DOI:
10.1039/C9NH00106A
(Communication)
Nanoscale Horiz., 2019,
4, 999-1005
Nonmetallic plasmon induced 500-fold enhancement in the upconversion emission of the UCNPs/WO3−x hybrid†
Received
21st February 2019
, Accepted 26th March 2019
First published on 27th March 2019
Abstract
The nonstoichiometric semiconductor tungsten oxide (WO3−x) is proposed to enhance the upconversion luminescence (UCL) of lanthanide doped upconversion nanoparticles (UCNPs) owing to its unique nonmetallic surface plasmon resonance (SPR) in the near-infrared (NIR) region. The UCNPs/WO3−x hybrid was synthesized on the surface of an optical fiber by a simple coating method. With a waveguiding laser excitation of 980 nm, the hybrid exhibits a drastic enhancement of more than 500-fold at 521 nm emission compared to that of UCNPs, which is even higher than those of some metallic plasmonic structures for UCL enhancement. Such great enhancement is attributed to the strong SPR absorption and thermal effect induced by plasmonic WO3−x, which is demonstrated by the UCL behavior studies of the hybrids by varying the SPR intensity of WO3−x. The UCNPs/WO3−x hybrids were found to exhibit a superior performance in temperature sensing with a wide temperature range (306–1354 K) and a high temperature resolution (0.17–1.18 K).
New concepts
Nonmetallic plasmonic materials with low-cost and abundant reserves have attracted much attention as potential substitutes for plasmonic noble metals. In this study, nonmetallic plasmonic WO3−x was used to substitute plasmonic metals for enhancing the upconversion luminescence (UCL) of upconversion nanoparticles (UCNPs). To our surprise, over 500-fold selective enhancement at the 521 nm emission was achieved by the UCNPs/WO3−x hybrid, which is larger than those of many reported metallic nanostructures. It is demonstrated that such significant selective enhancement can be mainly ascribed to the plasmonic WO3−x induced intense electrical field and thermal effect. This work is expected to pave the way for further development of low-cost nonmetallic plasmonic materials.
|
Lanthanide-doped upconversion nanoparticles (UCNPs) are attractive materials for their ability to convert two or more near-infrared (NIR) photons into one visible emitted photon by the photon upconversion process.1,2 In contrast to organic fluorophores, the lanthanide-doped UCNPs have short emission bands, large anti-Stokes shifts, high stability as well as low biotoxicity.3 Therefore, the UCNPs have important applications in various fields including photoelectric devices,4 biological imaging5 and sensing,6 infrared detection,7 photodynamic therapy8 and solar cells.9 Unfortunately, the low upconversion luminescence (UCL) efficiency of UCNPs hinders their widespread application. Among various methods, plasmonic enhancement is an effective strategy to improve the UCL efficiency of UCNPs.10 Many metallic plasmonic nanostructures including Au (nanorods,11,12 nanohole arrays,13 films14), Al nanodisk arrays,15 and Ag nanogratings16 were coupled with UCNPs to enhance the UCL by one or two orders of magnitude via the surface plasmon resonance (SPR) effect. Such plasmon coupling requires the SPR bands of metallic nanostructures to precisely match the excitation or emission bands of UCNPs.16,17 It is demonstrated that the SPR bands of metallic nanostructures are highly sensitive to their sizes, shapes, and compositions, and need to be accurately controlled. To effectively modulate the SPR bands and reinforce the plasmonic effect, photonic crystals18 and metal–insulator–metal nanopatterns19,20 were proposed to achieve a three-fold enhancement of the UCL. However, these well-designed architectures generally require a complex and time-consuming fabrication process. Moreover, plasmonic metals have a high cost and earth rarity,21 both of which limit the practical applications of plasmonic metals in UCL enhancement. Recently, nonmetallic nanostructures of nonstoichiometric semiconductors with heavy doping have been found to exhibit SPR arising from the high density of free carriers.22–26 Among them, readily synthesized tungsten oxides (WO3−x) have abundant oxygen vacancies, leading to a high intensity of free electrons and strong SPR in the visible-NIR region. Thus, WO3−x has become a potential substitute for plasmonic metals and has been applied in various research areas including light harvesting,27 phototherapy28 and catalysis.29 Considering that its intense SPR band covers a wide NIR region, plasmonic WO3−x is a potential material to replace plasmonic metals for the enhancement of the UCL of UCNPs, and there are few reports of such materials in the literature. Thus, it is necessary to carry out studies of nonmetallic plasmonic enhancement of UCL.
In this work, the UCNPs/WO3−x hybrid was prepared on the surface of an optical fiber, which was introduced to achieve high efficiency UCL via a waveguiding excitation method.30 Compared to the UCNPs, a selective UCL enhancement of more than 500-fold on 521 nm emission was observed for the UCNPs/WO3−x hybrid. Several hybrids of UCNPs and WO3−x with different SPR intensities were prepared, and their UCL study verified that the selective UCL enhancement of UCNPs/WO3−x is mainly attributed to SPR absorption and thermal effect of plasmonic WO3−x. Compared to some reported work, plasmonic WO3−x exhibit higher efficiency than many plasmonic metals in the UCL enhancement. The intensity ratio of 521 to 540 nm emissions of the hybrids is found to be related to the local temperature. Thus, the prepared UCNPs/WO3−x hybrids exhibited a superior performance in temperature sensing (with a range of 306–1354 K and a resolution of 0.17–1.18 K). This work shows that nonmetallic plasmonic materials may replace plasmonic metals in some applications.
Fig. 1a shows the TEM image of Yb3+ and Er3+ co-doped NaYF4 UCNPs with an average size of 40 nm (Fig. S1, ESI†). A protective silica layer with a thickness of ∼5 nm covers UCNPs, which can enhance the stability and also act as an isolation layer to suppress the energy transfer between UCNPs and WO3−x.11,31 The normalized UCL spectrum (Fig. S2, ESI†) of UCNPs shows three characteristic emission peaks at 521, 540, and 654 nm, corresponding to the transitions of 2H11/2 → 4I15/2, 4S3/2 → 4I15/2 and 4F9/2 → 4I15/2, respectively. The plasmonic WO3−x was prepared by a simple solvothermal method used in our previous work.32 The synthesized WO3−x has a bundle-like nanorod structure with a diameter of 10–30 nm and a length of 100–600 nm. (Fig. 1b and Fig. S3a, ESI†) In the high-resolution TEM (HRTEM) image (inset of Fig. S3a, ESI†), the lattice fringes with a spacing of 0.38 nm that corresponds to the (010) planes of W18O49 (JCPDS No. 05-0392) can be observed.33 The elemental composition and chemical states of the as-prepared tungsten oxides were investigated by X-ray photoelectron spectroscopy (XPS). The XPS spectrum of W 4f (Fig. 1c) can be deconvoluted into two pairs of peaks. The two peaks located at 38.2 and 36.1 eV (green lines) correspond to W6+ 4f5/2 and 4f7/2, respectively, while the peaks around 36.8 and 34.7 eV (pink lines) can be attributed to W5+ 4f5/2 and 4f7/2, respectively.33,34 The amount of W5+ is calculated to be 26% of the total W states, indicating that abundant oxygen vacancies exist in the crystal structure of the prepared WO3−x. The oxygen vacancies increase the density of free electrons in WO3−x, which would generate oscillations with the incident light leading to SPR.33 As shown in the UV-vis spectrum (Fig. 1d), WO3−x has a strong SPR absorption in the visible and near-infrared (NIR) light region. Most interestingly, WO3−x exhibits a strong absorption at 980 nm excitation wavelength (∼6.5) but a slight absorption in the major region of the UCNPs emission (500–600 nm), which is greatly beneficial for UCL enhancement. To enhance the utilization of the incident light, an optical fiber was introduced to achieve efficient UCL excitation via a simple waveguiding excitation method. The UCNPs/WO3−x hybrid was coated on the optical fiber by simple coating (see details in Experimental section). Fig. 1e shows a SEM image of the UCNPs/WO3−x coated optical fiber with a diameter of 9.5 μm and UCNPs/WO3−x hybrids are dispersed on its surface. The contacts of UCNPs and WO3−x are observed in the TEM image shown in Fig. 1f. The X-ray diffraction (XRD) pattern of UCNPs/WO3−x (Fig. S3b, ESI†) shows both characteristic peaks of UCNPs35 and WO3−x,33 verifying the hybrid structure of UCNPs/WO3−x.
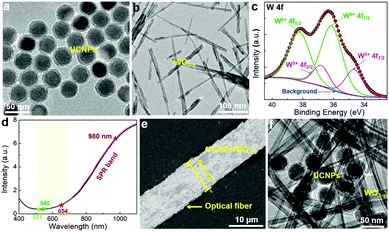 |
| Fig. 1 A typical TEM image of the UCNPs (a), TEM image of WO3−x (b), XPS spectrum of W 4f (c) and UV-vis spectrum of plasmonic WO3−x (d). SEM image (e) of the UCNPs/WO3−x coated optical fiber with a diameter of 9.5 μm. TEM image (f) of the UCNPs/WO3−x hybrids. | |
A schematic diagram of the UCNPs/WO3−x coated optical fiber is shown in Fig. 2a. The continuous wave (CW) laser with a wavelength of 980 nm is coupled with the optical fiber and the SPR of WO3−x is excited leading to a significant electric field enhancement, which plays a great role in the UCL of UCNPs. The UCL emission image and spectra of the samples were measured to investigate the effect of plasmonic WO3−x on the UCL of UCNPs. As reference, UCNPs were coated on the fiber using the same method and their UCL was investigated under the same experiment parameters. Fig. 2b and c are the UCL images of the UCNPs/WO3−x hybrid and UCNPs, respectively, with an incident laser power of 120 mW, and the detailed power density on the surface of the optical fiber was estimated to be ∼55 W cm−2 (see ESI†). To our surprise, the emission of the UCNPs/WO3−x hybrid is much brighter than that of UCNPs, which is further confirmed by the measured UCL spectra. From Fig. 2d, the UCL peak intensities of the UCNPs/WO3−x hybrid exhibit a remarkable enhancement, especially for the 521 nm green emission. The enhancement factors (EFs) for the emissions at 521, 540 and 654 nm were calculated to be 540.1, 27.9 and 1.2, respectively (Fig. S4, ESI†), leading to much higher intensity ratios of 521/540 nm and 521/654 nm. The Commission Internationale de l’Eclairage (CIE) chromaticity coordinates were calculated to be (0.41, 0.53) and (0.20, 0.73) for UCNPs and UCNPs/WO3−x, respectively, as plotted in the CIE diagram (Fig. 2e), clearly showing the emission color variation from yellow to green. In addition, the UCNPs/WO3−x hybrid was demonstrated to possess good photostability as shown in Fig. S5 (ESI†). Consequently, the significant selective UCL enhancement of UCNPs/WO3−x is much higher than those obtained by plasmonic metal structures.11–16 Such UCL enhancement of the UCNPs/WO3−x hybrid is attributed to the SPR effect of WO3−x activated via a waveguiding excitation. The SPR-induced UCL enhancement is related to two phenomena, one is the coupling of SPR with the emission band to accelerate the radiative transition rate, and the other one is the coupling of SPR with the excitation light to enhance the electrical field.36 To understand the detailed mechanism of the WO3−x SPR effect on the UCL, the UCL decay curves of UCNPs and the UCNPs/WO3−x hybrid at three characteristic emission peaks were measured (Fig. S6, ESI†) and the corresponding decay times τ were calculated as shown in Table S1 of ESI.† Compared to UCNPs, the decay times of UCNPs/WO3−x for the 521 and 540 nm emissions were nearly unchanged, but that for the 654 nm emission exhibits a decrease of 0.07 ms. This demonstrates that the UCL enhancement of UCNPs/WO3−x is mainly ascribed to the WO3−x SPR-induced electrical field enhancement at a 980 nm excitation.13 Since the 654 nm emission of UCNPs is overlapped with the SPR absorption band of WO3−x, it can be absorbed by the adjacent WO3−x, leading to a higher intensity ratio of 521/654 nm and a decrease in the decay time.
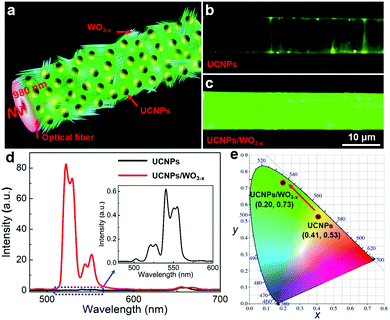 |
| Fig. 2 Schematic diagram (a) of the UCNPs/WO3−x coated optical fiber. UCL emission images of UCNPs/WO3−x (b) and UCNPs (c) at a laser power of 120 mW. Corresponding UCL spectra (d) of UCNPs/WO3−x (red) and UCNPs (black), the inset is the UCL spectra of UCNPs with a small intensity scale. CIE diagram (e) with plotted chromaticity coordinates for UCNPs and UCNPs/WO3−x. | |
The dependence of the UCL intensity on the laser power was investigated to study the UCL emission behaviours of the UCNPs/WO3−x hybrid. Fig. 3a and Fig. S7a (ESI†) show the UCL spectra of the UCNPs/WO3−x hybrid and UCNPs under a 980 nm laser excitation with varying powers from 50 to 176 mW. As the pump power increases, all the intensities of the emission peaks were enhanced for the UCNPs/WO3−x hybrid and UCNPs. The emission intensity of UCNPs is known to be proportional to the nth power of the laser power P,37,38 described as
Here,
n is the number of photons involved in the UCL process, which is generally larger than or equal to 2 due to the multiphoton absorption in UCNPs. A plot of log(
I)
versus log(
P) for the three peak emissions of the UCNPs/WO
3−x hybrid and UCNPs are shown in
Fig. 3b and Fig. S7b (ESI
†), respectively. For UCNPs, the calculated
n values for 521, 540 and 654 nm are 1.57, 1.47 and 1.69, respectively, which are smaller than 2 and this can be attributed to the energy loss during the UCL process.
14 For UCNPs/WO
3−x, the power dependence of the UCL emissions can be divided into two periods. In the low laser power regime, the
n values are 4.32, 3.05 and 2.74 for the 521, 540 and 654 nm emissions, respectively. Such obvious increase in the
n value means an improvement in the utilization efficiency of the incident light, which is consistent with the observed emission enhancement of UCNPs/WO
3−x. However, at high laser powers, the
n values of the UCNPs/WO
3−x hybrid drastically decreased to 1.13, 0.34 and 0.64 for 521, 540 and 654 nm emissions, respectively. This is mainly attributed to the intermediate energy state saturation and the local thermal effect induced by the SPR of plasmonic WO
3−x.
37 The saturation of the excitation state coupled with the upconversion at a low laser power indicates that plasmonic WO
3−x successfully induce a strong electrical field under 980 nm excitation. The absorption of the excitation light and the subsequent photothermal conversion of plasmonic WO
3−x lead to a high local temperature. In addition, in contrast to UCNPs, all the emission intensities of the UCNPs/WO
3−x hybrid exhibit a remarkable enhancement but with different EFs (
Fig. 3c). The EFs exhibit a similar trend of an initial increase and then decrease for all three emissions as the laser power increases, which can be attributed to the saturation effect at high laser powers.
Fig. 3c also shows that EFs are highly dependent on the emission peak positions, and a maximum enhancement of more than 500 fold is observed at a 521 nm emission, while only ∼40 and 3 fold enhancement can be achieved at 540 and 654 nm emissions, respectively. In
Fig. 3d, the intensity ratios (IRs) of UCNPs/WO
3−x for three emissions are significantly dependent on the laser power. Both the IRs of
I521/I
540 and
I521/
I654 improve with the increase in the laser power. This is further observed by the power-dependent variation of colour coordinates in the CIE chromaticity diagram (Fig. S7c, ESI
†), clearly evidencing a shift in the UC emission colour towards the green emission with the increase in the laser power.
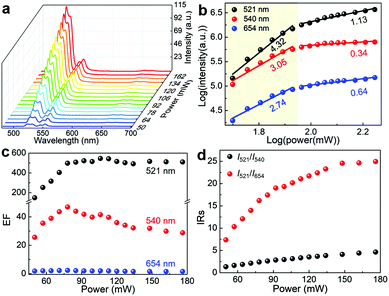 |
| Fig. 3 UCL spectra (a) of UCNPs/WO3−x hybrid under a 980 nm laser excitation with varying powers from 50 to 176 mW. Plot of log(I) versus log(P) for the three peak emissions of the UCNPs/WO3−x (b). EFs of UCNPs/WO3−x for three emissions as a function of laser power (c). IRs of UCNPs/WO3−x3−x as a function of laser power (d). | |
To verify that the selective UCL enhancement of the UCNPs/WO3−x hybrid is mainly caused by the SPR of plasmonic WO3−x, its SPR intensity was tuned by H2O2 oxidation to remove oxygen vacancies. Fig. 4a shows the UV-vis spectra of the obtained four WO3−x samples with different SPR intensities, which are labelled as WO3−x-1, WO3−x-2, WO3−x-3 and WO3−x-4, respectively. From WO3−x-4 to WO3−x-1, their SPR absorption for the 980 nm excitation light is gradually decreased. Four samples of UCNPs/WO3−x-1, UCNPs/WO3−x-2, UCNPs/WO3−x-3 and UCNPs/WO3−x-4 were coated on optical fibers following the same procedure. The power-dependent UCL intensities of the four samples under the same experimental parameters were measured as shown in Fig. S8 (ESI†). The enhancement factors (EFs) of three emissions at a laser power of 120 mW were calculated for the four samples as shown in the histogram in Fig. 4b. As WO3−x gradually transforms from non-plasmonic to plasmonic, EFs become larger, especially for the 521 nm emission, which exhibits a quadratic increase with the increase in the SPR intensity of WO3−x (Fig. S9a, ESI†). The power-dependent I521/I654 for the four samples is shown in Fig. S9b (ESI†). It is observed clearly that I521/I654 shows little change for the sample with non-plasmonic WO3−x, but exhibits a drastic increase as the SPR intensity of WO3−x increases, further demonstrating the plasmon-induced selective UCL enhancement. Such a distinct difference in the I521/I654 values leads to an obvious emission color change as shown in the CIE diagram at the 120 mW laser power (Fig. S9c, ESI†).
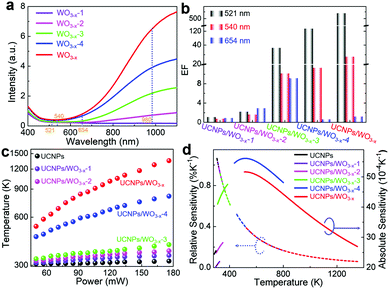 |
| Fig. 4 UV-vis spectra (a) of samples with different SPR intensities labelled as WO3−x-1, WO3−x-2, WO3−x-3, WO3−x-4, respectively. EFs histogram (b) of three emissions for different samples at a laser power of 120 mW. Local temperatures of different samples as a function of the laser power (c). Values of Sr and Sa for different samples as a function of temperature (d). | |
The UCL emission intensities at 521 and 540 nm are proportional to the population of the corresponding energy levels (2H11/2 and 4S3/2). The relative population of these two levels is thermally driven and conforms to the Boltzmann distribution. The IR of the emission intensities at 521 and 540 nm (I521/I540) is known as the thermometric parameter and can be expressed as follows39
| I521/I540 = B exp(−ΔE/kBT) | (2) |
where
I521 and
I540 are the intensities of the
2H
11/2 →
4I
15/2 and
4S
3/2 →
4I
15/2 emissions, respectively,
B is a pre-exponential constant, Δ
E is the separation energy between the
2H
11/2 and
4S
3/2 levels,
kB is the Boltzmann's constant and
T is the absolute temperature. Based on
eqn (2), the IR will increase as the local temperature of plasmonic WO
3−x increases, that is, the selective enhancement of the UCL emission at 521 nm can be achieved by plasmonic WO
3−x induced thermal effect. To further demonstrate this thermal effect, an additional sample with a polymer (PMMA) insulation layer inserted between UCNPs and WO
3−x was fabricated and denoted as UCNPs/PMMA/WO
3−x (see details in ESI
†). In this case, the thermal effect was largely suppressed. This result shows that, compared to UCNPs, the UCL intensity enhancement factors of the UCNPs/PMMA/WO
3−x sample are nearly the same (∼1.5) for the 521, 540 and 655 nm emissions (Fig. S10, ESI
†). No selective UCL enhancement was observed, indicating that the UCL selective enhancement of UCNPs/WO
3−x is mainly attributed to the plasmonic WO
3−x induced thermal effect.
As presented above, the IR of the 521 and 540 nm emissions increase with the increase in the laser power. According to the ratiometric method (see details with Fig. S11 in ESI†), the local temperatures of the samples at different laser powers can be determined as shown in Fig. 4c. As the SPR intensity of WO3−x increases, the initial local temperature becomes higher and the temperature range becomes wider. For the sample of UCNPs/WO3−x, the local temperature increases rapidly with the increase in the laser power and reaches up to over 1300 K at 176 mW. As reference, the temperature of UCNPs shows little change (306 to 318 K), which indicates the negligible influence of the laser-caused thermal effect. Consequently, the change in the temperature of UCNPs/WO3−x is mainly attributed to the photothermal effect of plasmonic WO3−x. Thus, the I521/I540 ratio can be used for temperature sensing. Besides, the XRD pattern of UCNPs/WO3−x after a 980 nm laser irradiation is shown in Fig. S12 (ESI†), which demonstrates their stable crystal structure during the experimental process.
For practical applications, the sensing capability of the samples could be quantitatively evaluated by thermal sensitivity, namely, the relative sensitivity (Sr) and absolute sensitivity (Sa). The values of Sr and Sa for different samples as a function of temperature are plotted in Fig. 4d (see details in ESI†). The close values of Sr (∼0.97% K−1 at 320 K) observed for these samples were due to their similar energy gaps. The Sa of the samples gradually increased with the increase in the temperature and achieved a maximum value of 0.0056 at 533 K for UCNPs/WO3−x-4. Besides sensitivity, another important parameter, namely, temperature resolution was also estimated (see details with Fig. S13 in ESI†). A summary of the temperature variation range (ΔT), the maximum values of Sr and Sa and the temperature resolution (δT) for different samples are shown in Table S2 in ESI.† By varying the laser power and SPR intensity of WO3−x, it is possible to detect a wide range of temperatures (306 to 1354 K) with resolutions between 0.17–1.18 K. The reported temperature sensing parameters for Er3+-based up-conversion materials are summarized in Table S3 in ESI.† The temperature-detection range achieved in this work is much larger and the temperature resolution is smaller than those for the previously reported Er3+-based up-conversion materials including some metallic plasmonic enhancements (36-fold enhancement in UCNPs/Au14).
In summary, a UCNPs/WO3−x hybrid was prepared and coated on an optical fiber via a simple method. The UCNPs/WO3−x hybrid was found to exhibit selective UCL enhancement of more than 500-fold on a 521 nm emission. The influence of different WO3−x (from non-plasmonic to plasmonic) on the UCL behaviours of UCNPs was investigated systemically, verifying that the significant selective enhancement of the UCNPs/WO3−x hybrid is attributed to the plasmon-induced electrical field and thermal effect of plasmonic WO3−x. The intensity ratio of the 521 to 540 nm emission increases significantly as the laser power increases, which is attributed to the thermal effect of plasmonic WO3−x. Thus, the hybrids can act as thermometers, exhibiting a wide temperature range (306–1354 K) and a high temperature resolution (0.17–1.18 K), which is superior or comparable to those previously reported, including some metallic plasmonic materials. This work not only provides a novel strategy of nonmetallic SPR to effectively improve the UCL of UCNPs, but also provides the UCNPs/WO3−x hybrid with potential applications in bioimaging, photothermal therapy and temperature sensing.
Experimental section
Preparation of the optical fiber
The optical fiber was fabricated by drawing a commercial single/multiple-mode optical fiber through a flame-heating technique.11 The polymer jacket of the fiber was stripped off to form a bare fiber with a diameter of 125 μm. The bare fiber was heated for ∼1 min to reach its melting point and was drawn at a speed of 0.2 mm s−1 until the diameter of the fiber was decreased to several micrometers over a 5 mm length. Fig. S14 (ESI†) shows the SEM image of the fiber (diameter: ∼9.4 μm) used in the experiment.
Preparation of the UCNPs
The NaYF4:Yb3+ (18%), Er3+ (2%) UCNPs were prepared by the well-known solvothermal method.40 YCl3 (0.8 mmol), YbCl3 (0.18 mmol) and ErCl3 (0.02 mmol) were added to a 50 mL flask containing 6 mL of oleic acid and 15 mL of octadecene. The mixture was heated to 160 °C to form a homogeneous solution, and then cooled to room temperature. 10 mL of methanol solution containing NaOH (2.5 mmol) and NH4F (4 mmol) was added into the flask and was stirred at 50 °C followed by heating to 100 °C. After the methanol was evaporated, the solution was heated to 300 °C and maintained for 1 h under a gentle argon flow. After the solution was naturally cooled to room temperature, the prepared UCNPs were collected by centrifugation and washed twice with ethanol.
Preparation of the UCNPs with a silica shell
0.1 mL CO-520, 6 mL of cyclohexane and a UCNPs cyclohexane solution (4 mL, 0.01 M) were mixed and stirred for 10 min. Then 0.4 mL of CO-520 and 0.08 mL of ammonia (30 wt%) were added followed by 20 min of ultrasonication to form a transparent emulsion. Then, 0.04 mL of tetraethoxysilane (TEOS) was added into the solution with continuous stirring. Finally, the silica-coated UCNPs were purified using acetone and ethanol.
Preparation of plasmonic WO3−x
The plasmonic WO3−x were prepared via a simple solvothermal method.24 First, 50 mg of hexacarbonyltungsten (W(CO)6) powder was dissolved in 20 mL of ethanol under constant stirring to form a yellow precursor solution. Then, 5 mL of the above solution and 25 mL of ethanol were transferred into a Teflon-lined stainless steel autoclave. Subsequently, the autoclave was heated up to 180 °C and kept for 12 h, and then cooled to room temperature. The obtained blue colour sample was separated via centrifugation, washed three times with ethanol, and finally dried in an oven at 60 °C for 12 h.
Preparation of the UCNPs/WO3−x coated optical fiber
First, 1 mg of WO3−x was added to 2 mL alcohol solution under ultrasonication for 40 min. Then, 20 μL of the UCNPs ethanol dispersion (concentration: 1 mg mL−1) was added to the WO3−x solution and magnetically stirred at 25 °C for 10 min to obtain a uniform mixture of UCNPs/WO3−x. Finally, the optical fiber was vertically immersed in the above mixture and then placed in an oven at 35 °C for 1 h. The UCNPs/WO3−x sample would self-assemble uniformly onto the surface of the fiber with the slow evaporation of ethanol. In addition, five control samples were also prepared by a similar method: one was a blank control sample of UCNPs without WO3−x; the other four controls were hybrid samples of UCNPs and varied WO3−x with different SPR intensities, labelled as UCNPs/WO3−x-1, UCNPs/WO3−x-2, UCNPs/WO3−x-3 and UCNPs/WO3−x-4.
Characterization
The SEM images were obtained by an FEI APREO SEM operated at 5 kV. The TEM images and HRTEM images were taken with a JEOL JEM-2100F at an operating voltage of 200 kV. The XRD patterns of samples were recorded on an X-ray diffractometer (Bruker D8 Advance, Germany) with Cu Kα irradiation (λ = 0.15418 nm) operating at 40 kV and 35 mA. The XPS was performed on a THERMO ESCALAB 250 Xi with a monochromatic Al Kα X-ray source (1486.68 eV, 12 kV). The UV-vis absorption spectra of the samples were recorded on a UV-vis UV-3600 spectrophotometer (Shimadzu). The UCL images and spectra were measured by a micro-spectrophotometer (CRAIC, 20/20 PV, USA) incorporating a charge-coupled device (CCD). The specifications of the objective in the microscope, including magnification, numerical aperture and working distance, are 100×, 0.85, and 3.4 mm, respectively. A band-pass filter (450–700 nm) was used to suppress the excited light of 980 nm. The UCL decay curves of the samples excited by an external 980 nm laser were measured using a phosphorescence spectrometer (FLS980, Edinburgh) equipped with a time-correlated single-photon counting system.
Conflicts of interest
There are no conflicts to declare.
Acknowledgements
This work was supported by the National Natural Science Foundation of China (No. 21703083, 51872125 and 11804120), Guangdong Natural Science Funds for Distinguished Young Scholar (No. 2018B030306004), the Natural Science Foundation of Guangdong Province (2017A030310463) and Project supported by GDUPS (2018).
References
- Y. S. Liu, D. T. Tu, H. M. Zhu and X. Y. Chen, Chem. Soc. Rev., 2013, 42, 6924–6958 RSC.
- W. Feng, C. M. Han and F. Y. Li, Adv. Mater., 2013, 25, 5287–5303 CrossRef CAS PubMed.
- S. L. Gai, C. X. Li, P. P. Yang and J. Lin, Chem. Rev., 2014, 114, 2343–2389 CrossRef CAS PubMed.
- M. Kataria, K. Yadav, S. Y. Cai, Y. M. Liao, H. I. Lin, T. L. Shen, Y. H. Chen, Y. T. Chen, W. H. Wang and Y. F. Chen, ACS Nano, 2018, 12, 9596–9607 CrossRef CAS PubMed.
- J. N. Liu, W. B. Bu and J. L. Shi, Acc. Chem. Res., 2015, 48, 1797–1805 CrossRef CAS PubMed.
- K. C. Liu, Z. Y. Zhang, C. X. Shan, Z. Q. Feng, J. S. Li, C. L. Song, Y. N. Bao, X. H. Qi and B. Dong, Light: Sci. Appl., 2016, 5, e16136 CrossRef CAS PubMed.
- Q. Liu, J. J. Peng, L. N. Sun and F. Y. Li, ACS Nano, 2011, 5, 8040–8048 CrossRef CAS PubMed.
- F. Y. Li, Y. Du, J. N. Liu, H. Sun, J. Wang, R. Q. Li, D. Kim, T. Hyeon and D. S. Ling, Adv. Mater., 2018, 30, 1802808 CrossRef PubMed.
- D. L. Zhou, D. L. Liu, J. J. Jin, X. Chen, W. Xu, Z. Yin, G. C. Pan, D. Y. Lia and H. W. Song, J. Mater. Chem. A, 2017, 5, 16559 RSC.
- J. X. Liu, H. L. He, D. Xiao, S. T. Yin, W. Ji, S. Z. Jiang, D. Luo, B. Wang and Y. J. Liu, Materials, 2018, 11, 1833 CrossRef PubMed.
- Y. X. Xue, C. J. Ding, Y. Y. Rong, Q. Ma, C. D. Pan, E. Wu, B. T. Wu and H. P. Zeng, Small, 2017, 13, 1701155 CrossRef PubMed.
- N. J. Greybush, M. Saboktakin, X. C. Ye, C. D. Giovampaola, S. J. Oh, N. E. Berry, N. Engheta, C. B. Murray and C. R. Kagan, ACS Nano, 2014, 8, 9482–9491 CrossRef CAS PubMed.
- M. Saboktakin, X. Ye, U. K. Chettiar, N. Engheta, C. B. Murray and C. R. Kagan, ACS Nano, 2013, 7, 7186–7192 CrossRef CAS PubMed.
- W. N. Zhang, J. Li, H. X. Lei and B. Li, ACS Appl. Mater. Interfaces, 2017, 9, 42935–42942 CrossRef CAS PubMed.
- H. L. Liu, J. H. Xu, H. Wang, Y. J. Liu, Q. F. Ruan, Y. M. Wu, X. G. Liu and J. K. W. Yang, Adv. Mater., 2019, 1807900 CrossRef PubMed.
- W. Zhang, F. Ding and S. Y. Chou, Adv. Mater., 2012, 24, OP236 CAS.
- D. W. Lu, S. K. Cho, S. Ahn, L. Brun, C. J. Summers and W. Park, ACS Nano, 2014, 8, 7780–7792 CrossRef CAS PubMed.
- Z. Yin, H. Li, W. Xu, S. B. Cui, D. L. Zhou, X. Chen, Y. S. Zhu, G. S. Qin and H. W. Song, Adv. Mater., 2016, 28, 2518–2525 CrossRef CAS PubMed.
- K. T. Lee, J. H. Park, S. J. Kwon, H. K. Kwon, J. Kyhm, K. W. Kwak, H. Seong Jang, S. Y. Kim, J. S. Han, S. H. Lee, D. H. Shin, H. Ko, I. K. Han, B. K. Ju, S. H. Kwon and D. H. Ko, Nano Lett., 2015, 15, 2491–2497 CrossRef CAS PubMed.
- S. J. Kwon, G. Y. Lee, K. Jung, H. S. Jang, J. S. Park, H. Ju, I. K. Han and H. Ko, Adv. Mater., 2016, 28, 7899–7909 CrossRef CAS PubMed.
- J. A. Schuller, E. S. Barnard, W. Cai, Y. C. Jun, J. S. White and M. L. Brongersma, Nat. Mater., 2010, 9, 193 CrossRef CAS PubMed.
- X. Liu and M. T. Swihart, Chem. Soc. Rev., 2014, 43, 3908–3920 RSC.
- D. Dorfs, T. Härtling, K. Miszta, N. C. Bigall, M. R. Kim, A. Genovese, A. Falqui, M. Povia and L. Manna, J. Am. Chem. Soc., 2011, 133, 11175–11180 CrossRef CAS PubMed.
- Z. Z. Lou, M. S. Zhu, X. G. Yang, Y. Zhang, M. H. Whangbo, B. J. Li and B. B. Huang, Appl. Catal., B, 2018, 226, 10–15 CrossRef CAS.
- J. M. Luther, P. K. Jain, T. Ewers and A. P. Alivisatos, Nat. Mater., 2011, 10, 361–366 CrossRef CAS PubMed.
- Z. Y. Zhang, Y. Liu, Y. R. Fang, B. S. Cao, J. D. Huang, K. C. Liu and B. Dong, Adv. Sci., 2018, 5, 1800748 CrossRef PubMed.
- J. Q. Yan, T. Wang, G. J. Wu, W. L. Dai, N. J. Guan, L. D. Li and J. L. Gong, Adv. Mater., 2015, 27, 1580–1586 CrossRef CAS PubMed.
- J. J. Qiu, Q. F. Xiao, X. P. Zheng, L. B. Zhang, H. Y. Xing, D. L. Ni, Y. Y. Liu, S. J. Zhang, Q. G. Ren, Y. Q. Hua, K. L. Zhao and W. B. Bu, Nano Res., 2015, 8, 3580–3590 CrossRef CAS.
- Z. Z. Lou, Q. Gu, Y. S. Liao, S. J. Yu and C. Xue, Appl. Catal., B, 2016, 184, 258–263 CrossRef CAS.
- J. Li, W. N. Zhang, Q. G. Li and B. J. Li, Nanoscale, 2015, 7, 2889–2893 RSC.
- R. G. Geitenbeek, T. Prins, W. Albrecht, A. Blaaderen, B. M. Weckhuysen and A. Meijerink, J. Phys. Chem. C, 2017, 121, 3503–3510 CrossRef CAS PubMed.
- Z. Z. Lou, Q. Gu, L. Xu, Y. S. Liao and C. Xue, Chem. – Asian J., 2015, 10, 1291 CrossRef CAS PubMed.
- Y. Wang, X. X. Wang, Y. H. Xu, T. Chen, M. L. Liu, F. S. Niu, S. Wei and J. Q. Liu, Small, 2017, 13, 1603689 CrossRef PubMed.
- M. Qiu, D. Q. Zhu, X. C. Bao, J. Y. Wang, X. F. Wang and R. Q. Yang, J. Mater. Chem. A, 2016, 4, 894–900 RSC.
- Z. Y. Hou, C. X. Li, P. A. Ma, Z. Y. Cheng, X. J. Li, X. Zhang, Y. L. Dai, D. M. Yang, H. Z. Lian and J. Lin, Adv. Funct. Mater., 2012, 22, 2713–2722 CrossRef CAS.
- Z. Yin, H. Li, W. Xu, S. B. Cui, D. L. Zhou, X. Chen, Y. S. Zhu, G. S. Qin and H. W. Song, Adv. Mater., 2016, 28, 2518–2525 CrossRef CAS PubMed.
- M. Pollnau, D. R. Gamelin, S. R. Lüthi, H. U. Güdel and M. P. Hehlen, Phys. Rev. B: Condens. Matter Mater. Phys., 2000, 61, 3337–3346 CrossRef CAS.
- H. C. Liu, K. Huang, R. R. Valiev, Q. Q. Zhan, Y. Zhang and H. Ågren, Laser Photonics Rev., 2018, 12, 1700144 CrossRef.
- M. L. Debasu, D. Ananias, I. P. Santos, L. M. Liz-Marzán, J. Rocha and L. D. Carlos, Adv. Mater., 2013, 25, 4868–4874 CrossRef CAS PubMed.
- R. A. Jalil and Y. Zhang, Biomaterials, 2008, 29, 4122–4128 CrossRef PubMed.
Footnote |
† Electronic supplementary information (ESI) available: Details of power-dependent UCL spectra of UCNPs, CIE chromaticity diagram of UCNPs, power-dependent UCL spectra of the control samples, the ratiometric method and so on. See DOI: 10.1039/c9nh00106a |
|
This journal is © The Royal Society of Chemistry 2019 |