DOI:
10.1039/C9NR04301B
(Review Article)
Nanoscale, 2019,
11, 17967-17980
Recent advances in ultra-small fluorescent Au nanoclusters toward oncological research
Received
20th May 2019
, Accepted 15th July 2019
First published on 17th July 2019
Abstract
Au nanoclusters possess a series of excellent properties owing to their size being comparable to the Fermi wavelength of electrons. For example, they show excellent biocompatibility, optical stability, large Stokes shift, intense size-dependent emission and monodispersion, and thus could effectively compensate for the shortcomings of traditional organic fluorescent dyes and fluorescent quantum. In this review, we detail the latest developments of Au nanoclusters employed in the field of biomedicine, especially in oncology research, by summarizing the application of imaging, sensing and drug delivery based on their excellent luminescent properties and unique structural features. We also discuss the significant work relating to Au NCs that now is being devoted in other therapeutic strategies, such as radiotherapy, photothermal therapy and photodynamic therapy, for example. It is anticipated that this review will provide new insights and theoretical guidance to allow the advantages of Au nanoclusters to be realized in oncotherapy.
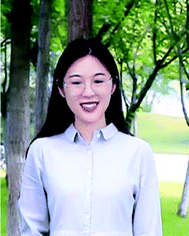 Jingjing Yang | Jingjing Yang is currently pursuing a master's degree within the School of Materials Science and Engineering at Shandong University under the supervision of Professor Yanyan Jiang. She is conducting research on the synthesis of metal nanoparticles and clusters for targeting anti-cancer applications. |
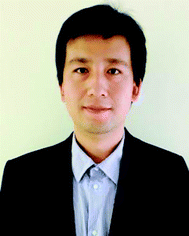 Fenglong Wang | Fenglong Wang gained his PhD in chemical engineering under the supervision of Professor Rose Amal and Dr Yijiao Jiang at the University of New South Wales, Australia. Afterwards, he worked as a specific researcher at Kyoto University within Professor Hiroshi Kitagawa's group from 2016 to 2018. He started his independent career as a professor of materials science at Shandong University in April 2018. His research interests are nanomaterials for solar energy conversion and environmental remediation applications. |
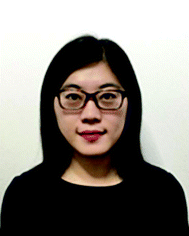 Yanyan Jiang | Yanyan Jiang completed her PhD in Professor Martina Stenzel's group, School of Chemical Engineering at the University of New South Wales, Australia in 2016. She was awarded (2016) Japan Society for Promotion of Science (JSPS) Postdoctoral Research Fellowship at Kyoto University under the supervision of Professor Itaru Hamachi. Since 2018, she has been appointed as a full professor of materials science and engineering at Shandong University. Her research interests are in the synthesis of functional nanoparticles serving as anti-cancer drug carriers, biosensors, catalysts, and theoretical studies of the mechanism and properties of these nanoparticles. |
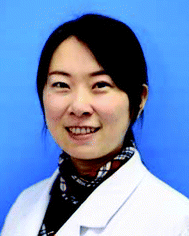 Chao Liu | Chao Liu completed her PhD at Shandong University in 2015 and is now working as a postdoc fellow at Shanghai Jiaotong University. She worked in Ying Xu's laboratory in Georgia University in the US from 2013 to 2015. Her research interests are the mechanism of cancer development, biomarkers of early cancer, and new treatments for cancers. |
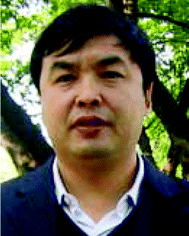 Hui Li | Hui Li received his PhD in 1999 from Shandong University. He then carried out his postdoctoral research in Nanjing University (1999) and University of Trento (2002). He has been promoted to full professor since 2005 in the School of Materials Science and Engineering at Shandong University. He has also been appointed as a Taishan Scholar by Shandong Province since 2013. His research interests are in the areas of wetting transformation of novel 2D materials, synthesis of low expansion alloy and refractory alloys and design of nano-electronic devices. |
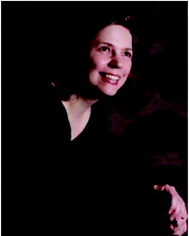 Martina Stenzel | Martina Stenzel studied chemistry at the University of Bayreuth, Germany, before completing her PhD in 1999 at the University of Stuttgart, Germany. She started as a postdoctoral fellow at UNSW in 2000 and is now a full professor. Her research interest is focused on the synthesis of functional polymers with complex architectures such as glycopolymers and other polymers for biomedical applications, especially polymers with in-built metal complexes for the delivery of metal-based anti-cancer drugs. She is a fellow of the Australian Academy of Science and currently the chair of the National Chemistry Committee. |
1 Introduction
The precondition of the quality of human life is vitality and health. The rapid growth of medical nanotechnology promotes the progress of life science and accelerates the development of technologies for human health.1 In most countries, malignant tumors, a heterogeneous pathological condition, are a major cause of human death.2,3 The early detection and accurate diagnosis of cancer before its metastasis are fundamentally important for cancer therapy. At present, for the early stage of non-expandable tumors, surgery is widely adopted as a general means of treatment; for malignant tumors, pre/postoperative radiotherapy and chemotherapy is usually combined with radical operations.4,5 However, these therapies are invasive and painful for patients and are not specific to cancer cells, such that there are side effects that restrict their application.6 Therefore, researchers have been working on early detection and more targeted therapies to achieve diagnosis at an early stage, allowing the elimination of tumors at an earlier level.
In the past few decades, X-ray imaging, magnetic resonance imaging (MRI), ultrasound computer tomography (CT), positron emission tomography (PET), or the combination of PET-CT and other techniques have been used for cancer diagnosis.7,8 In recent years, multifunctional molecular labeling techniques have been applied in the field of oncology, and the progresses of molecular probes has greatly promoted the development of molecular imaging.9 Molecular imaging probe is a technique which can identify and bind to the lesions at the molecular level, produce specific images at the target, obtain molecular information via high-precision imaging techniques, and then trace the path of specific molecules in vivo, to improve diagnostic capacity toward small lesions.10,11 The development of molecular imaging probes provides a new window for the precise diagnosis of diseases and enables the detection of targets to be promoted from the original individuals, organs, tissues into the cell and molecular level, achieving a new breakthrough in in vivo observation.12,13
In order to meet the needs of molecular imaging, nanotechnology has been widely used in molecular probe construction.14,15 The presence of nano-fluorescent probes opens up a new path for targeted detection.16,17 Fluorescent probes with their unique fluorescence lifetime and fluorescence quenching profile can be used as specific markers of biological events, such as monitoring the metabolic activity of the organism in a qualitative and quantitative way.18,19 Traditional fluorescent probes include organic fluorescent dyes,20 quantum dots (QDs)21 and core–shell fluorescent silica nanoparticles.22 However, shortcomings remain in the practical application of these methods; for example, the biological safety and light stability of some of these probes.11 In recent years, Au nanoclusters (Au NCs)23 have drawn significant attention as new fluorescent nanomaterials, as they offset the shortcomings of traditional nanomaterials to a large extent.
Au NCs, generally composed of several to hundreds of Au atoms, are relatively stable molecular-level aggregates.24 Because their size is generally within the low nanometers range, which is comparable to the Fermi wavelength of electrons, the continuous energy states are divided into discrete energies, resulting in so-called quantum effects, such as discrete electronic states and size-dependent fluorescence luminescence.25 Compared with larger nanoparticles, Au NCs possess many attractive features such as a surface effect, small size effect and macroscopic quantum tunneling effect.26 More importantly, unlike Au nanoparticles, Au NCs exhibit no plasma resonance absorption in the visible light region, and their fluorescence maxima lies in the visible to near-infrared region (NIR), making this material more attractive for biological applications.27 In general, Au NCs show great promise as imaging or biosensing agents in biological applications by virtue of their high stability and excellent fluorescence lifetime in vivo.28 Furthermore, the unique size advantages and well-defined surface chemistry offer Au NCs great potential as drug carriers to improve the pharmacodynamical effect of the drug.29
Based on encouraging results of their use in oncology, the current review aims to summarize the main approaches used in the synthesis of Au nanoclusters with unique biochemical and optical properties. Their application in bioimaging, biosensing, drug delivery and other treatments is subsequently highlighted. We also provide perspectives on the future of Au nanoclusters and their entry into clinical trials.
2 Preparation of fluorescent Au nanoclusters
Traditionally, Au NCs are usually prepared using NaBH4 as a reducing agent and polyamidoamine or cetyltrimethylammonium bromide (CTAB) as a stabilizer.30 However, their large specific surface area and high surface free energy makes them prone to agglomeration,27 meaning that gold nanoparticles (Au NPs) are always obtained as a by-product when these methods are employed.27 As such, the focus has been on finding better stabilizers and optimal synthetic procedures to create reliable experimental reaction conditions to prepare Au NCs with precisely controlled sizes.
2.1 Types of stabilizers
Given that ultra-small Au NCs possess a large surface area, various ligands must be attached to its surface as a stabilizing group, forming the idiographic “core–shell” structure to avoid its further agglomeration, which simultaneously furnishes the Au nanoclusters with functional groups with biological activity.31 Since the ligand shell provides copious detection target sites, the diversity of targeted detection is increased, allowing the possibility of application in the field of oncology.32 Furthermore, at the appropriate temperature and pH, ligands, as the electron provider, can further efficaciously affect the properties of luminescence and lifetime Au NCs by influencing the efficiency of electron transfer.33 The types of ligands can be principally divided into thiols, polymers and proteins.
2.1.1 Thiols.
Thiols interact with Au atoms, forming stable semi-covalent Au–S bonds,24 which help stabilize the Au NCs. Commonly used thiol include glycine, glutathione (GSH) and cysteine (Cys).34 Gold–sulfur complex binary systems offer the unique opportunity to prepare Au NCs with different fluorescence emission wavelengths by controlling the chain-length of Au(I)–thiolate complexes on the Au(0) NCs surface and the aggregation of Au(0) atoms.34,35 Moreover, Au–thiolate NCs display aggregation-induced emission (AIE)36 as the continuous aggregation and resulting intermolecular interaction of oligomeric Au(I)–thiolate complexes leads to restriction of the internal motion of the molecule. As a result, the limited movement of the molecule is replaced by light as the energy output, causing a significantly fluorescence-enhanced phenomenon (Fig. 1).36,37
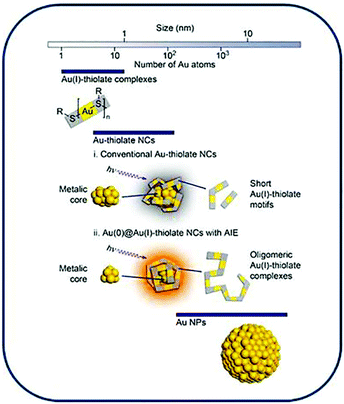 |
| Fig. 1 Schematic illustration of the structures of Au-thiolate NCs and the mechanism of aggregation-induced emission (AIE).36 Adapted from ref. 36. Copyright 2012 American Chemical Society. | |
2.1.2 Polymers.
Polymers, especially dendritic polymers rich in functional groups, can be used as templates to devise Au NCs, improving the sensitivity of the probe and prolonging the fluorescence lifetime.38,39 For example, Fatemi et al. fabricated Au NCs with poly(ethylene glycol)-dithiolane polymers which exhibited high biocompatibility and stability in cells.40 Santiago González et al. utilized poly(N-vinyl-2-pyrrolidone) (PVP) as a template to prepare Au NCs (<1.5 nm) with stable photoluminescent and magnetic properties by electroreduction.41 Interestingly, PVP stabilized Au NCs have also been successfully applied to oxidize benzylic alcohols to the corresponding aldehydes and/or carboxylic acids under ambient temperature in water.39
2.1.3 Proteins.
Proteins have frequently been used as a template when synthesizing high-quality Au nanoclusters, as they offer both high functionality and biodegradability.42 Commonly used proteins include serum albumin, insulin and a variety of small molecule polypeptides.25,43 Once proteins are absorbed onto the Au NCs surface, the fluorescence of the Au NCs is obviously enhanced while the decay of luminescence is retarded, due to the favorable environment provided by the proteins.44 Protein-stabilized Au NCs were first reported in 2009 by Xie et al., who fabricated Au NCs with red photon emission properties using bovine serum albumin (BSA) (Fig. 2a).23 A “green synthesis method”, based on physiological temperatures and mild alkaline conditions, was used create Au NCs with egg white,45 which is a mixture of several proteins (Fig. 2b). Furthermore, it was suggested that the Au NCs form primarily in ovalbumin, a major component of egg white.
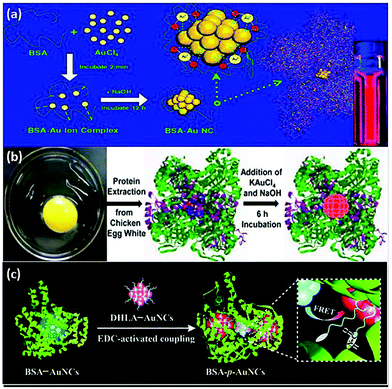 |
| Fig. 2 Schematic illustration of the synthesis of protein-stabilized gold nanoclusters. (a) Schematic of the formation of Au NCs in BSA solution.23 Adapted from ref. 23. Copyright 2009 American Chemical Society. (b) A schematic representation of the experimental procedure for the formation of Au NCs using proteins.45 Adapted from ref. 45. Copyright 2013 Elsevier B.V. (c) Schematic of the synthesis steps of BSA-p-Au NCs.46 Reproduced from ref. 46 with permission from the Royal Society of Chemistry. | |
However, it has been noted that the protein shell is often subject to degradation by reactive oxygen species and enzymes, resulting in fluorescence quenching. This issue can be combated by fabricating ultra-bright BSA-capped Au NCs through efficient fluorescence resonance energy transfer (FRET) between the blue-emitting BSA-Au NCs and the red-emitting thiolated Au NCs (Fig. 2c).46 The as-prepared BSA-p-Au NCs were highly intracellularly stable against proteases due to the formation of covalent bonds between BSA and dithiol ligands of the Au NC precursors, forming polymer-like shielding layers around the Au cores.
2.2 Approaches to the synthesis of fluorescent Au nanoclusters
A prerequisite for the application of Au NCs for medical applications is the design of robust synthesis procedures. The method needs to assist the formation of Au NCs with excellent optical properties, while surface functionality needs to endow the cluster with bioactivity; for example ligands that helps targeting of specific sites.47 Currently, there are many methods for synthesizing Au NCs, including microwave-assisted synthesis,48,49 ultrasonic synthesis,50 phase transfer synthesis,51 kinetic control synthesis,52 and so on. However, some of these methods have shortcomings, such as high cost, low efficiency and the requirement for complex synthetic environments and advanced experimental equipment, which are not suitable for a wide range of applications. Accordingly, we will mainly introduce three common strategies (e.g. chemical reduction, photo reduction and chemical etching) as they yield fluorescent Au NCs with high quantum yield with mild experimental conditions.
2.2.1 Chemical reduction.
Chemical reduction is generally carried out in the presence of reducing agents and capping agents, with HAuCl4 as the substrate substance.27 The purpose of the reducing agent is to reduce Au3+ to Au+ and further to the Au atom in order to form the core of Au NCs.53 Zhang et al. synthesized special water-soluble Au NCs with almost 22.6% quantum yield using multidentate thioether-terminated poly(methacrylic acid) (PTMP-PMAA) as the reducing agent and stabilizer simultaneously.54 As a typical example of the chemical reduction method, Au NCs also can be synthesized in the presence of NaBH4 and dodecanethiolate. The size of the Au cores (∼1.5–5.2 nm) could be precisely controlled via changing the environment of reduction in Hostetler's research. A lower temperature and high thiol-to-Au ratio usually results in smaller cores size and polydispersity; the slower addition rate of the reducing agent increases the odds for particle aggregation during reduction reaction.55
2.2.2 Photo reduction.
The advantage of light is the spatial and temporal control over a reaction.56 Studies have explored whether photo-irradiation could induce the reduction of Au ions free in a polymer matrix to Au atoms acting as the core of nanoclusters.57 For instance, Li et al. utilized three types of multidentate thioether-terminated polymers as ligands, poly(methyl methacrylate) (PTMP-PMMA), poly(n-butyl methacrylate) (PTMP-PBMA) and poly(tert-butyl methacrylate) (PTMP-PtBMA), to efficiently synthesize fluorescent Au NCs (Fig. 3a).58 This approach is not limited to oil-soluble polymers, as Zhang et al. showed.59 They prepared water-soluble fluorescent Au nanoclusters by photo reduction in the presence of poly(methacrylic acid) and pentaerythritol tetrakis 3-mercaptopropionate (PTMP-PMAA), which exhibited excellent photo stability. Formation of Au NCs by photo reduction can be achieved directly on the surface. A two-step process of chemisorption of Au(III) on TiO2 surfaces via ultraviolet (UV) irradiation has been carried out by Soejima et al.60 The proposed ligand and reducing agent-free technique can easily produce Au nanoclusters with a mean size of 3.0 nm.
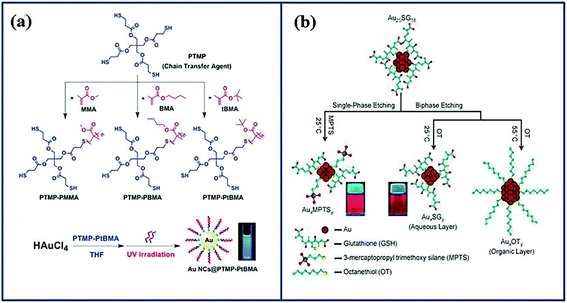 |
| Fig. 3 Schematic illustration of the approaches to synthesis gold nanoclusters. (a) Schematic illustration of the synthesis of three multidentate thioether-terminated ligands PTMP-PMMA, PTMP-PBMA and PTMP-PtBMA, and the preparation of gold nanoclusters under ultraviolet light irradiation.58 Reproduced from ref. 58 with permission from the Royal Society of Chemistry. (b) Formation of the three nanoclusters from Au25SG18 by core etching through two routes.61 Adapted from ref. 61. Copyright 2009 WILEY-VCH. | |
2.2.3 Chemical etching.
Chemical etching is commonly classified as a “top-down” method.62 Generally, the size of the gold nanoparticles (Au NPs) is reduced by ligand-induced etching in the presence of etchants, thereby obtaining small-sized Au NCs.63 Lin et al.64 prepared Au NCs with precursor-induced Au NPs etching. This method is not only robust over a large pH range, but can also generate Au NCs with controllable structure and properties.61–63 Au25SG18, as a more stable Au NCs, has a quantum yield of less than 0.1%. However, Muhammed et al. devised Au25SG18 as the precursor using two different etching methods; single-phase etching and interfacial etching, to controllably prepare Au22, Au23, and Au33 cores with higher quantum yields, as shown in Fig. 3b.61 Furthermore, this research pioneered the preparation of Au23 nanoclusters by etching, and created a unique method of interfacial etching.51
3 Properties of fluorescent Au nanoclusters
3.1 Mechanisms of the optical properties of Au nanoclusters
Compared with Au bulk or Au nanoparticles, the size of Au NCs close to the Fermi level causes the surface plasmon resonance (SPR) to no longer occupy the dominant position.42 Due to electron transition between discrete energy levels, as well as the strong interaction with photons, fluorescent Au NCs effectively compensate for the energy level gap by emitting intense fluorescence.65,66 The principle of illumination follows the laws of quantum mechanics, such that the free electron density and energy spacing determine the efficiency of energy transfer.67 The fluorescence emission wavelength covers the range of visible light to the NIR.68 Due to the size advantage and optical properties, Au NCs can be selectively modified and used to understand intracellular processes.69
In addition to the unique size-dependent optical properties, Au NCs also possess others excellent properties, such as the unique two-photon absorption effect (TPA), excellent biocompatibility, low toxicity, easy surface modification, spectrally coordinated control, good chemical and photostability.70–73 This series of excellent properties paved the way for the wide application of Au NCs, and also opened a new door for their clinical application in oncology.
3.2 Influencing factors on the optical properties of Au nanoclusters
Investigation on fluorescence properties is, of course, conducive to the formation of Au NCs with decent fluorescent intensity, as well as imperative for establishing the application system of Au NCs. Hence, we not only discuss the mechanism of fluorescence phenomena of Au NCs above, but also summarize the influencing factors of the optical performance below. Herein, we divide these factors into intrinsic and external factors.
3.2.1 Intrinsic factors.
The intrinsic factors of fluorescent Au nanoclusters mainly refer to size. The investigation of the relationship between the core number and optical properties is of paramount importance in guiding the further experimental design of the desired Au NCs and analysis of the relevant phenomenon.74,75 Negishi et al. expounded that the optical absorption, photoemission, photoexcitation as well as photoluminescence behavior are largely determined by core number of Aun(SG)m clusters.53 In pepsin-Au NCs systems, Au5 (Au8), Au13 and Au25 correspond with blue-, green-, and red-fluorescent emission respectively.76 Qian et al. proposed that the reason for the presence of different numbers core atoms in nanoclusters systems is based upon the different stability of the polymeric intermediate during the reducing process, indispensably leading to different degrees of atomic aggregation.77
3.2.2 External factors.
The properties of Au NCs can moreover be adjusted by important external factors, including the ligands, the pH value of the solution, the temperature and the quencher.78 For instance, within a certain range, the fluorescence intensity of Au NCs could be increased by increasing the concentration of the template human serum albumin (HSA).44 Since fluorescent probes generally contain acidic or alkaline auxiliary chromophore, the acidity and alkalinity of the solution have a significant effect on their ionization.79,80 Kawasaki et al. utilized the principle that different pH values during the synthesis lead to different Au NCs sizes (Fig. 4) to synthesize pepsin-capped Au NCs with various fluorescence emissions, such as green-, blue- and red-fluorescence.76 Significant effects on the properties were also observed when adjusting the temperature, as the quantum yield and fluorescence intensity of Au NCs generally increases with a decrease in solution temperature.81
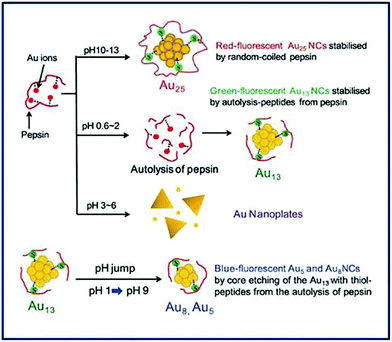 |
| Fig. 4 Schematic illustration of the pH-dependent synthesis of pepsin-mediated Au NCs with blue-, green-, and red-fluorescent emission.76 Adapted from ref. 76. Copyright 2011 WILEY-VCH. | |
4 Applications of fluorescent Au nanoclusters in oncology
The synthesis of Au NCs offers excellent possibilities to create functional nanoparticles, which can be used as imaging/sensing agents and even targeted therapy in the field of oncology.82 With an in-depth understanding of the synthesis–structure relationship of Au NCs, researchers can precisely tune the properties of Au NCs so as to meet the needs for medical applications. In the following sections, the main applications of fluorescent Au NCs in oncological research are summarized.
4.1 Imaging
Based on the unique size effect of Au NCs, the internal electrons can move freely between the discrete energy levels under certain wavelength excitation.67 In other words, the electrons transfer to a higher energy level after absorbing the energy of the excitation light, and thereafter the energy is released in the form of photons when transiting to a stable low energy level.83 The inherent fluorescence of Au NCs makes it naturally suitable for bioimaging and disease diagnosis. As Au NCs were reported to have low cytotoxicity and high biocompatibility, they offer great opportunities for cellular imaging (Table 1). For example, it has been observed that L-carnosine-Au NCs, which emit bright blue fluorescence at high quantum yield and possess a long fluorescence lifetime, could be extensively used in HeLa cell imaging as a fluorescence nanoprobe.84 Moreover, Shang et al. used dihydrolipoic acid (DHLA)-Au NCs to image HeLa cells via fluorescence-lifetime imaging microscopy (FLIM) technology, benefited by the long fluorescence lifetime (>100 ns).85
Table 1 Examples of basic properties of Au NCs for cancer cell imaging
Ligand–Au NCs |
Fluorescent color |
QY |
Fluorescent lifetime/ns |
λ
ex/nm |
λ
em/nm |
Primary imaging cell |
NC, nanocluster; QY, quantum yield. |
L-Carnosine-Au NCs84 |
Blue |
3.4% |
7.48 |
385 |
448 |
HeLa cell |
DHLA-Au NCs85 |
Red |
∼0.6% |
>100 |
684 |
550 |
HeLa cell |
GSH-Au NCs86 |
Green |
_— |
1–3 |
405 |
510 |
MCF7, etc. |
Methionine-Au NCs69 |
Yellow |
1.38% |
∼37.3 |
420 |
530 |
HepG2, etc. |
After the carcinogenesis of normal cells, the intracellular content of GSH increases up to 20–40 times to that of normal cells.87 The in situ formation of gold nanoclusters in cancer cells can be accomplished by the high intracellular GSH.86 As the fluorescence intensity will be significantly higher in cancerous cells owing to the higher GSH concentration, this approach can distinguish healthy cells from cancerous cells. Based on this principle, Chattoraj et al.86 employed the in situ generation of Au NCs capped by intracellular GSH to image cancer cells, including lung cells (A549), breast cells (MCF7), and colon cells (HCT116) (Fig. 5a). In this way, systemic bio-administration of gold precursors, fluorescent Au NCs may be generated in situ for efficacious labeling and target-imaging aiming at precise tumor diagnosis with bright luminescence.88 Upon the reduction of GSH, Au NCs and iron complexes could also be biosynthesized in situ as biocompatibility fluorescent magnetic probes for powerful multimodal tumor bioimaging in vivo.89 Au NCs@GSH is an ideal candidate, allowing for fluorescence/CT dual-mode imaging of gastric cancer with specific biocompatibility and negligible toxicity.90
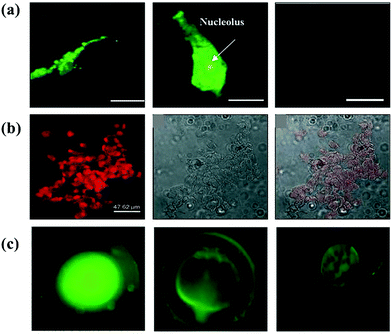 |
| Fig. 5 (a) Confocal images of colon cancer cell stained by in situ generated Au NCs at different concentrations of [pmim] AuCl4: 200 μm [pmim][AuCl4], 600 μm [pmim] [AuCl4] and non-cancer colon cells.86 Adapted from ref. 86. Copyright 2015 WILEY-VCH. (b) Images of human hepatoma (HepG2) cells with streptavidin-conjugated Au23 fluorescence, bright field, and overlay of fluorescent and bright field, respectively.61 Adapted from ref. 61. Copyright 2009 WILEY-VCH. (c) Fluorescent images of zebra-fish embryos at different stage.73 Adapted from ref. 73. Copyright 2016 Elsevier B.V. | |
Further accumulation of Au nanoclusters could also be achieved by surface functionalization to enhance selective binding toward cancerous cells. Li et al.91 used a series of natural proteins, such as egg white, egg yolk, human serum and mouse serum, to prepare Au NCs in order to achieve efficient in vitro cellular uptake by HepG2 cells and better in vivo targeting. Since HepG2 cells contain an excess of biotin, Au23 nanoclusters decorated with streptavidin can strongly bind to biotin in the cells (Fig. 5b).61 Folate receptors (FRs) are overexpressed in most malignant tumor tissues.69 The folate receptor-mediated tumor imaging has received widespread attention. Retnakumari et al. conjugated folic acid (FA) to Au nanoclusters (Au25) to create a versatile fluorescence probe to image carcinoma cells.92 A derivative of the NIR fluorescence dye, Indocyanine Green MPA as a labeling agent, was covalently linked to the FA-Au NCs surface for ameliorating the tumor-targeted imaging and improving affinity to FR-overexpressing tumors.93 Excepting active targeting, fluorescent Au NCs could also target cancer cells by enhancing penetration and retention (EPR) effects, and this phenomenon endows them with the capacity for tumor imaging in vivo.94 Instead of surface modification of existing Au NCs, methionine-coated Au NCs were directly synthesized using a low-cost one-pot strategy. The motivation for using methionine was the specific recognition of the L-type amino acid transporter overexpressed in cancerous cells. As a result, cells (such as A549, HeLa cell, MCF7 and HepG2) in the early stages of cancer can be imaged.69
Au NCs as a class of powerful imaging agents can not only be used in the precise imaging of cancerous cells/tissues, but their application can furthermore be expanded to visualize other living organisms. Chandirasekar et al. generated Au NCs, stabilized and reduced with bio-surfactants (sodium cholate), emitting bluish-green fluorescence as an effective fluorescent probe (Fig. 5c).73 Their subsequent study on life zebra-fish embryos as an in vivo model demonstrated that Au NCs can provide important bioinformatics in bioimaging applications, while displaying high biosafety.
4.2 Sensing
Biosensing, the detection of compounds in a biological setting, has raised substantial interest in oncological fields.12 The key elements of biosensing is analyte-induced fluorescence enhancement or quenching, allowing the quantification of specific markers.21 This can be accomplished using Au NCs, as the fluorescence intensity, fluorescence peak position or related excitation peak positions shift when specific physical interactions and chemical reactions take place between Au NCs and the target molecule. As a consequence, Au NCs can be used to detect overexpressed substances originating from the carcinogenic state of the cell.95 In this section, we will focus on the biorecognition mechanisms of Au NCs, particularly in oncological sensing.
Cystatin C (Cys C) can effectively limit the spread of cancer cells, and it plays an important role in regulating and protecting cells.96 Hence, Lin et al. utilized BSA-Au NCs as a turn-on fluorescent signal to detect Cys C at high sensitivity.97 T Papain, a common cysteine protease, digests BSA to trigger fluorescence quenching of BSA-Au NCs.97 However, the digestion of BSA by papain was inhibited after the addition of Cys C, and consequently fluorescent quenching was absent.97 Thus, the fluorescence intensity could be directly correlated to the presence of Cys C, as shown in Fig. 6a. Citrate is currently considered a preferred biomarker for the early stage detection of prostate cancer. In the presence of H2O2, cysteine-capped Au NCs could work as a visual, sensitive and simple colorimetric system for citrate determination.98
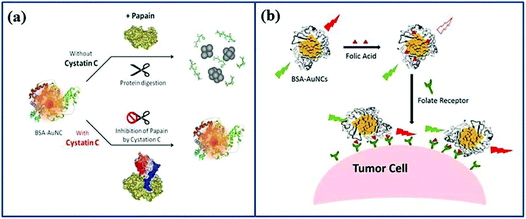 |
| Fig. 6 Schematic illustration of Au NCs as a fluorescent signal to detect substances. (a) Detection of Cys C.97 Adapted from ref. 97. Copyright 2013 Elsevier B.V. (b) Detection of overexpressed FR in cancer cells.103 Adapted from ref. 103. Copyright 2016 Elsevier B.V. | |
A similar approach was used to detect alkaline phosphatase (ALP), another monitoring biomarker, which plays a crucial role in dephosphorylating compounds in biological systems.99 ALP is a promising predictor as its content changes are closely related to a variety of diseases, and the detection of ALP is of practical significance in terms of the sensitivity monitoring the progression of livers metastases.100 Halawa et al.101 found that the interaction between BSA-Au NCs and pyridoxal phosphate (PLP) causes fluorescence quenching, while ALP could restore the fluorescence after hydrolysis of PLP. Hence, BSA-Au NCs as a fluorescent nanoprobe and PLP as a detection substrate were successfully used for the detection of ALP along the range of ∼1–200 U L−1, with a low detection limit of 0.05 U L−1. The disruption of the fluorescence can be utilized to detect other compounds such as endogenous GSH whose excess content is highly correlated with tumor tissues. The on–off–on of the fluorescence of Au NCs@Tf–Cu2+ system made it effectively detect endogenous GSH in tumor cells.102
Cancerous cells contain not only different biomarker levels inside the cell, but they also display an altered surface structure as many receptors (e.g. FRs) are overexpressed.104 Li et al. immobilized FA on BSA-Au NCs as a fluorescent probe to detect over-expression of FR in cancer cells (Fig. 6b).103 FA causes fluorescence quenching of BSA-Au NCs as it changes the environment of BSA.105 However, once the cluster is in contact with FR overexpressing cells, FA is extracted from the BSA shell, and the fluorescence of BSA-Au NCs is restored. This approach can detect FR on cancer cells with excellent sensitivity while minimizing false positive results.106 Human epidermal growth factor receptor 2 (HER2), an overexpressed receptor of HER2+ in breast cancer, could be easily detected by a mesoporous silica–Au NCs hybrid nanoplatform modified with HER2 antibody more aggressively and with greater sensitivity to HER2-positive breast cancer, with the aim of achieving point-of-care cancer diagnostics.107
Diversity affinities and interactions with cell membranes cause fluorescence changes of Au NCs, which act as an effective response mechanism for cell discrimination.108–110 Therefore, Tao et al. demonstrated powerful “chemical nose” array sensors using seven dual-ligand cofunctionalized Au NCs.108 The progress of unbiased cell-recognizers and signal converters achieved unparalleled cell identification, particularly triple negative breast cancers, with excellent biocompatibility, exceptional luminescence, superb biostability and high efficiency.
4.3 Drug delivery
The main challenge of many anti-cancer drugs is their off-target effects which damage healthy cells.111 One way of addressing this issue is by using nano-sized drug delivery vehicles, which are widely studied as a means of targeting tumors with higher specificity.112 Au NCs are usually connected113 or loaded114 anti-cancer drugs based on their special structure, which allows to be Au NCs extensively used as drug delivery systems.93
Cisplatin, a widely used anti-cancer drug, can be generated after reduction of the oxidized cisplatin prodrug (cis,cis,trans-[Pt(NH3)2Cl2(OH) (O2CCH2CH2CO2H)]) (MDDP) with the help of GSH, which is overexpressed in cancer cells.115 Based on the above principle, Zhou et al. modified BSA-Au NCs with MDDP while simultaneously decorating the surface with FA, which enhances entry and targeting of FR positive cells such breast cancer cells and tumors (Fig. 7a).113
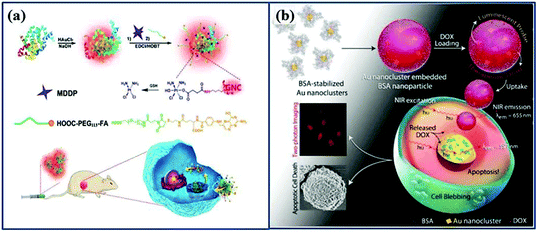 |
| Fig. 7 Schematic illustration of Au NCs as drug delivery systems. (a) Schematic illustration of FA-Au NCs-Pt nanoparticles for tumor-targeted therapy and fluorescence imaging.113 Adapted from ref. 113. Ivyspring International Publisher. (b) Schematic illustration of the formation of DOX-loaded Au nanocluster embedded BSA nanoparticles, leading to the apoptosis of HeLa cell.116 Adapted from ref. 116. Copyright 2015 WILEY-VCH. | |
Au NCs are occasionally not used alone as the actual drug carrier, but the clusters can be processed into larger particles as drug carriers. Chen et al. wrapped Au NCs with a layer of poly(L-lactide), followed by the conjugation of sulfated polysaccharide-folic acid (Au NCs-PLA-GPPS-FA).114 The core–shell nanostructure was able to hold the hydrophobic drug camptothecin (CPT) and to specifically deliver the drug to the FR (+) HeLa cell. Au NCs can also be embedded in albumin nanoparticles as Khandelia et al.116 has shown, which is inspired by the well-known albumin-based nanoformulation Abraxane©.117,118 Similar to the nanoparticle albumin-bound (Nab-) technology, which was used to prepare Abraxane©, Au NCs were processed with doxorubicin (DOX) to create composite nanoparticles that possesses two-photon excitation within the biological window, with the additional advantage of superior spatial resolution, as shown in Fig. 7b. In addition, lysozyme-Au NCs was used to load DOX, creating particles that show high selectivity to breast cancer cells.119
4.4 Other therapeutic strategies
Au nanoclusters, as a promising nanoplatform, are useful for target-imaging, sensing and drug delivery for efficient and accurate conveyance of probes or anti-cancer drugs to lesions.98,120 Such an elegant application of Au NCs in oncology may appear a valuable investigation; it could, however, also be used in other cancer therapeutic strategies, such as radiotherapy (RT), photodynamic therapy (PDT) and photothermal therapy (PTT), for example.121
Radiotherapy is commonly used for topical radiotherapy or to assist surgery.122 Nevertheless, it has been noted that healthy tissues are often subject to unavoidable damage by high-energy radiation (X-ray, γ-ray, and electron beam), potentially resulting in certain serious autoimmune systemic conditions.123,124 Developing ideal radiosensitizers to address the radiation side effects has attracted considerable research attention.125 GSH-coated Au25 NCs inherit attractive features of both the Au core (strong radiosensitizing effect) and GSH shell (good biocompatibility).126 In addition, this nanomaterial is preferentially passive in target-deposition in tumors via the EPR effect, and could also be metabolized efficiently after treatment while minimizing extra toxicity.126,127 Wang et al.128 evaluated the effect of surface charge of Au NCs on radiation therapy, and demonstrated that the negatively charged Au NCs exhibited higher radiotherapy sensitivity and tumor update than positively charged ones.
As a new promising intervention in malignant tumors, PDT generates reactive oxygen species when the photosensitizer is excited, which oxidizes the surrounding macromolecules increases toxicity and induces cell apoptosis.129,130 Xia et al.131 prepared a new Au NC-based nanometric system through a self-assembly approach mediated by hydrophobic and electrostatic effects between fluorescent Au NCs with protamine and hyaluronic acid, which could label specific cells by targeting CD44 and induced cell death by producing singlet oxygen. To accurately destruct specific cancerous tissue, Huang et al. integrated photosensitizer chlorin e6 (Ce6) with silica-coated Au NCs (AuNCs@SiO2) to achieve simultaneous fluorescence imaging-guided PDT, which shows remarkably improved therapeutic effects.132 Yang et al. prepared the nanocomposites by modifying 1D black anatase TiO2 nanotubes with Au NCs, and the obtained materials overcame the two barriers in PDT; e.g., the low reactive oxygen species production capability and the shallow tissue penetration of excited light.133 There are also other valuable novel treatment modalities relevant to Au NCs, such as photothermal therapy and gene therapy.134,135 Au NCs are currently viewed as favorable agents based on either fluorescence-based or vehicle-based formulas as applied to the above treatments.
Although progress in the use of fluorescence Au NCs in advanced treatment technologies has ignited the hopes for improved treatments, we cannot ignore the inherent shortcomings.129 Ideally, effective strategies such as multimodal combination therapy are emerging with the intent to layout an integrated platform for efficient diagnosis and treatment.136,137 For example, chemo-photodynamic therapy138 and chemo-gene therapy139,140 are generally well established in lung or hepatic carcinoma treatment, typically using Au NCs as effective multifunctional delivery vehicles. These multi-model combination therapies are usually accompanied with targeted cellular fluorescence imaging. Generally, high-performance cancer treatment is the culmination of a multifunctional and multi-therapeutic synergy. Further work should be done to realize well-tolerated individualized targeting treatments due to the rare breakthroughs in this aspect.
5 Applications of Au nanoclusters in oncological analysis combined with other substances/particles
Au NCs possess outstanding properties after combination with ligands that can selectively modify or participate in intracellular processes.31 Au NCs can be used alone for cancer diagnosis and treatment, but their combination with other nanosubstances/nanoparticles via surface conjugation enables the design of a powerful entity for imaging, detection and treatment.141
Hyperuricemia, one of the most common clinical manifestations of tumor lysis syndrome (TLS), which is a metabolic disorder occurring during chemotherapy, is a serious threat to the lives of patients.142–144 Thus, effectively monitoring of uric acid in urine samples of patients has practical implications in oncology.145 By coating Au NCs with chondroitin sulfate ((CS)-Au NCs), Liu et al.141 developed a fluorescent nanoprobe ((CS)-Au NCs) whose fluorescence can be quenched by poly(vinylpyrrolidone) (PVP)-Au NPs. The enzyme uricase catalyzes the oxidation of uric acid (UA), which subsequently produces H2O2. This triggers the agglomeration of PVP-coated seeds to (PVP)-Au NPs, which is able to decrease the fluorescence of (CS)-Au NCs (Fig. 8a). This system was able to detect UA in real time and showed higher sensitivity than the fluorescence quenching of Au NCs directly by H2O2.146
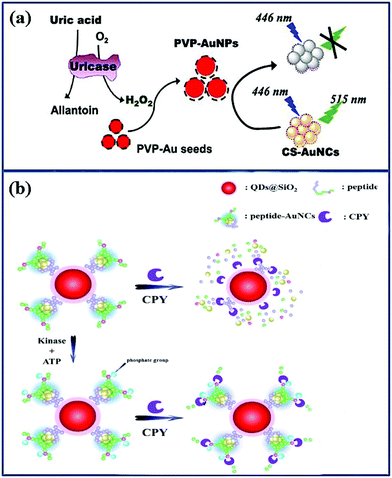 |
| Fig. 8 Schematic illustration of Au NCs combination with other nanosubstances as nanoprobes to detect overexpressing substances in oncology. (a) Schematic illustration of the interaction between PVP-Au NPs and CS-Au NCs to detect UC.141 Adapted from ref. 141. Copyright 2017 Elsevier B.V. (b) Schematic illustration of the CdSe/ZnS QDs@SiO2 Au NCs to detect CPY.72 Adapted from ref. 72. Copyright 2015 Elsevier B.V. | |
The combination of Au NCs and quantum dots (QDs) can be used to synthesize ratio-metric fluorescence sensors to create an efficient protein kinase A (PKA) detection assay.147 PKA catalyzes the transfer of phosphates onto amino acids and is therefore crucial in the activity of proteins. Once the concentration of PKA is unbalanced, diseases such as cancer can occur.148 Song et al. used protein-Au NCs and QDs@SiO2 to form QDs@SiO2@peptide-Au NCs nanohybrids as dual-emission nanoprobes, modified with CdSe/ZnS QDs.72 The as-synthesized QDs@SiO2@peptide-Au NCs nanohybrids were free from environmental interference and reached the lesions accurately, achieving antibody-free fluorescence quantification of carboxypeptidase Y (CPY, active substance of PKA), as shown in Fig. 8b. The research on PKA in cancer detection contributes to find the lesion at the early stage of cancer, achieving the objective of earlier diagnosis and treatment. In addition, Au NCs can also be hybridized with carbon QDs. Xu et al. utilized carbon dots (CDs) to conjugate them to GSH-Au NCs as a ratio-metric nanoprobe for Cys C detection.149
Au NCs could widely interact with other nanoparticles to form complex nanostructures to serve in the treatment of cancer. For example, Chen et al.150 synthesized nanocomposites with nuclear-satellite nanostructures to carry hydrophobic drugs, and subsequently safely deliver the drug to the endosomes or lysosomes of cancer cells. The nanocomposite particles are predominantly based on Au NCs for imaging, FA for targeting, pH-responsive amphoteric polymeric nanocarriers that control the release of the drug and hydrophobic anti-cancer drugs as therapeutics. As a result, the system simultaneously realizes multiple functions, such as imaging, sensing and drug delivery. Sahoo et al.151 implanted Au NCs into chitosan nanoparticles which carried s suicide gene inducing apoptosis of cancer cells. Wang et al. combined BSA-Au NCs and Her-Au NCs into nanoparticles to create a vehicle targeted to breast cancer cells that can simultaneously be imaged.152 Wang et al. prepared Au NCs/reduced graphene oxide (RGO) nanocomposites carrying DOX for oncotherapy.153 Multifunctional Au NCs could also be embedded into polyacylic acid (PAA)@calcium phosphate (CaP) shell as drug delivery vehicles to load the anti-cancer drug DOX with pH-sensitivity releasing properties.154
6 Summary and outlook
In summary, we have systematically described the synthetic methods, properties and the relevant influencing factors of Au NCs, and emphasized the research progress of Au NCs in the field of oncological science. Based on its excellent properties, we have elaborated their applications in detail with regards to bioimaging, biosensing, and drug delivery for efficient cancer treatment therapy. Chemotherapy, however, is not the only method to evade cancer cell proliferation, and significant progress has been made in systems amenable with other treatment, such as radiotherapy, photodynamic therapy, photothermal therapy and multimodal combination therapy. The composites consisting of Au NCs and other nanospecies have also been discussed. Although the research of gold nanoclusters has made promising progress, its further development still faces great challenges. These multimodal approaches aim at systematic treatment with fewer side effects. It is, thus, necessary to integrate the prominent properties of Au NCs for the development of well-tolerated individualized targeting treatments. Extended investigations in combination with other nano-platforms should be also considered for highly efficient tumor therapy with strong biosafety. Until now, although cellular targeted cancer therapy has been fairly high profile, in-depth exploration of Au NC products toward the subcellular-level tumor inhibition is still needed and its therapeutic mechanisms should be investigated. Through functionalization of Au NCs, there is no doubt that Au NCs could become a dispensable tool in cancer clinical trials, affording precise anti-cancer therapy in the near future. There is still space for researchers to explore Au NCs and their derivatives as powerful tools to protect human life and health.
Abbreviations
AIE | Aggregation-induced emission |
ALP | Alkaline phosphatase |
Au NCs | Au nanoclusters/gold nanoclusters |
Au NPs | Au nanoparticles/gold nanoparticles |
BSA | Bovine serum albumin |
CaP | Calcium phosphate |
CDs | Carbon dots |
Ce6 | Chlorin e6 |
CPT | camptothecin |
CPY | Carboxypep-tidase Y |
CS | Chondroitin sulfate |
CT | Computer tomography |
CTAB | Cetyltrimethylammonium bromide |
Cys | Cysteine |
DHLA | Dihydrolipoic acid |
DOX | Doxorubicin |
EPR | Permeation and retention effect |
FA | Folic acid |
FLIM technology | Fluorescence-lifetime imaging microscopy technology |
FRET | Fluorescence resonance energy transfer |
FRs | Folate receptors |
GPPS | Sulfated polysaccharide |
GSH | Glutathione |
Her | Herceptin |
IFE | Inner filter effect |
MDDP |
cis,cis,trans-[Pt(NH3)2Cl2(OH) (O2CCH2CH2CO2H)] |
MRI | Magnetic resonance imaging |
NIR | Near-infrared |
PAA | Polyacylic acid |
PAMAM | Polyamidoamines |
PBMA | Poly(n-butyl methacrylate) |
PDT | Photodynamic therapy |
PEG | Poly(ethylene glycol) |
PEI | Polyethylenimine |
PET | Ultrasound and positron emission tomography |
PKA | Protein kinase A |
PLA | Poly(L-lactide) |
PLP | Pyridoxal phosphate |
PMAA | Poly(methacrylic acid) |
PMMA | Poly(methyl methacrylate) |
PtBMA | Poly(tert-butyl methacrylate) |
PTMP | Pentaerythritol tetrakis 3-mercaptopropionate |
PTT | Photothermal therapy |
PVP | Poly(N-vinyl-2-pyrrolidone) |
QDs | Quantum dots |
RGO | Reduced graphene oxide |
RT | Radiotherapy |
SPR | Surface plasmon resonance |
TA | Thioctic acid |
TLS | Tumor lysis syndrome |
TPA | Two-photon absorption effect |
UA | Uric acid |
UV-irradiation | Ultraviolet-irradiation |
Conflicts of interest
There are no conflicts to declare.
Acknowledgements
The authors would like to acknowledge the support from the National Natural Science Foundation of China (Grant No. 51671114) and the Fundamental Research Funds of Shandong University (Grant No. 2018JC046, 2018JC047). This work is also supported by the Special Funding in the Project of the Taishan Scholar Construction Engineering and Qilu Young Scholar Program of Shandong University.
References
- J. Hadley, Med. Care Res. Rev., 2003, 60, 3S–75S CrossRef PubMed.
- F. Binder-Foucard, N. Bossard, P. Delafosse, A. Belot, A. S. Woronoff and L. Remontet, Rev. Epidemiol. Sante, 2014, 62, 95–108 CrossRef CAS PubMed.
- A. Montazeri, J. Exp. Clin. Cancer Res., 2008, 27, 32 CrossRef PubMed.
- I. J. Fidler and G. Poste, Springer Semin. Immunopathol., 1982, 5, 161–174 CrossRef CAS.
- S. T. Ong and N. J. Vogelzang, J. Clin. Oncol., 1996, 14, 1007–1017 CrossRef CAS PubMed.
- C. H. Chang, J. Horton, D. Schoenfeld, O. Salazer, R. Perez-Tamayo, S. Kramer, A. Weinstein, J. S. Nelson and Y. Tsukada, Cancer, 1983, 52, 997–1007 CrossRef CAS PubMed.
- S. S. Gambhir, Nat. Rev. Cancer, 2002, 2, 683 CrossRef CAS PubMed.
- H. J. Choi, J. W. Roh, S.-S. Seo, S. Lee, J.-Y. Kim, S.-K. Kim, K. W. Kang, J. S. Lee, J. Y. Jeong and S.-Y. Park, Cancer, 2006, 106, 914–922 CrossRef PubMed.
- H. Kobayashi, M. R. Longmire, M. Ogawa and P. L. Choyke, Chem. Soc. Rev., 2011, 40, 4626–4648 RSC.
- Y. Urano, D. Asanuma, Y. Hama, Y. Koyama, T. Barrett, M. Kamiya, T. Nagano, T. Watanabe, A. Hasegawa, P. L. Choyke and H. Kobayashi, Nat. Med., 2008, 15, 104 CrossRef PubMed.
- H. Kobayashi, M. Ogawa, R. Alford, P. L. Choyke and Y. Urano, Chem. Rev., 2010, 110, 2620–2640 CrossRef CAS PubMed.
- K. Pu, A. J. Shuhendler, J. V. Jokerst, J. Mei, S. S. Gambhir, Z. Bao and J. Rao, Nat. Nanotechnol., 2014, 9, 233 CrossRef CAS PubMed.
- K. C. Briley-Saebo, W. J. M. Mulder, V. Mani, F. Hyafil, V. Amirbekian, J. G. S. Aguinaldo, E. A. Fisher and Z. A. Fayad, J. Magn. Reson. Imaging, 2007, 26, 460–479 CrossRef PubMed.
- M. Ferrari, Nat. Rev. Cancer, 2005, 5, 161 CrossRef CAS PubMed.
- F. Alam, M. Naim, M. Aziz and N. Yadav, Indian J. Cancer, 2014, 51, 506–510 CrossRef CAS PubMed.
- S. Wang, N. Li, W. Pan and B. Tang, TrAC, Trends Anal. Chem., 2012, 39, 3–37 CrossRef CAS.
- S. Santra, D. Dutta, G. A. Walter and B. M. Moudgil, Technol. Cancer Res. Treat., 2005, 4, 593–602 CrossRef CAS PubMed.
- J. Zhang, R. E. Campbell, A. Y. Ting and R. Y. Tsien, Nat. Rev. Mol. Cell Biol., 2002, 3, 906 CrossRef CAS PubMed.
- J. Chan, S. C. Dodani and C. J. Chang, Nat. Chem., 2012, 4, 973 CrossRef CAS PubMed.
- M. S. T. Gonçalves, Chem. Rev., 2009, 109, 190–212 CrossRef PubMed.
- X. Gao, Y. Cui, R. M. Levenson, L. W. K. Chung and S. Nie, Nat. Biotechnol., 2004, 22, 969 CrossRef CAS PubMed.
- H. Ow, D. R. Larson, M. Srivastava, B. A. Baird, W. W. Webb and U. Wiesner, Nano Lett., 2005, 5, 113–117 CrossRef CAS PubMed.
- J. Xie, Y. Zheng and J. Y. Ying, J. Am. Chem. Soc., 2009, 131, 888–889 CrossRef CAS PubMed.
- R. Jin, Nanoscale, 2010, 2, 343–362 RSC.
- L. Shang, S. Dong and G. U. Nienhaus, Nano Today, 2011, 6, 401–418 CrossRef CAS.
- D. Chevrier, A. Chatt and P. Zhang, J. Nanophotonics, 2012, 6, 064504 CrossRef.
- Y. Zheng, L. Lai, W. Liu, H. Jiang and X. Wang, Adv. Colloid Interface Sci., 2017, 242, 1–16 CrossRef CAS PubMed.
- J. Xu and L. Shang, Chin. Chem. Lett., 2018, 29, 1436–1444 CrossRef CAS.
- X. Qu, Y. Li, L. Li, Y. Wang, J. Liang and J. Liang, J. Nanomater., 2015, 784097 Search PubMed.
- J. Zhong, J. Qu, F. Ye, C. Wang, L. Meng and J. Yang, J. Colloid Interface Sci., 2011, 361, 59–63 CrossRef CAS PubMed.
- Z. Wu and R. Jin, Nano Lett., 2010, 10, 2568–2573 CrossRef CAS PubMed.
- M. A. Boles, D. Ling, T. Hyeon and D. V. Talapin, Nat. Mater., 2016, 15, 141 CrossRef CAS PubMed.
- K. Z. Milowska and J. K. Stolarczyk, Phys. Chem. Chem. Phys., 2016, 18, 12716–12724 RSC.
- R. R. Nasaruddin, T. Chen, N. Yan and J. Xie, Coord. Chem. Rev., 2018, 368, 60–79 CrossRef CAS.
- Y. Negishi, K. Nobusada and T. Tsukuda, J. Am. Chem. Soc., 2005, 127, 5261–5270 CrossRef CAS PubMed.
- Z. Luo, X. Yuan, Y. Yu, Q. Zhang, D. T. Leong, J. Y. Lee and J. Xie, J. Am. Chem. Soc., 2012, 134, 16662–16670 CrossRef CAS PubMed.
- Y. Hong, J. W. Y. Lam and B. Z. Tang, Chem. Soc. Rev., 2011, 40, 5361–5388 RSC.
- Y. Bao, C. Zhong, D. M. Vu, J. P. Temirov, R. B. Dyer and J. S. Martinez, J. Phys. Chem. C, 2007, 111, 12194–12198 CrossRef CAS.
- H. Tsunoyama, H. Sakurai, Y. Negishi and T. Tsukuda, J. Am. Chem. Soc., 2005, 127, 9374–9375 CrossRef CAS PubMed.
- E. Oh, F. K. Fatemi, M. Currie, J. B. Delehanty, T. Pons, A. Fragola, S. Lévêque-Fort, R. Goswami, K. Susumu, A. L. Huston and I. L. Medintz, Part. Part. Syst. Charact., 2013, 30, 453–466 CrossRef CAS.
- B. Santiago González, M. J. Rodríguez, C. Blanco, J. Rivas, M. A. López-Quintela and J. M. G. Martinho, Nano Lett., 2010, 10, 4217–4221 CrossRef PubMed.
- X. Qu, Y. Li, L. Lei, Y. Wang, J. Liang and J. Liang, J. Nanomater., 2015, 2015, 4 Search PubMed.
- L. Chien-Liang, W. Hung-Tsung, H. Yi-Hsuan, L. Chih-Wei, S. Chun-Wei, P. Yung-Kang, T. Kuo-Chun, C. Hsing-Wei, C. Yun-Chen and H. Jong-Kai, Angew. Chem., Int. Ed., 2011, 50, 7056–7060 CrossRef PubMed.
- L. Shang, S. Brandholt, F. Stockmar, V. Trouillet, M. Bruns and G. U. Nienhaus, Small, 2012, 8, 661–665 CrossRef CAS PubMed.
- D. Joseph and K. E. Geckeler, Colloids Surf., B, 2014, 115, 46–50 CrossRef CAS PubMed.
- W. Zhou, Y. Cao, D. Sui, W. Guan, C. Lu and J. Xie, Nanoscale, 2016, 8, 9614–9620 RSC.
- P. Podsiadlo, G. Krylova, B. Lee, K. Critchley, D. J. Gosztola, D. V. Talapin, P. D. Ashby and E. V. Shevchenko, J. Am. Chem. Soc., 2010, 132, 8953–8960 CrossRef CAS PubMed.
- Y. Yue, T. Y. Liu, H. W. Li, Z. Liu and Y. Wu, Nanoscale, 2012, 4, 2251–2254 RSC.
- L. Yan, Y. Cai, B. Zheng, H. Yuan, Y. Guo, D. Xiao and M. M. F. Choi, J. Mater. Chem., 2012, 22, 1000–1005 RSC.
- H. Liu, X. Zhang, X. Wu, L. Jiang, C. Burda and J.-J. Zhu, Chem. Commun., 2011, 47, 4237–4239 RSC.
- X. Yuan, Z. Luo, Q. Zhang, X. Zhang, Y. Zheng, J. Y. Lee and J. Xie, ACS Nano, 2011, 5, 8800–8808 CrossRef CAS PubMed.
- N. G. Bastús, J. Comenge and V. Puntes, Langmuir, 2011, 27, 11098–11105 CrossRef PubMed.
- Y. Negishi, Y. Takasugi, S. Sato, H. Yao, K. Kimura and T. Tsukuda, J. Am. Chem. Soc., 2004, 126, 6518–6519 CrossRef CAS PubMed.
- J. Zhang, C. Cai, S. Razzaque, I. Hussain, Q.-W. Lu and B. Tan, J. Mater. Chem. B, 2017, 5, 5608–5615 RSC.
- M. J. Hostetler, J. E. Wingate, C.-J. Zhong, J. E. Harris, R. W. Vachet, M. R. Clark, J. D. Londono, S. J. Green, J. J. Stokes, G. D. Wignall, G. L. Glish, M. D. Porter, N. D. Evans and R. W. Murray, Langmuir, 1998, 14, 17–30 CrossRef CAS.
- Q. Sha, B. Sun, C. Yi, R. Guan, J. Fei, Z. Hu, B. Liu and X. Liu, Sens. Actuators, B, 2019, 294, 177–184 CrossRef CAS.
- F. Karadas, G. Ertas, E. Ozkaraoglu and S. Suzer, Langmuir, 2005, 21, 437–442 CrossRef CAS PubMed.
- L. Li, Z. Li, H. Zhang, S. Zhang, I. Majeed and B. Tan, Nanoscale, 2013, 5, 1986–1992 RSC.
- H. Zhang, X. Huang, L. Li, G. Zhang, I. Hussain, Z. Li and B. Tan, Chem. Commun., 2012, 48, 567–569 RSC.
- T. Soejima, H. Tada, T. Kawahara and S. Ito, Langmuir, 2002, 18, 4191–4194 CrossRef CAS.
- M. A. H. Muhammed, P. K. Verma, S. K. Pal, R. C. A. Kumar, S. Paul, R. V. Omkumar and T. Pradeep, Chem. – Eur. J., 2009, 15, 10110–10120 CrossRef CAS PubMed.
- Y. Shichibu, Y. Negishi, H. Tsunoyama, M. Kanehara, T. Teranishi and T. Tsukuda, Small, 2007, 3, 835–839 CrossRef CAS PubMed.
- H. Duan and S. Nie, J. Am. Chem. Soc., 2007, 129, 2412–2413 CrossRef CAS PubMed.
- C.-A. J. Lin, T.-Y. Yang, C.-H. Lee, S. H. Huang, R. A. Sperling, M. Zanella, J. K. Li, J.-L. Shen, H.-H. Wang, H.-I. Yeh, W. J. Parak and W. H. Chang, ACS Nano, 2009, 3, 395–401 CrossRef CAS PubMed.
- S. Chen, S. Wang, J. Zhong, Y. Song, J. Zhang, H. Sheng, Y. Pei and M. Zhu, Angew. Chem., 2015, 127, 3188–3192 CrossRef.
- A. L. West, M. H. Griep, D. P. Cole and S. P. Karna, Anal. Chem., 2014, 86, 7377–7382 CrossRef CAS PubMed.
- G. Schmid and B. Corain, Eur. J. Inorg. Chem., 2003, 2003, 3081–3098 CrossRef.
- R. Philip, P. Chantharasupawong, H. Qian, R. Jin and J. Thomas, Nano Lett., 2012, 12, 4661–4667 CrossRef CAS PubMed.
- Y. Pan, Q. Li, Q. Zhou, W. Zhang, P. Yue, C. Xu, X. Qin, H. Yu and M. Zhu, Talanta, 2018, 188, 259–265 CrossRef CAS PubMed.
- G. Ramakrishna, O. Varnavski, J. Kim, D. Lee and T. Goodson, J. Am. Chem. Soc., 2008, 130, 5032–5033 CrossRef CAS PubMed.
- P. Zhang, X. X. Yang, Y. Wang, N. W. Zhao, Z. H. Xiong and C. Z. Huang, Nanoscale, 2014, 6, 2261–2269 RSC.
- W. Song, R.-P. Liang, Y. Wang, L. Zhang and J.-D. Qiu, Sens. Actuators, B, 2016, 226, 144–150 CrossRef CAS.
- S. Chandirasekar, C. Chandrasekaran, T. Muthukumarasamyvel, G. Sudhandiran and N. Rajendiran, Colloids Surf., B, 2016, 143, 472–480 CrossRef CAS PubMed.
- Q. Tang, G. Hu, V. Fung and D.-e. Jiang, Acc. Chem. Res., 2018, 51, 2793–2802 CrossRef CAS PubMed.
- C. M. Aikens, Acc. Chem. Res., 2018, 51, 3065–3073 CrossRef CAS PubMed.
- H. Kawasaki, K. Hamaguchi, I. Osaka and R. Arakawa, Adv. Funct. Mater., 2011, 21, 3508–3515 CrossRef CAS.
- R. Jin, H. Qian, Z. Wu, Y. Zhu, M. Zhu, A. Mohanty and N. Garg, J. Phys. Chem. Lett., 2010, 1, 2903–2910 CrossRef CAS.
- R. Chib, S. Butler, S. Raut, S. Shah, J. Borejdo, Z. Gryczynski and I. Gryczynski, J. Lumin., 2015, 168, 62–68 CrossRef CAS PubMed.
- T. Peng and D. Yang, Org. Lett., 2010, 12, 496–499 CrossRef CAS PubMed.
- K. Sokołowska, S. Malola, M. Lahtinen, V. Saarnio, P. Permi, K. Koskinen, M. Jalasvuori, H. Häkkinen, L. Lehtovaara and T. Lahtinen, J. Phys. Chem. C, 2019, 123, 2602–2612 CrossRef.
- L. Zhang, M. Zhang and Y. Wu, J. Mol. Struct., 2014, 1069, 245–250 CrossRef CAS.
-
R. Shiji, M. J. Manu, B. S. Unnikrishnan, G. U. Preethi and T. T. Sreelekha, in Advances in Biomaterials for Biomedical Applications, ed. A. Tripathi and J. S. Melo, Springer Singapore, Singapore, 2017, pp. 385–428 Search PubMed.
- M. S. Devadas, J. Kim, E. Sinn, D. Lee, T. Goodson and G. Ramakrishna, J. Phys. Chem. C, 2010, 114, 22417–22423 CrossRef CAS.
- H. Li, J. Chen, H. Huang, J.-J. Feng, A.-J. Wang and L.-X. Shao, Sens. Actuators, B, 2016, 223, 40–44 CrossRef CAS.
- L. Shang, N. Azadfar, F. Stockmar, W. Send, V. Trouillet, M. Bruns, D. Gerthsen and G. U. Nienhaus, Small, 2011, 7, 2614–2620 CrossRef CAS PubMed.
- S. Chattoraj, M. A. Amin, S. Mohapatra, S. Ghosh and K. Bhattacharyya, ChemPhysChem, 2016, 17, 61–68 CrossRef CAS PubMed.
- J. P. Richie, Exp. Gerontol., 1992, 27, 615–626 CrossRef CAS PubMed.
- J. Wang, Y. Jing, J. Hui, S. Gao, G. Wei, C. Yun, C. Liu, C. Amatore and X. Wang, RSC Adv., 2014, 4, 37790 RSC.
- C. Zhao, T. Du, F. U. Rehman, L. Lai, X. Liu, X. Jiang, X. Li, Y. Chen, H. Zhang and Y. Sun, Small, 2016, 12, 6255–6265 CrossRef CAS PubMed.
- C. Zhang, Z. Zhou, Q. Qian, G. Gao, C. Li, L. Feng, Q. Wang and D. Cui, J. Mater. Chem. B, 2013, 1, 5045–5053 RSC.
- L. Li, X. Liu, C. Fu, L. Tan and H. Liu, Opt. Commun., 2015, 355, 567–574 CrossRef CAS.
- A. Retnakumari, S. Setua, D. Menon, P. Ravindran, H. Muhammed, T. Pradeep, S. Nair and M. Koyakutty, Nanotechnology, 2010, 21, 055103 CrossRef PubMed.
- H. Chen, S. Li, B. Li, X. Ren, S. Li, D. M. Mahounga, S. Cui, Y. Gu and S. Achilefu, Nanoscale, 2012, 4, 6050–6064 RSC.
- W. Xu, H. Xiaoxiao, W. Kemin, X. Can, Z. Bing and Q. Zhihe, Nanoscale, 2010, 2, 2244–2249 RSC.
- T.-M. Cheng, H.-L. Chu, Y.-C. Lee, D.-Y. Wang, C.-C. Chang, K.-L. Chung, H.-C. Yen, C.-W. Hsiao, X.-Y. Pan, T.-R. Kuo and C.-C. Chen, Anal. Chem., 2018, 90, 3974–3980 CrossRef CAS PubMed.
- M. Yano, K. Hirai, Z. Naito, M. Yokoyama, T. Ishiwata, Y. Shiraki, M. Inokuchi and G. Asano, Surg. Today, 2001, 31, 385–389 CrossRef CAS PubMed.
- H. Lin, L. Li, C. Lei, X. Xu, Z. Nie, M. Guo, Y. Huang and S. Yao, Biosens. Bioelectron., 2013, 41, 256–261 CrossRef CAS PubMed.
- S. Abarghoei, N. Fakhri, Y. S. Borghei, M. Hosseini and M. R. Ganjali, Spectrochim. Acta, Part A, 2019, 210, 251–259 CrossRef CAS PubMed.
- N. J. Fernandez and B. A. Kidney, Vet. Clin. Pathol., 2007, 36, 223–233 CrossRef PubMed.
- M. W. Saif, D. Alexander and C. M. Wicox, J. Appl. Res., 2005, 5, 88–95 CAS.
- M. I. Halawa, W. Gao, M. Saqib, S. A. Kitte, F. Wu and G. Xu, Biosens. Bioelectron., 2017, 95, 8–14 CrossRef CAS PubMed.
- H. Zhao, X. Wen, W. Li, Y. Li and C. Yin, J. Mater. Chem. B, 2019, 7, 2169–2176 RSC.
- H. Li, Y. Cheng, Y. Liu and B. Chen, Talanta, 2016, 158, 118–124 CrossRef CAS PubMed.
- S. D. Weitman, R. H. Lark, L. R. Coney, D. W. Fort, V. Frasca, V. R. Zurawski and B. A. Kamen, Cancer Res., 1992, 52, 3396 CAS.
- B. Hemmateenejad, F. Shakerizadeh-shirazi and F. Samari, Sens. Actuators, B, 2014, 199, 42–46 CrossRef CAS.
- I. B. Vergote, C. Marth and R. L. Coleman, Cancer Metastasis Rev., 2015, 34, 41–52 CrossRef CAS PubMed.
- M. Li, Y.-H. Lao, R. L. Mintz, Z. Chen, D. Shao, H. Hu, H.-X. Wang, Y. Tao and K. W. Leong, Nanoscale, 2019, 11, 2631–2636 RSC.
- Y. Tao, M. Li and D. T. Auguste, Biomaterials, 2017, 116, 21–33 CrossRef CAS PubMed.
- L. Gong, Y. Chen, K. He and J. Liu, ACS Nano, 2019, 13, 1893–1899 CrossRef CAS PubMed.
- Z. Wu, Y. Du, J. Liu, Q. Yao, T. Chen, Y. Cao, H. Zhang and J. Xie, Angew. Chem., Int. Ed., 2019, 58, 8139–8144 CrossRef CAS PubMed.
- C. D. Geary and J. C. Sun, Semin. Immunol., 2017, 31, 11–19 CrossRef CAS PubMed.
- Y. Liu, H. Miyoshi and M. Nakamura, Int. J. Cancer, 2007, 120, 2527–2537 CrossRef CAS PubMed.
- F. Zhou, B. Feng, H. Yu, D. Wang, T. Wang, J. Liu, Q. Meng, S. Wang, P. Zhang, Z. Zhang and Y. Li, Theranostics, 2016, 6, 679–687 CrossRef CAS PubMed.
- T. Chen, S. Xu, T. Zhao, L. Zhu, D. Wei, Y. Li, H. Zhang and C. Zhao, ACS Appl. Mater. Interfaces, 2012, 4, 5766–5774 CrossRef CAS PubMed.
- H. Xiao, R. Qi, S. Liu, X. Hu, T. Duan, Y. Zheng, Y. Huang and X. Jing, Biomaterials, 2011, 32, 7732–7739 CrossRef CAS PubMed.
- R. Khandelia, S. Bhandari, U. N. Pan, S. S. Ghosh and A. Chattopadhyay, Small, 2015, 11, 4075–4081 CrossRef CAS PubMed.
- M. J. Hawkins, P. Soon-Shiong and N. Desai, Adv. Drug Delivery Rev., 2008, 60, 876–885 CrossRef CAS PubMed.
- Y. Jiang and M. Stenzel, Macromol. Biosci., 2016, 16, 791–802 CrossRef CAS PubMed.
- S. Chattoraj, A. Amin, B. Jana, S. Mohapatra, S. Ghosh and K. Bhattacharyya, ChemPhysChem, 2015, 17, 253–259 CrossRef PubMed.
- R. Binaymotlagh, F. Hajareh Haghighi, F. Aboutalebi, S. Z. Mirahmadi-Zare, H. Hadadzadeh and M.-H. Nasr-Esfahani, New J. Chem., 2019, 43, 238–248 RSC.
- W.-H. Chen, G.-F. Luo and X.-Z. Zhang, Adv. Mater., 2019, 31, 1802725 CrossRef PubMed.
- J.-F. Bosset, L. Collette, G. Calais, L. Mineur, P. Maingon, L. Radosevic-Jelic, A. Daban, E. Bardet, A. Beny and J.-C. Ollier, N. Engl. J. Med., 2006, 355, 1114–1123 CrossRef CAS PubMed.
- D. Lin, E. J. Lehrer, J. Rosenberg, D. M. Trifiletti and N. G. Zaorsky, Radiother. Oncol., 2019, 135, 147–152 CrossRef PubMed.
- Q. Gao, G. Zhou, S.-J. Lin, R. Paus and Z. Yue, Exp. Dermatol., 2019, 28, 413–418 CrossRef PubMed.
- X. D. Zhang, Z. Luo, J. Chen, X. Shen, S. Song, Y. Sun, S. Fan, D. T. Leong and J. Xie, Adv. Mater., 2014, 26, 4565–4568 CrossRef CAS PubMed.
- X. D. Zhang, J. Chen, Z. Luo, D. Wu, X. Shen, S. S. Song, Y. M. Sun, P. X. Liu, J. Zhao and S. Huo, Adv. Healthcare Mater., 2014, 3, 152–152 CrossRef CAS.
- X. Zhang, X. Chen, Y.-W. Jiang, N. Ma, L.-Y. Xia, X. Cheng, H.-R. Jia, P. Liu, N. Gu, Z. Chen and F.-G. Wu, ACS Appl. Mater. Interfaces, 2018, 10, 10601–10606 CrossRef CAS PubMed.
- J. Y. Wang, J. Chen, J. Yang, H. Wang, X. Shen, Y. M. Sun, M. Guo and X. D. Zhang, Int. J. Nanomed., 2016, 11, 3475–3485 CrossRef CAS PubMed.
- D. E. Dolmans, D. Fukumura and R. K. Jain, Nat. Rev. Cancer, 2003, 3, 380–387 CrossRef CAS PubMed.
- B. Mansoori, A. Mohammadi, M. Amin Doustvandi, F. Mohammadnejad, F. Kamari, M. F. Gjerstorff, B. Baradaran and M. R. Hamblin, Photodiagn. Photodyn. Ther., 2019, 26, 395–404 CrossRef CAS PubMed.
- J. Xia, X. Wang, S. Zhu, L. Liu and L. Li, ACS Appl. Mater. Interfaces, 2019, 11, 7369–7378 CrossRef CAS PubMed.
- P. Huang, J. Lin, S. Wang, Z. Zhou, Z. Li, Z. Wang, C. Zhang, X. Yue, G. Niu, M. Yang, D. Cui and X. Chen, Biomaterials, 2013, 34, 4643–4654 CrossRef CAS PubMed.
- D. Yang, A. Gulzar, G. Yang, S. Gai, F. He, Y. Dai, C. Zhong and P. Yang, Small, 2017, 13, 1703007 CrossRef PubMed.
- S. K. Katla, J. Zhang, E. Castro, R. A. Bernal and X. Li, ACS Appl. Mater. Interfaces, 2018, 10, 75–82 CrossRef CAS PubMed.
- S. Yu, R. Wen, H. Wang, Y. Zha, L. Qiu, B. Li, W. Xue and D. Ma, Chem. Mater., 2019, 31, 3992–4007 CrossRef CAS.
- M. Li, Z. Luo, Z. Peng and K. Cai, Wiley Interdiscip. Rev.: Nanomed. Nanobiotechnol., 2019, e1555 Search PubMed.
- S. Gai, G. Yang, P. Yang, F. He, J. Lin, D. Jin and B. Xing, Nano Today, 2018, 19, 146–187 CrossRef CAS.
- F. Xia, W. Hou, C. Zhang, X. Zhi, J. Cheng, J. M. de la Fuente, J. Song and D. Cui, Acta Biomater., 2018, 68, 308–319 CrossRef CAS PubMed.
- S. Huang, Y. Liu, X. Xu, M. Ji, Y. Li, C. Song, S. Duan and Y. Hu, J. Mater. Chem. B, 2018, 6, 2217–2229 RSC.
- C. Chakraborty, A. R. Sharma, G. Sharma, B. K. Sarkar and S.-S. Lee, Oncotarget, 2018, 9, 10164–10174 CrossRef PubMed.
- Y. Liu, H. Li, B. Guo, L. Wei, B. Chen and Y. Zhang, Biosens. Bioelectron., 2017, 91, 734–740 CrossRef CAS PubMed.
- J. Hochberg and M. S. Cairo, Haematologica, 2008, 93, 9 CrossRef CAS PubMed.
- M. S. Cairo and M. Bishop, Br. J. Haematol., 2004, 127, 3–11 CrossRef PubMed.
- K. R. Hande and G. C. Garrow, Am. J. Med., 1993, 94, 133–139 CrossRef CAS PubMed.
- B. Coiffier, A. Altman, C. H. Pui, A. Younes and M. S. Cairo, J. Clin. Oncol., 2008, 26, 2767–2778 CrossRef CAS PubMed.
- J. Wang, Y. Chang, W. B. Wu, P. Zhang, S. Q. Lie and C. Z. Huang, Talanta, 2016, 152, 314–320 CrossRef CAS PubMed.
- X. Liu, N. Zhang, T. Bing and D. Shangguan, Anal. Chem., 2014, 86, 2289–2296 CrossRef CAS PubMed.
- C. Shen, X. Xia, S. Hu, M. Yang and J. Wang, Anal. Chem., 2015, 87, 693–698 CrossRef CAS PubMed.
- X. Yu, C.-X. Zhang, L. Zhang, Y.-R. Xue, H.-W. Li and Y. Wu, Sens. Actuators, B, 2018, 263, 327–335 CrossRef CAS.
- D. Chen, Z. Luo, N. Li, J. Y. Lee, J. Xie and J. Lu, Adv. Funct. Mater., 2013, 23, 4324–4331 CrossRef CAS.
- A. K. Sahoo, S. Banerjee, S. S. Ghosh and A. Chattopadhyay, ACS Appl. Mater. Interfaces, 2014, 6, 712–724 CrossRef CAS PubMed.
- Y. Wang, J. Chen and J. Irudayaraj, ACS Nano, 2011, 5, 9718–9725 CrossRef CAS PubMed.
- C. Wang, J. Li, C. Amatore, Y. Chen, H. Jiangand and X. M. Wang, Angew. Chem., Int. Ed., 2011, 50, 11644–11648 CrossRef CAS PubMed.
- L. Li, L. Zhang, T. Wang, X. Wu, H. Ren, C. Wang and Z. Su, Small, 2015, 11, 3162–3173 CrossRef CAS PubMed.
|
This journal is © The Royal Society of Chemistry 2019 |