama and G. M. Whitesides, A Survey of Structure–Property Relationships of Surfaces that Resist the Adsorption of Protein, Langmuir, 2001, 17, 5605–5620 CrossRef CAS
.
- C. Vericat, M. E. Vela, G. Benitez, P. Carro and R. C. Salvarezza, Self-assembled monolayers of thiols and dithiols on gold: new challenges for a well-known system, Chem. Soc. Rev., 2010, 39, 1805–1834 RSC
.
- A. Ulman, Formation and Structure of Self-Assembled Monolayers, Chem. Rev., 1996, 96, 1533–1554 CrossRef CAS PubMed
.
- G. M. Whitesides, J. K. Kriebel and J. C. Love, Molecular engineering of surfaces using self-assembled monolayers, Sci. Prog., 2005, 88, 17–48 CrossRef
.
- D. Chen,
et al., Low-Potential Detection of Endogenous and Physiological Uric Acid at Uricase–Thionine–Single-Walled Carbon Nanotube Modified Electrodes, Anal. Chem., 2010, 82, 2448–2455 CrossRef CAS PubMed
.
- L. R. Wetter and H. F. Deutsch, Immunological Studies on Egg White Proteins Iv. Immunochemical and Physical Studies of Lysozyme, J. Biol. Chem., 1951, 192, 237–242 CAS
.
- J. Houghtaling,
et al., Estimation of Shape, Volume, and Dipole Moment of Individual Proteins Freely Transiting a Synthetic Nanopore, ACS Nano, 2019, 13, 5231–5242 CrossRef CAS PubMed
.
-
T. Ando, et al., Nanopore formation in the cuticle of an insect olfactory sensillum, 2019, bioRxiv 444729. DOI: DOI:10.1101/444729.
- T. B. H. Schroeder, J. Houghtaling, B. D. Wilts and M. Mayer, It's Not a Bug, It's a Feature: Functional Materials in Insects, Adv. Mater., 2018, 30, e1705322 CrossRef PubMed
.
- M. G. Zagorski,
et al., Amyloid Abeta-the Peptide from Hell, Regul. Pept., 2001, 97, 16 Search PubMed
.
- M. Locke, Permeability of Insect Cuticle to Water and Lipids, Science, 1965, 147, 295–298 CrossRef PubMed
.
- A. Kleefen,
et al., Multiplexed Parallel Single Transport Recordings on Nanopore Arrays, Nano Lett., 2010, 10, 5080–5087 CrossRef CAS
.
- L. J. Steinbock, A. Lucas, O. Otto and U. F. Keyser, Voltage-driven transport of ions and DNA through nanocapillaries, Electrophoresis, 2012, 33, 3480–3487 CrossRef CAS PubMed
.
- Z. Wang,
et al., Nanopipettes: a potential tool for DNA detection, Analyst, 2019, 144, 5037–5047 RSC
.
- L. Xue,
et al., Gated Single-Molecule Transport in Double-Barreled Nanopores, ACS Appl. Mater. Interfaces, 2018, 10, 38621–38629 CrossRef CAS PubMed
.
- A. Abou Chaaya,
et al., Enhanced Ionic Transport Mechanism by Gramicidin A Confined Inside Nanopores Tuned by Atomic Layer Deposition, J. Phys. Chem. C, 2013, 117, 15306–15315 CrossRef CAS
.
- F. Picaud,
et al., Enhanced potassium selectivity in a bioinspired solid nanopore, Phys. Chem. Chem. Phys., 2013, 15, 19601–19607 RSC
.
- S. Balme,
et al., Controlling potassium selectivity and proton blocking in a hybrid biological/solid-state polymer nanoporous membrane, Nanoscale, 2013, 5, 3961–3968 RSC
.
- S. Cabello-Aguilar,
et al., Slow translocation of polynucleotides and their discrimination by α-hemolysin inside a single track-etched nanopore designed by atomic layer deposition, Nanoscale, 2013, 5, 9582–9586 RSC
.
-
S. Balme, M. Lepoitevin, M. Bechelany and J. M. Janot, Hybrid biological/artificial nanopore, in Physics, Chemistry and Applications of Nanostructures, World Scientific, 2015, pp. 454–456. DOI:10.1142/9789814696524_0112
.
- A. R. Hall,
et al., Hybrid pore formation by directed insertion of α-haemolysin into solid-state nanopores, Nat. Nanotechnol., 2010, 5, 874–877 CrossRef CAS PubMed
.
- S. Balme,
et al., New Bioinspired Membrane Made of a Biological Ion Channel Confined into the Cylindrical Nanopore of a Solid-State Polymer, Nano Lett., 2011, 11, 712–716 CrossRef CAS PubMed
.
- R. J. White,
et al., Single Ion-Channel Recordings Using Glass Nanopore Membranes, J. Am. Chem. Soc., 2007, 129, 11766–11775 CrossRef CAS PubMed
.
- D. P. Hoogerheide, B. Lu and J. A. Golovchenko, Pressure–Voltage Trap for DNA near a Solid-State Nanopore, ACS Nano, 2014, 8, 7384–7391 CrossRef CAS PubMed
.
- N. Di Fiori,
et al., Optoelectronic control of surface charge and translocation dynamics in solid-state nanopores, Nat. Nanotechnol., 2013, 8, 946–951 CrossRef CAS PubMed
.
- X. Hou, Y. Hu, A. Grinthal, M. Khan and J. Aizenberg, Liquid-based gating mechanism with tunable multiphase selectivity and antifouling behaviour, Nature, 2015, 519, 70–73 CrossRef CAS PubMed
.
- M. Waugh,
et al., Interfacing solid-state nanopores with gel media to slow DNA translocations, Electrophoresis, 2015, 36, 1759–1767 CrossRef CAS PubMed
.
- M. Davenport, A. Rodriguez, K. J. Shea and Z. S. Siwy, Squeezing Ionic Liquids through Nanopores, Nano Lett., 2009, 9, 2125–2128 CrossRef CAS PubMed
.
- C. M. Frament, N. Bandara and J. R. Dwyer, Nanopore Surface Coating Delivers Nanopore Size and Shape through Conductance-Based Sizing, ACS Appl. Mater. Interfaces, 2013, 5, 9330–9337 CrossRef CAS PubMed
.
- S. H. Petrosko, R. Johnson, H. White and C. A. Mirkin, Nanoreactors: Small Spaces, Big Implications in Chemistry, J. Am. Chem. Soc., 2016, 138, 7443–7445 CrossRef CAS PubMed
.
- S. Balme, M. Lepoitevin, L. F. Dumée, M. Bechelany and J.-M. Janot, Diffusion dynamics of latex nanoparticles coated with ssDNA across a single nanopore, Soft Matter, 2017, 13, 496–502 RSC
.
|
This journal is © The Royal Society of Chemistry 2019 |