DOI:
10.1039/C9NJ02275A
(Paper)
New J. Chem., 2019,
43, 17696-17702
Vanadium(V) complexes of mandelic acid†
Received
3rd May 2019
, Accepted 4th July 2019
First published on 5th July 2019
Abstract
New vanadium complexes of mandelic acid (NMe4)4[V2O4((R)-mand)2][V2O4((S)-mand)2] (1), (NMe4)2[V2O4((S)-mand)2]·H2O (2), (NEt4)2[V2O4((S)-mand)2]·H2O (3), (PPh4)2[V2O4((R)-mand)((S)-mand)]·2H3CCOCH3·2H2O (4), and (NH4)2.5(NEt4)0.5[V3O7((R)-mand)((S)-mand)]·2H2O (5) (mand2− = mandelato ligand) have been synthesized and characterized by single crystal X-ray diffraction and FT-IR spectroscopy. The band assignment in the IR spectra was corroborated by DFT calculations. While the structures of 1–4 comprise the expected dinuclear [V2O4(mand)2]2− (V2L2) anions, the structure of [V3O7((R)-mand)((S)-mand)]3− (V3L2) in 5 is unique and represents a new structural type in vanadium(V) chemistry. Solution studies of vanadate with mandelic acid by 51V NMR revealed the presence of two dominant species V2L2 and V3L2 in aqueous solutions and increasing fraction of V3L2 species at slightly acidic pH (pH ≈ 6).
Introduction
Vanadium participates in an essential way in several biochemical processes. This element was found at the active centre of some enzymes, including vanadium dependent nitrogenases or vanadium haloperoxidases which enable halogenation of organic compounds.1 On the other hand α-hydrocarboxylic acids are also involved in many basic biochemical processes (such as the Krebs cycle, Cori cycle, photorespiration and others).2 Due to the importance of both vanadates and α-hydroxycarboxylic acids the mutual interaction of these groups of compounds was intensively studied.3–551V NMR studies revealed complex equilibria involving several species of different nuclearities; however, solid state investigations confirmed with certainty only dinuclear complexes.6 The dominant components in the vanadate–α-hydroxycarboxylic acid system, apart from common vanadates, are the dinuclear and trinuclear complexes of the composition V2L2 and V3L2 (L = anion of the α-hydroxycarboxylic acid) accompanied by a mononuclear complex VL.7 Several dinuclear vanadium(V) complexes with α-hydroxycarboxylato ligands were also reported in the solid state: (NH4)2[V2O4(glyc)2],8,9 Rb2[V2O4(glyc)2],10 Na2[V2O4(mlact)2]·7H2O,11 (NBu4)2[V2O4(mlact)2]·2H2O,12 (NH4)2[V2O4(ehba)2]·H2O,13 and Cs2[V2O4((S)-lact)2]·2H2O10‡ and a richer series of vanadium complexes with α-hydrocarboxylic acids with more than one carboxyl group such as malic,14 citric,15–23 homocitric24 and tartaric acid.25–28 The crystal structures confirmed the composition and geometry as were proposed by 51V NMR spectroscopy; however, the V3L2 complexes do not have a model compound with a solved crystal structure. In this list, mandelato complexes are obviously missing. Mandelic acid is a common chiral aromatic α-hydroxycarboxylic acid (Scheme 1). Isolation of non-peroxido vanadium(V) complexes of mandelic acid was, so far, not possible mainly due to its strong reduction potential towards VV. In this work we augment the family of vanadium(V) complexes of α-hydroxycarboxylic acids by mandelato complexes. We present the synthesis and characterization of dinuclear V2L2 complexes and a trinuclear V3L2 complex which revealed a surprising structure not identical to the previously proposed geometry.
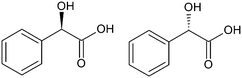 |
| Scheme 1 Stereoisomers of mandelic acid (R)-(−)-H2mand = D-H2mand and (S)-(−)-H2mand = L-H2mand. | |
Experimental
Materials and methods
All chemicals were of analytical grade and used as received. Stock aqueous solutions of (NMe4)VO3 and (NEt4)VO3 (1 mol dm−3) were prepared by dissolution of V2O5 in conc. (NMe4)OH and (NEt4)OH, adjustment of the pH to 8.0 and dilution. Elemental analyses (C, H, and N) were performed on a Vario MIKRO cube (Elementar). The analysis of hydrogen might be influenced by hygroscopic properties of the samples. Vanadium was determined gravimetrically as V2O5. Solid state IR spectra were recorded on a Thermo Scientific Nicolet 6700 FT IR spectrometer in Nujol mulls, KBr pellets and by the ATR technique. The 51V NMR spectra were recorded at 278 K on a Varian Mercury Plus 600 MHz spectrometer operating at 157.88 MHz for 51V in 5 mm tubes. Chemical shifts (δ) are given in ppm relative to VOCl3 as an external standard (δ = 0 ppm).
X-ray diffraction
Diffraction data were collected using a Kappa Apex II (Bruker) diffractometer equipped with a Cryostream Cooler (Oxford Cryosystems) and D8 VENTURE Kappa Duo (Bruker) using detector PHOTON 100 CMOS. Data were collected using graphite-monochromated Mo Kα radiation (λ = 0.71073 Å) and were corrected for radiation absorption and polarization effects by methods incorporated in the diffractometer software. The phase problem was solved by direct methods (SHELXS)29 and refined by full-matrix least-squares based on F2 (SHELXL).30 All non-hydrogen atoms were refined with no restraints and with anisotropic displacement parameters. Hydrogen atoms were included in idealized positions and refined as riding atoms. Graphics were obtained with DIAMOND.31 In the case of compound 5 we were forced to use SQUEEZE to expel cations and molecules of the solvent because of heavy disorder. We therefore present only the structure of the anionic component of the compound. Crystal structure data and refinement details are summarized in Table S1 (see ESI†).
Calculation of infrared spectra
The molecular structure of [V2O4((S)-mand)2]2− from compound 2 has been optimized at the DFT level with the BP86 functional32,33 using tight convergence criteria and an ultrafine integration grid in vacuo. We have employed the all-electron Wachsters+f (14s11p6d3f)/[8s6p4d1f] basis set34,35 for the vanadium atom and the 6-31++G(d,p) basis set36,37 for the remaining atoms. Afterwards, harmonic vibrational frequencies were calculated. Quantum chemical calculations were performed using the Gaussian 09 Revision D.01 program38 and for subsequent potential energy distribution (PED) analysis the VEDA4 program was utilized.39
Syntheses
(NMe4)4[V2O4((R)-mand)2][V2O4((S)-mand)2] (1).
To 9.4 mL of acetone, 0.4 mL of aqueous rac-H2mand (0.5 mol dm−3; 0.2 mmol) and 0.2 mL of NMe4VO3 (1 mol dm−3; 0.2 mmol) were added. The solution was kept at −20 °C. After three weeks, yellow needle-like crystals of 1 were isolated. Yield: 50 mg (40% based on V). Analytical data for C24H36N2O10V2 in % (calc. %): C 46.05 (46.91), H 6.24 (5.91), N 4.58 (4.56), V 16.3 (16.6).
(NMe4)2[V2O4((S)-mand)2]·H2O (2).
To 9.5 mL of acetone, 0.3 mL of aqueous (S)–H2mand (0.5 mol dm−3; 0.15 mmol) and 0.2 mL of NMe4VO3 (1 mol dm−3; 0.2 mmol) were added. The solution was kept at −20 °C. After six weeks, yellow needle-like crystals of 2 were isolated. Yield: 25 mg (20% based on V). Analytical data for C24H38N2O11V2 in % (calc. %): C 45.10 (45.58), H 6.05 (6.06), N 4.59 (4.43).
(NEt4)2[V2O4((S)-mand)2]·H2O (3).
To 100 mL of acetone, 5 mL of NEt4VO3 (1 mol dm−3; 5 mmol) and 5 mL of (S)–H2mand (1 mol dm−3 in EtOH; 5 mmol) were added. The solution was kept at −20 °C. After one day, yellow plate-like crystals of 3 were isolated. Yield: 69 mg (20% based on V). Analytical data for C32H54N2O11V2 in % (calc. %): C 50.49 (51.61), H 7.40 (7.31), N 3.73 (3.76).
(PPh4)2[V2O4((R)-mand)((S)-mand)]·2H3CCOCH3·2H2O (4).
To 9.4 mL of acetone, 0.4 mL of aqueous rac-H2mand (0.5 mol dm−3; 0.2 mmol), 0.2 mL of NMe4VO3 (1 mol dm−3; 0.2 mmol) and 0.225 g of PPh4Cl (0.6 mmol) were added. The solution was kept at −20 °C. After one month yellow needle-like crystals of 4 were isolated. Yield: 44 mg (17% based on V). Analytical data for V2P2C70H68O14 in % (calc. %): C 63.77 (64.82), H 4.92 (5.28), V 8.5 (7.9).
(NH4)2.5(NEt4)0.5[V3O7((R)-mand)((S)-mand)]·2H2O (5).
The pH of an aqueous solution of NEt4VO3 (1.5 mL, c = 1 mol dm−3, 1.5 mmol) was adjusted to 9.0 with concentrated NH3. Afterwards, the solution was mixed with 1.2 mL of aqueous solution of rac-H2mand (1.2 mmol; c = 1 mol dm−3). To the obtained solution acetone (35 mL) was added. The resulting solution was allowed to crystallize at −20 °C. After 3 weeks light yellow crystals in the form of rectangular plates were isolated. Yield: 117 mg (11% based on V). Analytical data for C20H36N3O15V3 in % (calc. %) C 33.35 (33.77), H 6.46 (5.10), N 6.02 (5.91), V 21.89 (21.48).
The (NEt4)3[{VO2(mlact)}2VO2(OH)2] (type V3L2) complex was obtained in the mixture with (NEt4)2[VO2(mlact)2] (type V2L2).7 Attempts to isolate the V3L2 complex in a form suitable for X-ray studies failed. The proposed structure of this complex we discussed in the text.
Results and discussion
Crystal structures
The prepared dinuclear mandelato complexes can be divided into two groups. For compounds 1–3 we observed that the central {V2O4} unit binds always to the mandelato anion of the same configuration. Thus, racemic compound 1 contains both enantiomers [V2O4((S)-mand)2]2− and [V2O4((R)-mand)2]2− related in the crystal packing by the center of symmetry (Fig. 1), while compounds 2 and 3 contain only the [V2O4((S)-mand)2]2− anion. The [V2O4((R)-mand)((S)-mand)]2− anion in 4 contains both enantiomers of the racemic mandelic acid (Fig. 2). Because of the Ci symmetry it is a meso-anion. It can be concluded from the stereochemical point of view that the formation of vanadium(V) mandelato complexes is not stereospecific, because it is possible to obtain the chiral anion (1) and the achiral compound in complex (4) starting from a racemic precursor of rac-mandelic acid. Table 1 summarizes geometrical data for the anions in all compounds. As confirmed by the calculation of τ parameters in pentacoordinated systems, the vanadium atoms V1 and V2 form slightly distorted tetragonal pyramidal coordination geometry and are displaced above the calculated plane by about 0.5 Å. Axial positions are occupied by oxido ligands O1 and O2, respectively. The tetragonal pseudoplane is completed by one oxido ligand (O3, and O4, resp.), and one oxygen atom from the carboxylate anion (O6, and O9, resp.) and the two vanadium atoms are connected by hydroxylate oxygen atoms O5 and O8.
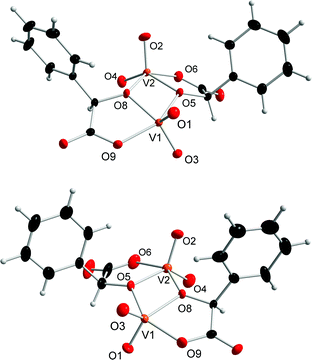 |
| Fig. 1 Molecular structures of anions [V2O4((R)-mand)2]2− (upper, from 1) and [V2O4((S)-mand)2]2− (lower, from 2) showing atom labeling in the central {V2O8} group. Colour code: V orange, O red, C black, and H grey. Displacement ellipsoids are displayed at a 30% probability level. | |
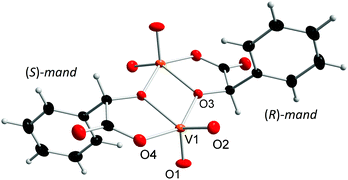 |
| Fig. 2 Molecular structure of the anion [V2O4((R)-mand)((S)-mand)]2− found in compound 4 showing atom labeling in the central {V2O8} group (symmetrically independent part). Colour code: V orange, O red, C black, and H grey. Displacement ellipsoids are displayed at a 30% probability level. | |
Table 1 Selected geometrical parameters of compounds 1–4
|
|
Compound bond lengths and δ in Å |
1
|
2
|
3
|
4* |
Oh – oxygen atom from the original alcoholic group, Oc – oxygen atom from the original carboxylic acid group. τ – parameter calculated from two bond angles in the tetragonal pseudoplane as (α − β)/60; τ = 0 tetragonal pyramidal geometry, τ = 1 trigonal bipyramidal geometry. δ – displacement of the V atom from the ideal plane calculated from the positions of four atoms forming the tetragonal pseudoplane. * – only symmetrically independent bond parameters of the anion are given. |
V O |
V1–O1 |
1.6206(12) |
1.621(4) |
1.622(3) |
1.6213(9) |
|
V2–O2 |
1.6213(13) |
1.613(4) |
1.617(3) |
1.6248(8) |
|
V1–O3 |
1.6305(12) |
1.617(4) |
1.628(3) |
— |
|
V2–O4 |
1.6308(13) |
1.617(4) |
1.636(3) |
— |
|
V–Oh |
V1–O5 |
1.9730(10) |
1.957(3) |
1.954(3) |
V1–O3′ 1.9636(8) |
|
V2–O5 |
2.0438(11) |
2.017(3) |
2.033(3) |
V1–O3 2.0319(8) |
|
V1–O8 |
2.0280(11) |
2.015(3) |
2.032(3) |
1.9637(8) |
|
V2–O8 |
1.9690(11) |
1.969(3) |
1.980(3) |
— |
|
V–Oc |
V1–O9 |
1.9714(11) |
1.960(4) |
1.965(3) |
V1–O4 1.9775(9) |
|
V2–O6 |
1.9711(12) |
1.989(4) |
1.957(3) |
— |
|
τ
V1
|
|
0.2 |
0.38 |
0.11 |
0.15 |
δ
V1
|
|
0.527 |
0.533 |
0.487 |
0.513 |
τ
V2
|
|
0.04 |
0.15 |
0.18 |
— |
δ
V2
|
|
0.565 |
0.555 |
0.533 |
— |
Compound 5 is the first isolated vanadium(V) complex of an α-hydroxycarboxylic with the stoichiometry V3L2. Previous attempts to clarify the structure of such complexes relied mostly on 51V NMR spectroscopy.7 The proposed structure is shown in Scheme 2. However, the X-ray structure analysis of 5 revealed a different structure (Fig. 3). The [V3O7((R)-mand)((S)-mand)2]3− anion contains both enantiomers of rac-mandelic acid. Unlike in Scheme 2, all vanadium atoms are pentacoordinated. Two oxido ligands always occupy the axial positions and one equatorial position. The central vanadium atom V1 is further coordinated by two oxygen atoms coming from the hydroxylate group and the oxido ligand, O1. The oxygen atom, O1, represents an unexpected feature of the anion as it was not expected in V3L2 complexes. The bridging μ3-O1 ligand is connecting all three vanadium atoms of the anion. The coordination of the V2 atom is completed by the chelating mandelato ligand that coordinates through one hydroxylato (O2) and one carboxylate (O3) oxygen atom. Key structural parameters of [V3O7((R)-mand)((S)-mand)]3− are summarized in Table 2.
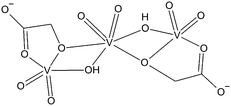 |
| Scheme 2 Structure of V3L2 type complexes as proposed by Tracey et al.7 | |
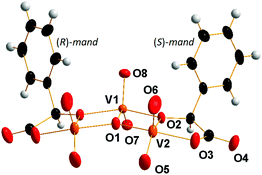 |
| Fig. 3 Molecular structure of the anion [V3O7((R)-mand)((S)-mand)]3− found in 5 showing atom labeling in the central {V3O12} group. Colour code: V orange, O red, C black, and H grey. Displacement ellipsoids are displayed at a 30% probability level. | |
Table 2 Selected geometrical parameters of compound 5
Bond lengths and δ in Å* |
|
|
Oh – oxygen atom from the original alcoholic group, Oc – oxygen atom from the original carboxylic acid group. τ – parameter calculated from the two bond angles in the tetragonal pseudoplane as (α − β)/60; τ = 0 tetragonal pyramidal geometry, τ = 1 trigonal bipyramidal geometry. δ – displacement of the V atom from the ideal plane calculated from the positions of four atoms forming the tetragonal pseudoplane. |
V O |
V2–O5 |
1.6157(17) |
V2–O6 |
1.6407(18) |
V1–O7 |
1.6419(18) |
V1–O8 |
1.6217(19) |
V–Oh |
V1–O2 |
1.9634(12) |
V2–O2 |
2.0183(13) |
V–Oc |
V2–O3 |
1.9775(15) |
V–(μ3-O) |
V2–O1 |
1.9061(7) |
|
V1–O1 |
1.9712(18) |
|
Structural parameters |
|
|
Torsion angle (O8–V1⋯V2–O6) |
23.930° |
τ
V1
|
0.168 |
δ
V1
|
0.462 |
τ
V2
|
0.169 |
δ
V2
|
0.521 |
The triangular center [V3(μ3-O)] has been known in several vanadium(II, III, IV) complexes. However, most of these compounds exhibit D3h symmetry; or at least approximate C3 axis passing through the (μ3-O) atom.40 In the case of anion [V3O7((R)-mand)((S)-mand)]3− the geometry around the O atom is different and it lies in the symmetry plane of the CS symmetry.
In conclusion, the molecular structure of [V3O7((R)-mand)((S)-mand)]3− differs from the proposed one7 in: (1) the absence of bridging OH− groups between V1 and V2 atoms, (2) the presence of a bridging (μ3-O) ligand connecting all vanadium atoms, (3) the coordination number of the central vanadium atom; and (4) the overall symmetry CS.
Infrared spectroscopy
The selected characteristic bands in the IR spectra of the prepared mandelato complexes and their assignment are summarized in Table 3 (the spectra are shown in Fig. S1–S10 (ESI†) and bands of the cations and solvents are tabulated in Table S2, (ESI†)). All compounds exhibit typical strong bands corresponding to ν(V
O) vibrations in the region around 930 cm−1 as well as to ν(C–O)h, ν(C–O)co and ν(C–O)un (for symbols see Table 2) vibrations of the coordinated carboxylato ligand.
Table 3 Selected bands in the IR spectra of the prepared compounds and their assignment
Characteristic group |
Wavenumbers in cm−1 |
1
|
2
|
3
|
4
|
5
|
(C–O)un – uncoordinated (C–O) groups, (C–O)co – coordinated (C–O) groups and (C–O)h – deprotonated alcoholic groups. |
ν(V O) |
921 (s) |
928 (vs) |
935 (s) |
925 (s) |
910 (s) |
|
942 (s) |
|
|
936 (m) |
924 (vs) |
ν(C–O)h |
1048 (m) |
1059 (s) |
1061 (m) |
1063 (m) |
1072 (m) |
|
|
1084 (m) |
|
|
|
ν(C–O)co |
1315 (m) |
1323 (s) |
1304 (m) |
|
1298 (s) |
|
1332 (m) |
|
1329 (m) |
|
1308 (s) |
ν(C–O)un |
1654 (vs) |
1655 (vs) |
1655 (v) |
1651 (s) |
1672 (vs) |
Theoretical calculations are very helpful for more precise band assignment using PED analysis that allows decomposing complicated vibrational movement of the molecule into several components. Thus, we employed this method for band assignment of the model [V2O4((S)-mand)2]2− anion from compound 2. The theoretical values are in good agreement with experimental ones (Table 4) and enable reliable band assignment as shown in Table 3. We recently employed DFT calculations of IR spectra for several chiral and non-chiral vanadium(V) complexes using similar basis sets and methods demonstrating that the interpretation of IR spectra of distinct geometries may be performed reliably using theoretical calculations.41–43
Table 4 Selected infrared bands for [V2O4((S)-mand)2]2−
Calculated wavenumbers with rel. intensity in parentheses |
Experimental wavenumbers |
Band assignment with PED |
Relative intensities are given on the scale of 0–100, the bands with intensities <5 were not included. ar – aromatic circle, un – uncoordinated C O group, co – coordinated C–O group, Cc – carbon atom from the carboxylic acid group, Ch – carbon atom from the original alcoholic group. |
3096 (9) |
2962 m |
ν(C–H)ar 92% |
3080 (11) |
2888 m |
ν(C–H)ar 92% |
1653 (9) |
|
ν(C O)un 83% |
1651 (100) |
1659 vs |
ν(C O)un 83% |
1299 (12) |
1336 m-s |
δ(HCC)ar 64% |
1293 (52) |
1323 s |
ν(C O)co 66% |
1082 (11) |
1084 m |
ν(C–O)h 37% δ(HCC)ar 21% |
1051 (15) |
1059 s |
ν(C–O)h 37% δ(HCC)ar 21% |
968 (17), 964 (10), 953 (16), 951 (21) |
928 vs |
ν(V O) 91% (mean) |
768 (8) |
765 s |
δ(OCO) 26% |
705 (11) |
696 m |
tors(HCCC)ar 36%, tors(CCCC)ar 18% |
675 (5) |
622 w |
tors(HCCC)ar 44%, tors(CCCC)ar 39% |
556 (6) |
537 w |
δ(OChCc)ar 23%, ν(Cc–Ch) 12% |
420 (15) |
444 s |
δ(OChCc)ar 18% |
Solution studies of vanadate with mandelic acid
The 51V NMR spectra have been measured for a number of nonperoxido vanadium(V) α-hydroxycarboxylato complexes.7,13,44–47 The only published data on nonperoxido vanadium(V) complexes with mandelic acid44 supposed the existence of complexes, “a” of the n(V)
:
n(L) = 1
:
1 type with a signal at −532 ppm and, “b” with unknown stoichiometry, the signal for which appeared at −548 ppm. Studied α-hydroxycarboxylic acids can be divided into two groups: The first one consists of the α-hydroxycarboxylic acids containing one carboxylic acid group and one alcoholic group, e.g. glycolic, lactic and mandelic acids. The second group involves α-hydroxycarboxylic acids with more than one carboxyl and hydroxyl groups; such as citric,45 glyceric or malic acids.44 Accordingly, the anions of α-hydroxycarboxylic acids of the second group can act as flexi-dentate ligands and have greater protonation variability in complexes. The most thorough studies7,46 devoted to α-hydroxycarboxylic acids of the first group suggested the existence of two dominant complexes with V2L22− and V3L23− stoichiometries (L represents the α-hydroxycarboxylato(2−) ligand). While the V2L22− complex gives rise to one 51V NMR signal, the trinuclear complex exhibits different peaks for the central and “peripheral” vanadium atoms. Besides the signals of V2L22− and V3L23−, very weak signals of other species (VL−, VL2−, VL3−, and V4L24−) have also been observed. The 51V NMR investigation we performed in the H2VO4−/rac-H2mand/H+ system agrees with the general picture of the composition of complexes obtained by Tracey et al.7,47 and Pettersson et al.46 Summarized data from so far studied systems are given in Table 5.
Table 5
51V NMR chemical shifts of α-hydroxycarboxylato vanadium(V) complexes in aqueous solutions, and ionic strength 1.0 mol dm−3 NaCl. Chemical shifts are given in ppm
|
V2L22− |
V3L22− |
Ref. |
Central V |
Peripheral V |
Ionic strength of 0.15 mol dm−3 NaCl.
Without adjustment of the ionic strength.
|
L-Lactic acid |
−533 |
−550 |
−533 |
7
|
L-Lactic acida |
−533 |
−551 |
−532 |
46
|
α-Hydroxyisobutyric acid |
−549 |
−569 |
−538 |
7
|
2-Ethyl-2-hydroxybutyric acid |
−554 |
−578 |
−538 |
7
|
rac-Mandelic acidb |
−533 |
−551 |
∼−530 |
This work |
In the 51V NMR spectrum of the NMe4VO3–rac-H2mand–H2O system (Fig. 4), it is possible to identify two groups of species. Besides various vanadates (V1: H2VO4−, V2: H2V2O72−, V4: V4O124−, V5: V5O155−, and V10: HxV10O28(6−x)−),48 two broader peaks are present. The signal at −533 ppm corresponds mainly to the V2L22− complex and the signal at −551 ppm arises from the central vanadium atom of the V3L23− complex. The common signal of the two peripheral vanadium atoms of the V3L22− complex is evidently overlapped by the signal at −533 ppm. This overlapped signal is sometimes observed as a downfield shoulder of the −533 ppm signal (Fig. 4). After subtraction of the doubled intensity of the −551 signal from the intensity of the −533 ppm signal, we can estimate the ratio of concentrations of the V2L22− and V3L23− complexes. This ratio decreases from pH = 4.65 (4.1) through pH = 5.37 (2.3), pH = 5.85 (1.4) and pH = 6.23 (1.2). Thus, the V3L23− complex is supported by higher pH values (≈6) in agreement with the literature data (Fig. 5).7,46,47
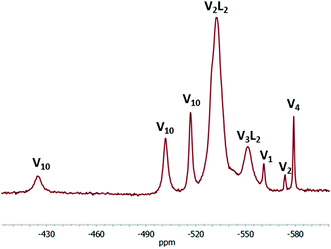 |
| Fig. 4 The 51V NMR spectrum of the NMe4VO3–rac-H2mand–H2O system, c(V) = 0.03 mol dm−3, c[rac-H2mand] = 0.02 mol dm−3, pH = 5.72. Assignment of the chemical shifts: V2L2 −533 ppm, V3L2 −551 ppm, V1 −561 ppm, V2 −574 ppm, V4 −579 ppm, and V10 −517 ppm, −502 ppm and −425 ppm. | |
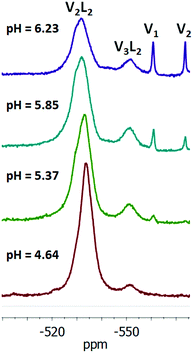 |
| Fig. 5 The 51V NMR spectra of the NMe4VO3–NMe4OH–rac-H2mand–H2O system. c(V) = 0.02 mol dm−3, and c(rac-H2mand) = 0.02 mol dm−3. The assignment of chemical shifts: V2L2 −534 ppm (pH = 4.64), −533 ppm (pH = 5.37), and −532 ppm (pH = 5.85 and 6.23); V3L2 −551 ppm (pH = 5.37); V1 −561 ppm (pH = 5.37, 5.85 and 6.23); and V2 −573 ppm (pH = 5.85 and 6.23). | |
As the complexes were isolated from mixed acetone–H2O solutions, we looked at the speciation also in mixed solvents (Fig. S11, ESI†). The addition of acetone to the aqueous solution results in the broadening of the peaks, raising the value of chemical shift and coalescence of the −551 ppm peak with the −533 ppm peak. On the other hand, the addition of acetone was necessary for the isolation of solid compounds from the solution.
Conclusions
In spite of the fact that the aqueous mandelic acid–vanadate system is prone to redox reactions, we succeeded in the synthesis of new vanadium(V) mandelato complexes by crystallization at −20 °C in acetone–water solvent. Dinuclear chiral [V2O4(mand)2]2− (V2L2) complexes in 1, 2 and 3 contain only one enantiomer of the mandelate anion regardless of whether chiral or racemic mandelic acid was used in the synthesis. On the other hand, the dinuclear achiral [V2O4((R)-mand)((S)-mand)]2− anion in 4 comprises both enantiomers of the mandelato ligand. The [V3O7((R)-mand)((S)-mand)] (V3L2) anion in 5 possesses several unique structural features. To the best of our knowledge, it is the first vanadium(V) trinuclear complex with a triangular {V3-μ3O} centre. The symmetry of the anion is CS and it contains both enantiomers of mandelate. The solution studies confirmed the expected similarity of the mandelic acid–vanadate system in acid aqueous solutions to systems with other α-hydroxycarboxylic acids.
Conflicts of interest
There are no conflicts to declare.
Acknowledgements
This work was supported by the Scientific Grant Agency of the Ministry of Education of Slovak Republic and Slovak Academy of Sciences VEGA project no. 1/0507/17, and by the Slovak Research and Development Agency (APVV-17-0324). LK acknowledges financial support from the Austrian Science Fund (FWF), project no. M2200; and the University of Vienna. RG acknowledges support from Charles University Centre of Advanced Materials (CUCAM) (OP VVV Excellent Research Teams) CZ.02.1.01/0.0/0.0/15_003/0000417.
Notes and references
-
D. Rehder, Bioinorganic vanadium chemistry, Wiley, 2007 Search PubMed.
- P. Schwendt, P. Švančárek, I. Smatanová and J. Marek, J. Inorg. Biochem., 2000, 80, 59 CrossRef CAS.
- E. Bermejo, R. Carballo, A. Castiñeiras and A. B. Lago, Coord. Chem. Rev., 2013, 257, 2639 CrossRef CAS.
- W. T. Jin, W. Z. Wenig and Z. H. Zhou, Eur. J. Inorg. Chem., 2019, 1228 CrossRef CAS.
- P. Schwendt, J. Tatiersky, L. Krivosudský and M. Šimuneková, Coord. Chem. Rev., 2016, 318, 135 CrossRef CAS.
-
A. S. Tracey, G. R. Willsky and E. S. Takeuchi, Vanadium. Chemistry, Biochemistry, Pharmacology and Practical Applications, Taylor & Francis Group, 2007 Search PubMed.
- S. Hati, R. J. Batchelor, F. W. B. Einstein and A. S. Tracey, Inorg. Chem., 2001, 40, 6258 CrossRef CAS.
- Z. H. Zhou, J. Z. Wang, H. L. Wan and K. R. Tsai, Chem. Res. Chin. Univ., 1994, 10, 102 CAS.
- S. Z. Hu, Jiegou Huaxue, 2000, 19, 157 Search PubMed.
- M. Biagioli, L. Strinna-Erre, G. Micera, A. Panzanelli and M. Zema, Inorg. Chim. Acta, 2000, 310, 1 CrossRef CAS.
- S. A. Bourne, J. J. Cruywagen and A. Kleinhorst, Acta Crystallogr., 1999, C55, 2002 CAS.
- I. Smatanová, J. Marek, P. Švančárek and P. Schwendt, Acta Crystallogr., 1998, C54, 1249 Search PubMed.
- T. W. Hambley, R. J. Judd and P. A. Lay, Inorg. Chem., 1992, 31, 343 CrossRef CAS.
- M. Biagioli, L. Strinna-Erre, G. Micera, A. Panzanelli and M. Zema, Inorg. Chim. Acta, 2000, 310, 1 CrossRef CAS.
- C. Gabriel, J. Venetis, M. Kaliva, C. P. Raptopoulou, A. Terzis, C. Drouza, B. Meier, G. Voyiatzis, C. Potamitis and A. Salifoglou, J. Inorg. Biochem., 2009, 103, 503 CrossRef CAS.
- M. Kaliva, C. P. Raptopoulou, A. Terzis and A. Salifoglou, J. Inorg. Biochem., 2003, 93, 161 CrossRef CAS.
- Z.-H. Zhou, H.-L. Wan, S.-Z. Hu and K.-R. Tsai, Inorg. Chim. Acta, 1995, 237, 193 CrossRef CAS.
- M. Tsaramyrsi, D. Kavousanaki, C. P. Raptopoulou, A. Terzis and A. Salifoglou, Inorg. Chim. Acta, 2001, 320, 47 CrossRef CAS.
- Z.-H. Zhou, W.-B. Yan, H.-L. Wan, K.-R. Tsai, J.-Z. Wang and S.-Z. Hu, J. Chem. Crystallogr., 1995, 25, 807 CrossRef CAS.
- D. W. Wright, P. A. Humiston, W. H. Orme-Johnson and W. M. Davis, Inorg. Chem., 1995, 34, 4194 CrossRef CAS.
- Z.-H. Zhou, H. Zhang, Y.-Q. Jiang, D.-H. Lin, H.-L. Wan and K.-R. Tsai, Transition Met. Chem., 1999, 24, 605 CrossRef CAS.
- C.-Y. Chen, Z.-H. Zhou, S.-Y. Mao and H.-L. Wan, J. Coord. Chem., 2007, 60, 1419 CrossRef CAS.
- M. Kaliva, T. Giannadaki, A. Salifoglou, C. P. Raptopoulou and A. Terzis, Inorg. Chem., 2002, 41, 3850 CrossRef CAS.
- D. W. Wright, R. T. Chang, S. K. Mandal, W. H. Armstrong and W. H. Orme-Johnson, J. Biol. Inorg. Chem., 1996, 1, 143 CrossRef CAS.
- J. Gáliková, P. Schwendt, J. Tatiersky, A. S. Tracey and Z. Žák, Inorg. Chem., 2009, 48, 8423 CrossRef.
- P. Schwendt, A. S. Tracey, J. Tatiersky, J. Gáliková and Z. Žák, Inorg. Chem., 2007, 46, 3971 CrossRef CAS.
- P. Antal, P. Schwendt, J. Tatiersky, R. Gyepes and M. Drábik, Transition Met. Chem., 2014, 39, 893 CrossRef CAS.
- J. Cao, Y. Xiong, X. Luo, L. Chen, J. Shi, M. Zhou and Y. Xu, Dalton Trans., 2018, 47, 6054 RSC.
- G. M. Sheldrick, Acta Crystallogr., 2008, A64, 112 CrossRef.
- G. M. Sheldrick, Acta Crystallogr., 2015, C71, 3 CrossRef.
- Diamond - Crystal and Molecular Structure Visualization. Crystal Impact - Dr H. Putz & Dr K. Brandenburg GbR, Kreuzherrenstr. 102, 53227 Bonn, Germany. http://www.crystalimpact.com/diamond.
- A. D. Becke, Phys. Rev., 1988, A38, 3098 Search PubMed.
- J. P. Perdew, Phys. Rev., 1986, B33, 8822 Search PubMed.
- A. J. H. Wachters, J. Chem. Phys., 1970, 52, 1033 CrossRef CAS.
- C. W. Bauschlicher, S. R. Langhoff and L. A. Barnes, J. Chem. Phys., 1989, 91, 2399 CrossRef CAS.
- P. C. Hariharan and J. A. Pople, Theor. Chim. Acta, 1973, 28, 213 CrossRef CAS.
- W. J. Hehre, R. Ditchfield and J. A. Pople, J. Chem. Phys., 1972, 56, 2257 CrossRef CAS.
-
M. J. Frisch, G. W. Trucks, H. B. Schlegel, et al., Gaussian 09, Revision D.01, Gaussian, Inc., Wallingford CT, 2013 Search PubMed.
-
M. H. Jamroz, Vibrational Energy Distribution Analysis VEDA 4, Warsaw, 2004–2010 Search PubMed.
- M. R. Maurya, Coord. Chem. Rev., 2019, 383, 43 CrossRef CAS.
- G. Orešková, J. Chrappová, J. Puškelová, J. Šimunek, P. Schwendt, J. Noga and R. Gyepes, Struct. Chem., 2016, 27, 605 CrossRef.
- L. Krivosudský, P. Schwendt, J. Šimunek and R. Gyepes, J. Inorg. Biochem., 2015, 147, 65 CrossRef PubMed.
- L. Krivosudský, P. Schwendt, R. Gyepes and J. Šimunek, Inorg. Chem. Commun., 2015, 56, 105 CrossRef.
- M. M. Caldeira, M. L. Ramos, N. C. Oliveira and V. M. S. Gil, Can. J. Chem., 1987, 65, 2434 CrossRef CAS.
- A. Gorzsás, K. Getty, I. Andersson and L. Petterson, Dalton Trans., 2004, 2873 RSC.
- A. Gorzsás, I. Andersson and L. Petterson, Dalton Trans., 2003, 2503 RSC.
- A. S. Tracey, Coord. Chem. Rev., 2003, 237, 113 CrossRef CAS.
-
D. C. Crans and A. S. Tracey, The Chemistry of Vanadium in Aqueous and Nonaqueous Solution, ACS Symposium series, 1998, vol. 711, p. 2 Search PubMed.
Footnotes |
† Electronic supplementary information (ESI) available. CCDC 1911693–1911697. For ESI and crystallographic data in CIF or other electronic format see DOI: 10.1039/c9nj02275a |
‡ Abbreviations of ligands: glyc2− – glycolato, lact2− – lactato, mlact2− – methyllactato, ehba2− – 2-ethyl-2-hydroxybutanoato. |
|
This journal is © The Royal Society of Chemistry and the Centre National de la Recherche Scientifique 2019 |