DOI:
10.1039/C8NH00340H
(Communication)
Nanoscale Horiz., 2019,
4, 747-756
Ultrasound activation of liposomes for enhanced ultrasound imaging and synergistic gas and sonodynamic cancer therapy†
Received
8th October 2018
, Accepted 31st January 2019
First published on 1st February 2019
Abstract
Ultrasound (US) imaging is widely applied in hospital and clinical settings due to its non-invasiveness, controllability, and high tissue-penetrating ability. Moreover, sonodynamic therapy (SDT) activated by US is a safe cancer therapeutic strategy with a deep tissue penetration effect. Simultaneous integration of US imaging contrast agents, controllable drug delivery vehicles, and effective therapeutic drugs in a single theranostic nanoplatform is a promising strategy for cancer therapy. Herein, we developed a 2,2′-azobis[2-(2-imidazolin-2-yl)propane]dihydrochloride (AIPH)-loaded liposome (Lip-AIPH) that can simultaneously generate gas bubbles and a high concentration of reactive oxygen species (ROS) under US irradiation. In vivo experimental results confirmed that the generated gas and alkyl radicals were independent of oxygen generation and were successfully used for synergistic gas therapy and SDT in a hypoxic tumor microenvironment. More importantly, the generated gas bubbles, when used as a powerful US contrast agent, greatly enhanced the US contrast to guide cancer therapy. As an US-activated theranostic agent, the liposome enhanced the US imaging and exhibited an improved anticancer efficacy, and it may prove promising for US imaging-guided hypoxic tumor therapy with deep tissue penetration.
Conceptual insights
Herein, we developed a biocompatible liposome from an ultrasound (US) activated organic sensitizer-loaded liposome that can simultaneously generate gas bubbles (N2) and a high concentration of alkyl radicals independent oxygen under US irradiation. Sonodynamic therapy (SDT) and US imaging activated by US of the liposome is a safe cancer therapeutic strategy and is able to overcome the restrictions of traditional photo-triggered therapeutic modalities such as their relatively low penetration and side effects. Moreover, the reported liposome is different from previously reported oxygen-dependent nanoplatforms that only generated gas or alkyl radicals. The main advantages of the functional liposome are (1) the generated gas and alkyl radicals were independent of oxygen generation and were successfully used for synergistic gas therapy and SDT in a hypoxic tumor microenvironment, and (2) the generated gas bubbles greatly enhanced US imaging to guide cancer therapy.
|
1. Introduction
Ultrasound (US) is a widely applied technique in hospital and clinical settings due to its convenience, low cost, and non-invasive modality, as well as its controllability and high tissue-penetrating ability.1,2 Nevertheless, its low sensitivity partly limits its application in localization of drug delivery carriers in vivo. In recent years, chemical agents that generate microbubbles have been extensively researched to enhance ultrasonic imaging to elucidate the location of the carriers and provide real-time diagnostic images.3–6 In addition, microbubbles can clearly change the in situ acoustic environment of tumors, providing more nucleation sites and reducing the cavitation threshold intensity.7–10 Based on the above properties, sonodynamic therapy (SDT) combined with microbubbles has emerged as a new form of ultrasonic therapy. The SDT can convert oxygen into reactive oxygen species (ROS) with the aid of sonosensitizers;11,12 not only that, SDT can also overcome the depth-penetration barrier of photo-triggered therapeutic modalities.13–15 For example, photodynamic therapy (PDT) is a typical form of photo-triggered therapy involving a photosensitizer and light. However, the limited penetration depth of the light reduced the PDT efficiency in vivo and is even not applicable for some diseases. SDT can also reduce damage to the surrounding normal tissue by effectively releasing drugs at a specific site.16,17 In recent years, the bubble liposomal systems have received widespread attention.18–21 Combined with bubbles, low frequency US-triggered delivery of drugs encapsulated within the liposomes could be controlled by adjusting the power density and irradiation time of the US.1,18–22 The development of novel therapeutic modalities that are highly efficient and have mitigated side effects is of great significance in combating cancer. In the past few years, microbubble-combined materials have been widely studied for drug delivery,23–26 SDT,27,28 and gas therapy.10,29,30 Nevertheless, few strategies have been developed for the successful integration of US imaging contrast agents, controllable drug delivery vehicles, and effective therapeutic drugs.
For the traditional cancer therapy strategies, such as PDT and radiotherapy, their performance usually depends on the oxygen content.31–33 However, a hypoxic microenvironment is common in most solid tumors due to the distorted tumor blood vessels and the rapid proliferation of tumor cells, leading to a reduction in the efficacy of oxygen-dependent therapies.34 In order to overcome the restriction of hypoxia, various strategies have been explored, including hyperbaric oxygen injection,35 oxygen-carrying microbubbles,36,37 and in situ oxygen production.31,38 However, these approaches are often accompanied by tedious processes and potential issues, such as side effects in hyperbaric oxygen injection, large size and poor stability in oxygen delivery, etc.34
Interestingly, a water-soluble azo compound, 2,2′-azobis[2-(2-imidazolin-2-yl)propane]dihydrochloride (AIPH), can simultaneously generate alkyl radicals and nitrogen gas.39 Even under hypoxic conditions, the alkyl radicals can directly oxidize cell components or induce oxidative-stress in biological systems, leading to cell death. Meanwhile, US can not only penetrate into the deep tissues but also control the generation of alkyl radicals by adjusting the power density and irradiation time. To date, the generation of nitrogen gas bubbles has been a special treatment in cancer therapy, and it could be used as an US contrast agent due to its non-linear oscillation in an US field.5,40 Previous research has led to the use of microbubbles as a potential therapeutic agent for gas therapy, including CO2 bubbling to cause cavitation-mediated tumor destruction.29 However, few studies have reported on the exploration of all-in-one, integrated nanoplatforms for use as both therapeutic and imaging agents.
Herein, we developed a new type of sonodynamic theranostic AIPH-loaded liposome (Lip-AIPH), which simultaneously generates gas bubbles and alkyl radicals upon US irradiation. Based on these properties, the US-activated liposome can potentially be used for enhanced US imaging and synergistic gas and SDT cancer therapy (Scheme 1). Liposomes have been widely used as drug-delivery vehicles able to reduce the drug toxicity and increase the drug concentrations in tumor cells.41–45 Moreover, liposomes are not limited by the shortcomings of inorganic nanocarriers, whose side effects and systemic toxicity limit the maximum tolerable dose.8,44 The Lip-AIPH liposomes of approximately 100 nm in diameter were prepared by the self-assembly of lipids and AIPH using a common film rehydration method, and proved to be suitable for in vivo applications. Upon US irradiation, the loaded AIPH was decomposed to efficiently generate nitrogen gas. The generated gas bubbles led to the disruption of the liposomes and AIPH release, producing free radicals without being dependent on oxygen. The technique was therefore applied in synergistic gas and SDT therapy in a hypoxic tumor microenvironment. More interestingly, the gas bubbles can act as feasible contrast agents for enhancing US imaging to guide cancer therapy.46–48 The developed strategy offers great promise to overcome the issues related to penetration depth and the limitations of therapeutic strategies in hypoxic tumors. The distinctive features of the Lip-AIPH liposomes not only make them suitable for the treatment of deep-seated tumors, but also as multifunctional delivery systems to improve cancer diagnosis. We believe that the Lip-AIPH liposomes have the potential to be an on-demand US-based therapeutic and imaging agent.
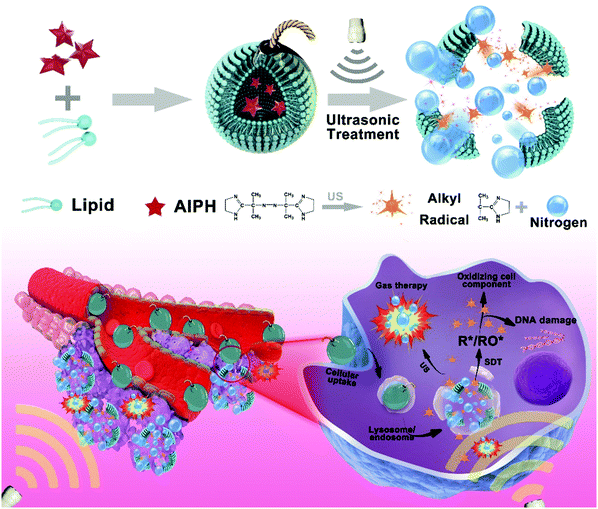 |
| Scheme 1 Schematic illustration of the synthetic process of Lip-AIPH and the generation of nitrogen gas and alkyl radicals and synergistic sonodynamic-gas therapy against solid tumor with contrast enhanced US imaging. The Lip-AIPHs as liposomes enter the blood stream and then target tumor tissue by passive targeting, and internalize into the cells by endocytosis and destroy the cells like a bomb under the trigger of ultrasound. | |
2. Results and discussion
2.1. Preparation and characterization of Lip-AIPH
AIPH was loaded into the liposomes by self-assembly of AIPH, lipid 1,2-dipalmitoyl phosphatidylcholine, amphiphilic PEGylated phospholipids, and cholesterol. The as-prepared AIPH-loaded liposomes had a uniform spherical nanostructure, as observed by TEM (Fig. 1a and b) and SEM (Fig. S1, ESI†). As indicated by the dynamic light scattering results, the average liposome particle size was approximately 100 nm, with a uniform size distribution (Fig. 1c), in agreement with the TEM results. The Lip-AIPH solution was shown to be highly stable when stored in phosphate buffer solution (PBS), fetal bovine serum (FBS), and cell culture medium (Dulbecco's modified Eagle's medium supplemented with 10% fetal bovine serum) for 1 week (Fig. S2, ESI†); no aggregation and no variation in size or surface charge were observed after this time (Fig. S3 and S4, ESI†). The surface conjugated PEG not only enhanced the dispersion but also inhibited the formation of a protein corona on the surface of the liposomes by resisting nonspecific protein adsorption. The characteristic UV absorption of AIPH at 356 nm was observed in the UV-Vis spectra of Lip-AIPH,49 suggesting that the AIPH molecules were successfully encapsulated into the liposomes (Fig. 1d). The loading capacity of AIPH was calculated to be 14.5 mg per g of liposome through assessment of the characteristic absorption of AIPH at 356 nm using a concentration curve (Fig. S5, ESI†). Furthermore, hemolysis experiments confirmed the safety of Lip-AIPH even at high concentrations (Fig. S6, ESI†).
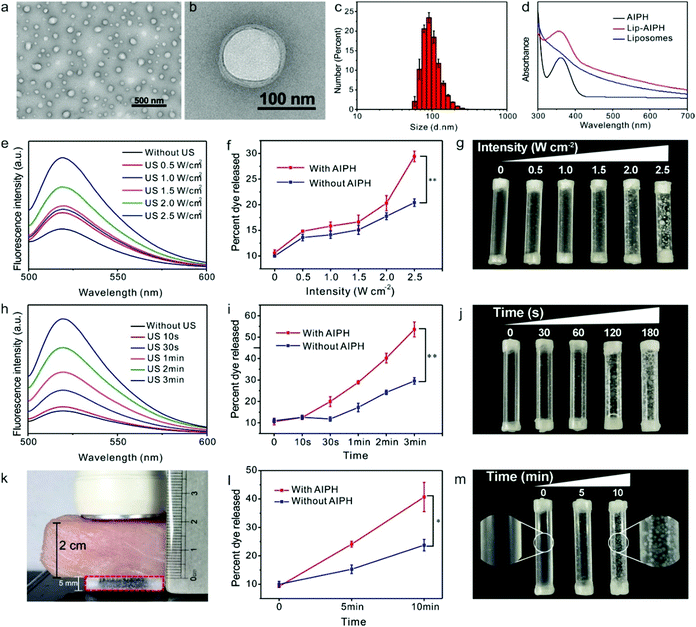 |
| Fig. 1 (a) TEM images of Lip-AIPH (scale bar: 500 nm). (b) TEM images of single Lip-AIPH (scale bar: 100 nm). (c) Size distribution of Lip-AIPH. (d) UV spectra of AIPH, liposomes and Lip-AIPH. (e) Fluorescence based assay of the FITC dye released from Lip-AIPH after 2 min isolation at different US intensities (1.0 MHz). (f) Release profiles of FITC dye from Lip-AIPH and blank liposomes under US irradiation at different power densities (2 min, 1.0 MHz). (g) Photographs of bubble generation of the Lip-AIPH solution after US irradiation with different intensities (1.0 MHz for 2 min). (h) Fluorescence based assay and (i) release profiles and (j) photographs of bubble generation at different US durations (1.0 MHz, 2.5 W cm−2). (k) Using 2 cm pork blocks as a medium and a tube (diameter 5 mm, length 2.5 cm) as a vessel. (l) Release profiles of dye and (m) photographs of bubble generation after different US durations by using 2 cm pork blocks as a medium. All data are means ± s.d.; n = 3. *P < 0.01, **P < 0.001, ***P < 0.0001. | |
After US irradiation, the absorption intensity of the characteristic AIPH peak at 356 nm gradually decreased (Fig. S7, ESI†), showing that Lip-AIPH underwent controlled release behavior. Nevertheless, it was difficult to accurately calculate the concentration of the released AIPH. Therefore, fluorescein isothiocyanate (FITC) dye was loaded into Lip-AIPH and its fluorescence signal was used to test the release behavior of the liposome. The experiment was processed in a plastic tube (diameter: 5 mm, length: 2.5 cm, sealed by a latex membrane) at a Lip-AIPH concentration of 1 mg mL−1 (500 μL) (Fig. 1e). Their leakage of the cargos in the release profiles in terms of release percentages showed satisfactory packaging and release effects (Fig. S8, ESI†). Moreover, the encapsulated AIPH molecules generate gas bubbles when triggered by ultrasonic stimulation.50–52 The generated microbubbles can oscillate and collapse rapidly, causing rapid drug release from bubbles and the generation of shock waves, shear forces, and microstreams,8 potentially serving as an US-sensitive delivery system to induce drug release.23,49 To demonstrate that the generated bubbles can trigger drug release, the release behavior of FITC-loaded Lip-AIPH following US treatment was also investigated (Fig. 1f). In the blank liposomes, the release of FITC dye following US treatment was greatly reduced compared to liposomes encapsulating AIPH, indicating that the generated gas was able to break the liposome shell and accelerate the release of encapsulated AIPH radicals and FITC dye molecules. The destruction of the liposome shell was observed by TEM images (Fig. S9, ESI†), with an accompanying change in the size distribution from 100 nm to 80 nm (Fig. S10, ESI†). Upon an increase in US intensity, there was a gradual increase in the amount of dye released, indicating that US (1.0 MHz, 2 min, 50% duty cycle) enabled the control of the dye release. When the US intensity reached 2.5 W cm−2, the release of the dye was more evident than that without AIPH co-encapsulation, attributed to the presence of US-induced gas bubbles. Indeed, the increase in the number of bubbles in the plastic tube was relevant to the concentration of the released dye (Fig. 1g). Similarly, with the prolonging of US-treatment duration, there was a corresponding increase in the amount of dye released, showing that US can modulate the extent of dye release by duration as well as intensity (Fig. 1h). The macroscopic appearance of gas bubbles in AIPH plays a positive role in drug delivery (Fig. 1i). The greatest amount of dye release was observed at 2.5 W cm−2, 1.0 MHz, 3 min, and 50% duty cycle US, suggesting that US could be used as a good trigger method (Fig. 1j). Thus, US provided flexible control of the magnitude of drug dosing.
To illustrate the responsive release behavior of the Lip-AIPH in a deep tissue upon US irradiation, a plastic tube (diameter: 5 mm, length: 2.5 cm, sealed with a latex membrane) containing Lip-AIPH (500 μL, 1 mg mL−1) was placed under a piece of pork meat with 2 cm thickness, used as simulated tissue (Fig. 1k). Following US (2.5 W cm−2, 1.0 MHz) irradiation, a large FITC fluorescence signal was detected, suggesting a deep penetration effect of the US (Fig. 1l). After 5 min of US irradiation (2.5 W cm−2, 1.0 MHz), a large amount of gas bubbles was also observed in the tube, which dramatically increased and dispersed when increasing the US irradiation time to 10 min (Fig. 1m). According to the above results, we speculated that the Lip-AIPHs have the ability to generate gas bubbles with high efficiency in deep tissue upon US irradiation.
2.2.
In vitro US imaging
To assess the enhanced US imaging efficiency of the Lip-AIPHs, we further systematically studied the generation of microsized gas bubbles upon US irradiation (1.0 MHz, 2.5 W cm−2, 5 min, 50% duty cycle) by confocal microscopy. Upon US irradiation, bright gas bubbles were observed (Fig. 2a) due to the triggering of gas formation and release from the Lip-AIPH by breaking the liposome shell. To further demonstrate the effect of enhanced US imaging, the samples of PBS (control), liposomes, and Lip-AIPH were placed in three transparent plastic tubes (diameter 5 mm, sealed with a latex membrane). The tubes were fixed in a tank filled with degassed water for US imaging. PBS and liposomes showed a negligible enhanced effect of US imaging even after prolonging the US radiation time to 30 min (Fig. 2b). In contrast, the Lip-AIPH showed obvious US contrast signals, with echo intensity gradually increasing with an increase in the US irradiation time (Fig. 2b). This was attributed to the microbubbles providing a significant acoustic impedance mismatch between the gas bubbles and the surrounding media, thereby achieving a great enhancement in contrast under US imaging.5 The average gray values of the US image increased from 12.7 to 53.0 due to the generated gas bubbles by Lip-AIPH, exhibiting a more than 4.2-fold increase in comparison to the PBS and blank liposome groups, which showed no significant change (Fig. 2c). Moreover, the rate constant for signal amplification of Lip-AIPHs upon US irradiation showed that a rapid signal amplification was achieved during the irradiation period (Fig. S11, ESI†).
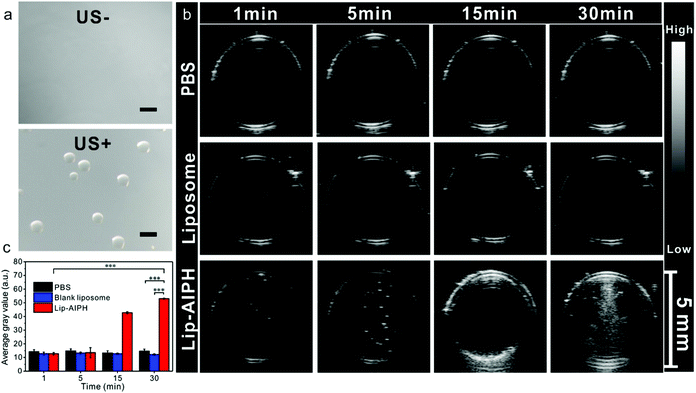 |
| Fig. 2 (a) Optical microscopic images of bubble generation of the Lip-AIPH solution before and after US irradiation (1.0 MHz, 2.5 W cm−2 for 5 min) (scale bar: 10 μm). (b) In vitro US images of PBS, blank liposomes and Lip-AIPH after US irradiation. (c) The corresponding gray values of PBS, blank liposome and Lip-AIPH solutions after US irradiation (1.0 MHz, 2.5 W cm−2, 1 min). All data are means ± s.d.; n = 3. ***P < 0.001. | |
2.3.
In vitro generation of alkyl free radicals triggered by US irradiation
It has been previously demonstrated that alkyl radicals can induce apoptosis by producing both alkoxyl and peroxyl radicals and can also directly oxidize cellular components even under hypoxic conditions.50,53–56 To determine the production of alkyl free radicals under US irradiation, we first utilized 2,2′-azino-bis(3-ethylbenzothiazoline-6-sulfonic acid) free radicals (ABTS*) as the indicator,39 which exhibit a characteristic absorbance at 734 nm following the reaction between ABTS and the free radicals from Lip-AIPH (Fig. S12, ESI†). As the power density of US increased, the characteristic absorption peak of ABTS* gradually increased, suggesting the efficient production of alkyl radical species from Lip-AIPH (Fig. 3a). Similarly, the characteristic absorption peak increased with the prolonged duration of US irradiation (Fig. 3b), indicating that the production of alkyl radicals is not only controlled by US intensity but also by irradiation duration. To further confirm that the alkyl radical species can induce the generation of ROS, we also used 2′,7′-dichlorofluorescein diacetate (DCFH-DA) as a ROS production indicator (Fig. 3c and Fig. S13, ESI†). Similarly, the fluorescence signal of DCFH-DA at 525 nm gradually increased with the increase in the US irradiation time (Fig. 3c), indicating that the local free radical concentration is controllable by adjusting the power density and duration of US, and thus suggesting that spatial-, temporal-, and dosage-controlled release is possible.
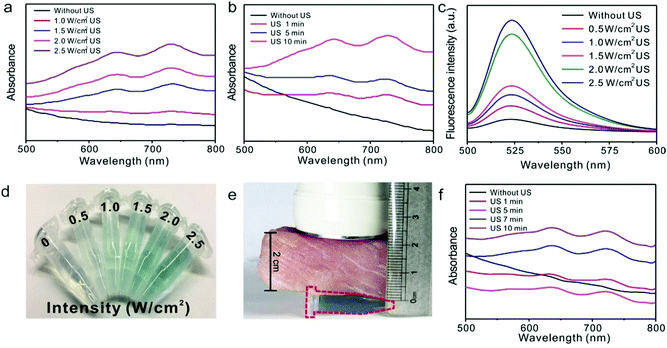 |
| Fig. 3 UV-Vis spectra of the Lip-AIPH solution containing ABTS and further exposure to US irradiation with (a) different intensities (1.0, 1.5, 2.0 and 2.5 W cm−2) and (b) different durations (1, 5 and 10 min). (c) The fluorescence of DCFH indicating ROS generated by Lip-AIPH under different US intensities (0.5, 1.0, 1.5, 2.0 and 2.5 W cm−2). (d) Photographs of the color change under different US irradiations (0, 0.5, 1.0, 1.5, 2.0 and 2.5 W cm−2) by using ABTS as an indicator. (e) Photographs of a 2 cm pork block as a barrier and a 0.6 mL centrifuge tube as a vessel, and (f) the absorption spectrum of ABTS* generation under US irradiation (1.0 MHz, 2.5 W cm−2). | |
As the power intensity of US increased, the color of the solution gradually changed from colorless to blue (Fig. 3d). In order to assess the US-triggered radical generation upon US irradiation in deep tissue, a piece of pork meat with 2 cm thickness was placed between the tube and the US probe. The solution in the tube turned blue after US (1.0 MHz, 2.5 W cm−2) irradiation, indicating that the free radicals were generated upon US irradiation and that US can penetrate into the tissue to trigger the AIPH functionality (Fig. 3e). Moreover, the generation efficiency of the radical increased with increasing US irradiation time (Fig. 3f). The above results indicate that this novel, integrated Lip-AIPH liposome is not only a suitable contrast agent for enhanced US imaging but also provides control of free radical production and drug delivery.
2.4. Cell therapy studies of synergistic gas and SDT therapy
Considering that Lip-AIPH can simultaneously generate gas bubbles and free radicals, we further evaluated whether Lip-AIPH could be effectively used for both hypoxia and normoxia cancer therapies by SDT (Fig. 4a). Firstly, no significant MCF-7 cell cytotoxicity was observed after US irradiation for 1 min at various US intensities (0.5, 1.5 and 2.5 W cm−2), suggesting the excellent safety of US (Fig. S14, ESI†). We then analysed the US-triggered potential cytotoxicity of Lip-AIPHs against MCF-7 cells (Fig. 4b) following incubation with Lip-AIPH at different concentrations (0, 30, 60, 250, 500 μg mL−1) for 24 h. The cells that did not undergo US irradiation exhibited no significant toxicity even at high Lip-AIPH concentrations (up to 500 μg mL−1). However, under US irradiation (1.0 MHz, 2.5 W cm−2, 1 min, 50% duty cycle), the viability of cells treated with Lip-AIPH decreased continuously with increasing Lip-AIPH concentration. The highest MCF-7 cell inhibition efficiency (74.8%) was demonstrated at a Lip-AIPH concentration of 500 μg mL−1.
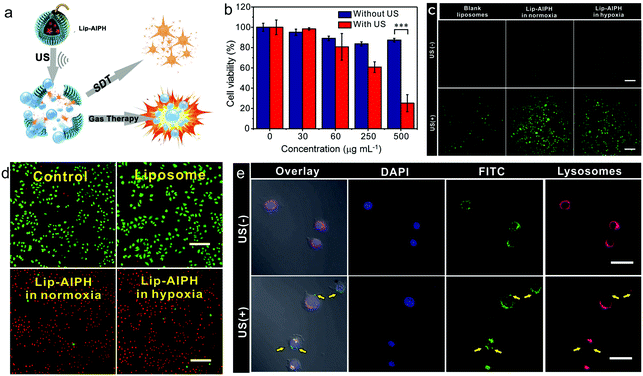 |
| Fig. 4
In vitro cell experiments. (a) The brief therapy mechanism of Lip-AIPH as a liposome. (b) Cell viability after treatment at different concentrations of Lip-AIPH with or without US irradiation (1.0 MHz, 2.5 W cm−2, 10 min) (***P < 0.0001). All data are means ± s.d.; n = 3. (c) Detection of AIPH-induced ROS in MCF-7 cells by DCFH-DA. The MCF-7 cells were treated with Lip-AIPH in normoxic and hypoxic culture media, respectively, for 2 h and then irradiated by US (1.0 MHz, 2.5 W cm−2, 10 min) (scale bar: 200 μm). (d) Confocal laser scanning microscopic images of MCF-7 cells costained with calcein AM (live cells, green fluorescence) and PI (dead cells, red fluorescence) after being incubated with PBS, blank liposome and Lip-AIPH (in normoxic and hypoxic culture media) under US irradiation (1.0 MHz, 2.5 W cm−2, 10 min) (scale bar: 200 μm). (e) US-induced lysosome membrane permeability in the cell (scale bar: 50 μm). | |
In order to assess the therapeutic mechanism of Lip-AIPH, we first investigated the generation of ROS within the cells triggered by US irradiation. Cells were incubated with the ROS indicator DCFH-DA, which has a weak green fluorescence signal that changes to 2′,7′-dichlorofluorescein (DCF), with a stronger green fluorescence signal, following reaction with the generated ROS. MCF-7 cells were co-incubated with Lip-AIPH for 4 h and then treated by US (1.0 MHz, 2.5 W cm−2, 1 min, 50% duty cycle). Without US irradiation, the cells showed no DCF green fluorescence signals (Fig. 4c and Fig. S15, ESI†), yet a significant fluorescence signal was observed only in the Lip-AIPH treated cells both under hypoxic and normoxic conditions after US irradiation (Fig. 4c). It is worth noting that a negligible green fluorescence signal was observed in the cells treated with blank liposomes under US irradiation (Fig. 4c). The above results confirm that Lip-AIPH exhibited a high ROS generation efficiency upon US irradiation.
The therapeutic effect of the Lip-AIPH liposome was further evaluated using the calcein-AM/propidium iodide double staining method. The green (calcein-AM) and red (propidium iodide) fluorescence represented the live and dead or late apoptosis cells, respectively. An intense red fluorescence signal was detected in Lip-AIPH-treated cells upon US irradiation under both hypoxic and normoxic conditions, indicating that most cells were killed (Fig. 4d). In contrast, a strong green fluorescence signal was observed in the cells treated with blank liposomes or without US, indicating that no cell death has been induced (Fig. S16, ESI†).
To further prove that US-induced cavitation and bubbles led to lysosome membrane destruction or at least led to an increase in the membrane permeability, we further assessed the drug diffusion effect of Lip-AIPH in MCF-7 cells (Fig. 4e). FITC was co-encapsulated with AIPH within the liposomes to trace the Lip-AIPH- and US-induced lysosome membrane permeability in the cell. It has been previously shown that the Lip-AIPH is mainly localized in the lysosomes following endocytosis. For cells without US irradiation (Fig. 4e), the green FITC fluorescence signal arising from Lip-AIPH overlapped with the red signal from the lysosome indicator, suggesting that the Lip-AIPH was indeed localized within the lysosome. In contrast, following US irradiation (1.0 MHz, 2.5 W cm−2, 20 s), most of the FITC and lysosome indicator signals were observed separately, with green fluorescence being observed also outside of the lysosome. This was ascribed to the gas bubbles activated by US being continuously generated and quickly growing, which led to disruption of the lysosomal membrane and release of lysosomal proteases, finally leading to cell necrosis (Fig. 4e).13,29,55
Gas-based cancer therapy is a novel therapeutic strategy that has shown high efficacy.10,27,56 Thus, the potential of using this gas generation nanoplatform in vivo for cancer therapy is of great interest. To demonstrate the effect of gas microbubbles, MCF-7 cells were sequentially treated with Lip-AIPH and US irradiation and the cellular morphology was recorded in real-time by confocal microscopy. With an increase in the US irradiation time, the amount of gas bubbles surrounding the cells was observed to increase gradually. More importantly, the cell lost its original morphology following its destruction by the generated gas bubbles (Fig. 5). Finally, cells were gradually killed after the US treatment (Fig. S17, ESI†) due to the instantaneous implosion of the generated gas bubbles by the US shock waves and cavitation-induced necrosis of the cells.29,55 However, without US irradiation, no obvious changes in the cell and membrane morphology were observed, with the cells remaining healthy, in agreement with the above results for cells in the control group. The combined effect of toxic alkyl free radicals and gas bubble generation resulted in a synergistic therapeutic effect of SDT and gas therapy. Thus, cell apoptosis and necrosis can be harnessed in conjunction to achieve a complementary cancer cell therapeutic regime with high efficacy.39,54,55
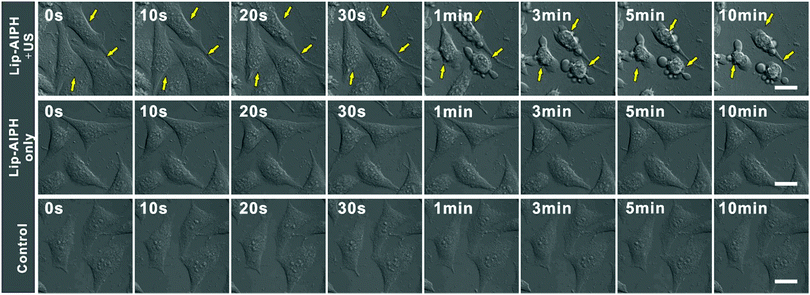 |
| Fig. 5 Confocal laser scanning microscopic observation of Lip-AIPH treated MCF-7 cells under US irradiation (1.0 MHz, 2.5 W cm−2) at different time points. Yellow arrows indicate the microbubble generation and significant cell membrane disruption (scale bar: 20 μm). | |
2.5.
In vivo enhanced US imaging
US imaging in clinical imaging and diagnosis commonly uses bubbles as contrast agents to enhance the image.3 To study the enhanced US imaging effects of Lip-AIPH in vivo, a MCF-7 tumor xenograft was established on nude mice (Fig. 6a). When the tumor reached a volume of approximately 90 mm,3 Lip-AIPH (200 μL, 500 μg mL−1) was intravenously injected into the tumor-bearing mice. At 24 h post-injection, the Lip-AIPH was efficiently accumulated within the tumor tissue, attributed to the typical enhanced permeability and retention effect for passively targeted tumor accumulation.57,58 US (1.0 MHz, 2.5 W cm−2) was then applied to irradiate the whole tumor region for 30 min and the US images and intensities of the tumor tissue were monitored and recorded. B-mode fundamental US imaging was employed with a transducer of 40 MHz, power intensity of 100%, and gain of 10 dB, and the images were captured before and after therapeutic US irradiation (5, 10, and 30 min). US signals were continuously enhanced in the tumor region (Fig. 6a), clearly showing the tumor morphology and its boundary within the normal tissue. Microbubble-enhanced US imaging was performed on three different groups (PBS, liposome, and Lip-AIPH), and the enhanced US imaging for the Lip-AIPH group was observed in the inner region of the tumor (Fig. 6a). The US signal was significantly enhanced due to the echo reflection of the generated gas bubbles from Lip-AIPH triggered by US irradiation. US irradiation for 30 min led to the most obvious signal enhancement within the tumor area. Moreover, there was an obvious increase in gray contrast values from 33.96 ± 3.45 to 61.37 ± 1.03 a.u. after 30 min US irradiation (Fig. 6b). However, there was no significant US imaging signal of the tumor in the PBS and empty liposome groups for any US irradiation intensity or duration. The increase in the contrast and average gray value was negligible in tumors of mice treated with PBS or Lip-AIPH without US treatment compared with those of the Lip-AIPH US-treated mice (Fig. 6b). Furthermore, it was demonstrated that Lip-AIPH exhibited a high gas production efficiency in vivo and can serve as an intelligent echogenic contrast agent for US imaging.
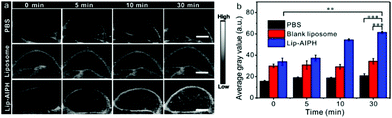 |
| Fig. 6 (a) In vivo US images of the tumor at different post-injection time points (0, 5, 10, 30 min) after US irradiation (1.0 MHz, 2.5 W cm−2, 10 min) (scale bar: 2 mm). (b) Average mean gray value of the US images signal (**P < 0.001, ***P < 0.0001). | |
2.6.
In vivo synergistic sonodynamic and gas cancer therapy
Encouraged by the high in vitro therapeutic effect and the excellent US imaging ability of Lip-AIPH, we further investigated the synergistic tumor therapeutic efficacy of SDT (the generated alkyl free radical) and gas therapy (the instantaneous implosion of gas bubbles). The MCF-7 tumor-bearing mice were randomly divided into four groups of five mice each, as follows: (1) control, (2) US only, (3) Lip-AIPH, and (4) Lip-AIPH with US irradiation. Lip-AIPH was intravenously injected into the tumor-bearing mice when the tumor reached a volume of approximately 90 mm.3 US irradiation (1.0 MHz, 2.5 W cm−2, 50% duty cycle, 10 min) was repeated on the first and third days during the therapeutic process and the in vivo treatment efficacy of Lip-AIPH was evaluated by measuring the tumor volume every 2 days (Fig. 7a and b). Tumors treated with US showed a slight suppression in growth possibly due to the effects of sonication, whereas those in the PBS and blank liposome groups kept growing at a similar rate (Fig. 7a). In contrast, a highly significant antitumor effect was achieved in mice in the group of Lip-AIPH with US irradiation, which exhibited a much slower tumor growth than other groups (P < 0.0001). Due to the excellent SDT and gas therapeutic effects, the survival rate of MCF-7 tumor-bearing mice in the Lip-AIPH plus US group was considerably prolonged over the monitoring duration of 40 days (Fig. 7c). Furthermore, the body weight of mice in the control and treatment groups showed a negligible difference, suggesting that all mice continued to mature without any noticeable toxic effects (Fig. S18, ESI†).
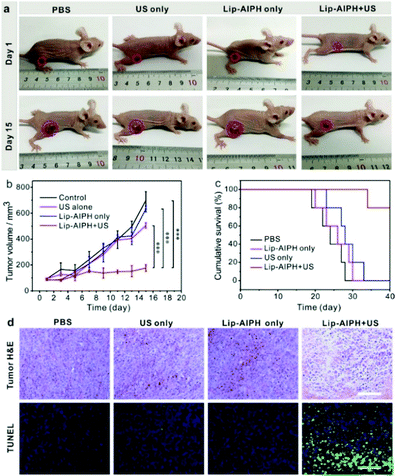 |
| Fig. 7 (a) Photographs of the tumor bearing mice in different treated groups at day 1 and day 15, and the in vivo therapeutic protocol of SDT on MCF-7-tumor-bearing mice. (b) Time-dependent tumor-volume curves of MCF-7-tumor-bearing mice in each experiment group. All data are means ± s.d.; n = 5. (c) Survival curves of MCF-7 tumor-bearing mice after different treatments. (d) Hematoxylin and eosin staining, and TUNEL staining in tumor sections of each experiment group (scale bar: 100 μm). | |
Hematoxylin and eosin staining and TdT-mediated dUPT nick-end labeling (TUNEL) of tumor tissues were conducted to investigate the synergistic mechanism of SDT and gas therapy after various treatments (Fig. 7d). The major organs from MCF-7 tumor-bearing mice of each group, including the heart, liver, spleen, lungs, and kidneys, were analyzed (Fig. S19, ESI†) and no organ damage was observed. Additionally, hematoxylin and eosin staining images of tumors injected with Lip-AIPH plus US irradiation showed a hiatus in the cytoplasm and tissue space along with karyopyknosis and plasmatorrhexis, indicating serious necrocytosis and apoptosis (Fig. 7d). Furthermore, the tumor TUNEL assay results (Fig. 7d) showed that the tumor sections of animals receiving Lip-AIPH and US treatment showed much more intensive green fluorescence, indicating a high level of apoptosis. However, no obvious green fluorescence signals were observed in the control groups. Thus, all of the above results demonstrate the potential of the US activator and Lip-AIPH liposome system in vivo, acting not only as a synergistic SDT and gas therapy but also an US imaging contrast agent.
3. Conclusions
In summary, we have successfully prepared US-activated Lip-AIPH with a remarkable ability for simultaneous US imaging and synergistic SDT and gas cancer therapy. Upon US irradiation, theranostic liposomes were able to produce gas bubbles and free radicals with high efficiency in the absence of oxygen. This novel theranostic nanoplatform possesses a number of advantages, including excellent stability, on-demand release, US-triggered imaging, and synergistic gas and SDT cancer therapy. Moreover, the Lip-AIPH-induced cancer therapy under US irradiation is oxygen independent and can be applied in the treatment of hypoxic and deep tumors, overcoming the limitations of traditional radiotherapy and chemotherapy, which are dependent on appropriate oxygen levels. In the future, we will further functionalize and modify the Lip-AIPH with targeting molecules to enrich tumor tissues by active-targeting. The ability of the Lip-AIPH to enrich the tumor tissues would be further improved. The prepared system is a promising US responsive theranostic nanoplatform with a suitable penetration depth, overcoming the current limitations of therapy in hypoxic tumors and enhancing US imaging.
Conflicts of interest
There are no conflicts to declare.
Acknowledgements
This research was supported by the National Natural Science Foundation of China (No. U1505221, 21475026, 21874024), and the Program for Changjiang Scholars and the Innovative Research Team in University (No. IRT15R11).
References
- S. Mitragotri, Nat. Rev. Drug Discovery, 2005, 4, 255 CrossRef CAS PubMed.
- L. B. Feril and T. Kondo, J. Radiat. Res., 2004, 45, 479–489 CrossRef PubMed.
- J. R. Lindner, Nat. Rev. Drug Discovery, 2004, 3, 527 CrossRef CAS PubMed.
- S. R. Wilson and P. N. Burns, Radiology, 2010, 257, 24–39 CrossRef PubMed.
- E. Jung, C. Kang, J. Lee, D. Yoo, D. W. Hwang, D. Kim, S. C. Park, S. K. Lim, C. Song and D. Lee, ACS Nano, 2018, 12, 392–401 CrossRef CAS PubMed.
- E. Huynh, B. Y. Leung, B. L. Helfield, M. Shakiba, J. A. Gandier, C. S. Jin, E. R. Master, B. C. Wilson, D. E. Goertz and G. Zheng, Nat. Nanotechnol., 2015, 10, 325–332 CrossRef CAS PubMed.
- M. Ma, H. Xu, H. Chen, X. Jia, K. Zhang, Q. Wang, S. Zheng, R. Wu, M. Yao, X. Cai, F. Li and J. Shi, Adv. Mater., 2014, 26, 7378–7385 CrossRef CAS PubMed.
- Y. Li, H. An, X. Wang, P. Wang, F. Qu, Y. Jiao, K. Zhang and Q. Liu, Nano Res., 2017, 11, 1038–1056 CrossRef.
- S. Ibsen, C. E. Schutt and S. Esener, Drug Des., Dev. Ther., 2013, 7, 375 CrossRef CAS PubMed.
- Y. Liu, F. Yang, C. Yuan, M. Li, T. Wang, B. Chen, J. Jin, P. Zhao, J. Tong, S. Luo and N. Gu, ACS Nano, 2017, 11, 1509–1519 CrossRef CAS PubMed.
- W.-P. Li, C.-H. Su, Y.-C. Chang, Y.-J. Lin and C.-S. Yeh, ACS Nano, 2016, 10, 2017–2027 CrossRef CAS PubMed.
- M. Kuroki, K. Hachimine, H. Abe, H. Shibaguchi, M. Kuroki, S.-i. Maekawa, J. Yanagisawa, T. Kinugasa, T. Tanaka and Y. Yamashita, Anticancer Res., 2007, 27, 3673–3677 CAS.
- X. Qian, Y. Zheng and Y. Chen, Adv. Mater., 2016, 28, 8097–8129 CrossRef CAS PubMed.
- L. Song, P. P. Li, W. Yang, X. H. Lin, H. Liang, X. F. Chen, G. Liu, J. Li and H. H. Yang, Adv. Funct. Mater., 2018, 1707496 CrossRef.
- X. H. Lin, L. Song, S. Chen, X. F. Chen, J. J. Wei, J. Li, G. Huang and H. H. Yang, ACS Appl. Mater. Interfaces, 2017, 9, 41181–41187 CrossRef CAS PubMed.
- I. Rosenthal, J. Z. Sostaric and P. Riesz, Ultrason. Sonochem., 2004, 11, 349–363 CAS.
- K. Tachibana, L. B. Feril Jr and Y. Ikeda-Dantsuji, Ultrasonics, 2008, 48, 253–259 CrossRef CAS PubMed.
- M.-F. Chung, K.-J. Chen, H.-F. Liang, Z.-X. Liao, W.-T. Chia, Y. Xia and H.-W. Sung, Angew. Chem., 2012, 124, 10236–10240 CrossRef.
- M. M. Chen, F. F. Song, M. Feng, Y. Liu, Y. Y. Liu, J. Tian, F. Lv and Q. Q. Zhang, Colloids Surf., B, 2018, 167, 104–114 CrossRef CAS PubMed.
- K. Alamoudi, P. Martins, J. G. Croissant, S. Patil, H. Omar and N. M. Khashab, Nanomedicine, 2017, 12, 1421–1433 CrossRef CAS PubMed.
- K. J. Chen, E. Y. Chaung, S. P. Wey, K. J. Lin, F. Cheng, C. C. Lin, H. L. Liu, H. W. Tseng, C. P. Liu and M. C. Wei, ACS Nano, 2014, 8, 5105–5115 CrossRef CAS PubMed.
- A. Y. Rwei, J. L. Paris, B. Wang, W. Wang, C. D. Axon, M. Vallet-Regi, R. Langer and D. S. Kohane, Nat. Biomed. Eng., 2017, 1, 644–653 CrossRef PubMed.
- A. L. Klibanov, Invest. Radiol., 2006, 41, 354–362 CrossRef PubMed.
- S. H. Medina, M. S. Michie, S. E. Miller, M. J. Schnermann and J. P. Schneider, Angew. Chem., Int. Ed., 2017, 56, 11404–11408 CrossRef CAS PubMed.
- K. Zhang, H. Xu, X. Jia, Y. Chen, M. Ma, L. Sun and H. Chen, ACS Nano, 2016, 10, 10816–10828 CrossRef CAS PubMed.
- Z.-G. Gao, H. D. Fain and N. Rapoport, J. Controlled Release, 2005, 102, 203–222 CrossRef CAS PubMed.
- Q. Feng, W. Zhang, X. Yang, Y. Li, Y. Hao, H. Zhang, L. Hou and Z. Zhang, Adv. Healthcare Mater., 2018, 7, 1700957 CrossRef PubMed.
- X. Pan, L. Bai, H. Wang, Q. Wu, H. Wang, S. Liu, B. Xu, X. Shi and H. Liu, Adv. Mater., 2018, 30, e1800180 CrossRef PubMed.
- K. Zhang, H. Xu, H. Chen, X. Jia, S. Zheng, X. Cai, R. Wang, J. Mou, Y. Zheng and J. Shi, Theranostics, 2015, 5, 1291–1302 CrossRef CAS PubMed.
- Y. Ma, X. Li, A. Li, P. Yang, C. Zhang and B. Tang, Angew. Chem., Int. Ed., 2017, 56, 13752–13756 CrossRef CAS PubMed.
- Y. Zhang, F. Wang, C. Liu, Z. Wang, L. Kang, Y. Huang, K. Dong, J. Ren and X. Qu, ACS Nano, 2018, 12, 651–661 CrossRef CAS PubMed.
- C. Yao, W. Wang, P. Wang, M. Zhao, X. Li and F. Zhang, Adv. Mater., 2018, 30, 1704833 CrossRef PubMed.
- Q. Jia, J. Ge, W. Liu, X. Zheng, S. Chen, Y. Wen, H. Zhang and P. Wang, Adv. Mater., 2018, 30, e1706090 CrossRef PubMed.
- J. Chen, H. Luo, Y. Liu, W. Zhang, H. Li, T. Luo, K. Zhang, Y. Zhao and J. Liu, ACS Nano, 2017, 11, 12849–12862 CrossRef CAS PubMed.
- C. A. Cook, K. C. Hahn, J. B. Morrissette-McAlmon and W. L. Grayson, Biomaterials, 2015, 52, 376–384 CrossRef CAS PubMed.
- C. McEwan, J. Owen, E. Stride, C. Fowley, H. Nesbitt, D. Cochrane, C. C. Coussios, M. Borden, N. Nomikou and A. P. McHale, J. Controlled Release, 2015, 203, 51–56 CrossRef CAS PubMed.
- C. McEwan, S. Kamila, J. Owen, H. Nesbitt, B. Callan, M. Borden, N. Nomikou, R. A. Hamoudi, M. A. Taylor and E. Stride, Biomaterials, 2016, 80, 20–32 CrossRef CAS PubMed.
- T. Liu, N. Zhang, Z. Wang, M. Wu, Y. Chen, M. Ma, H. Chen and J. Shi, ACS Nano, 2017, 11, 9093–9102 CrossRef CAS PubMed.
- S. Shen, C. Zhu, D. Huo, M. Yang, J. Xue and Y. Xia, Angew. Chem., Int. Ed., 2017, 56, 8801–8804 CrossRef CAS PubMed.
- C. Li, Y. Zhang, Z. Li, E. Mei, J. Lin, F. Li, C. Chen, X. Qing, L. Hou, L. Xiong, H. Hao, Y. Yang and P. Huang, Adv. Mater., 2018, 30, 1706150 CrossRef PubMed.
- A. Gabizon and D. Papahadjopoulos, Proc. Natl. Acad. Sci. U. S. A., 1988, 85, 6949–6953 CrossRef CAS.
- H. J. Chen, X. R. Huang, X. B. Zhou, B. Y. Zheng and J. D. Huang, Chem. Commun., 2015, 51, 4681–4684 RSC.
- Z.-J. Dai, S. Li, J. Gao, X.-N. Xu, W.-F. Lu, S. Lin and X.-J. Wang, Med. Hypotheses, 2013, 80, 300–302 CrossRef CAS PubMed.
- F. Y. Huang, J. Lei, Y. Sun, F. Yan, B. Chen, L. Zhang, Z. Lu, R. Cao, Y. Y. Lin, C. C. Wang and G. H. Tan, OncoImmunology, 2018, 7, e1446720 CrossRef PubMed.
- Q. Yu, Z. Zhang, J. Sun, Y. Xia, Q. Du and D. Liang, Sci. China: Chem., 2017, 60, 385–395 CrossRef CAS.
- Y. Watanabe, A. Aoi, S. Horie, N. Tomita, S. Mori, H. Morikawa, Y. Matsumura, G. Vassaux and T. Kodama, Cancer Sci., 2008, 99, 2525–2531 CrossRef CAS PubMed.
- J. Wu and W. L. Nyborg, Adv. Drug Delivery Rev., 2008, 60, 1103–1116 CrossRef CAS PubMed.
- E. Kang, H. S. Min, J. Lee, M. H. Han, H. J. Ahn, I. C. Yoon, K. Choi, K. Kim, K. Park and I. C. Kwon, Angew. Chem., Int. Ed., 2010, 49, 524–528 CrossRef CAS PubMed.
- A. Kheirolomoom, P. A. Dayton, A. F. Lum, E. Little, E. E. Paoli, H. Zheng and K. W. Ferrara, J. Controlled Release, 2007, 118, 275–284 CrossRef CAS PubMed.
- X. Q. Wang, F. Gao and X. Z. Zhang, Angew. Chem., Int. Ed., 2017, 56, 9029–9033 CrossRef CAS PubMed.
- W. Gao, S. Li, Z. Liu, Y. Sun, W. Cao, L. Tong, G. Cui and B. Tang, Biomaterials, 2017, 139, 1–11 CrossRef CAS PubMed.
- W. L. Wan, Y. J. Lin, H. L. Chen, C. C. Huang, P. C. Shih, Y. R. Bow, W. T. Chia and H. W. Sung, J. Am. Chem. Soc., 2017, 139, 12923–12926 CrossRef CAS PubMed.
- Y. Yoshida, N. Itoh, Y. Saito, M. Hayakawa and E. Niki, Free Radical Res., 2009, 38, 375–384 CrossRef.
- A. Piermattei, S. Karthikeyan and R. P. Sijbesma, Nat. Chem., 2009, 1, 133–137 CrossRef CAS PubMed.
- H. Mohapatra, M. Kleiman and A. P. Esser-Kahn, Nat. Chem., 2016, 9, 135–139 CrossRef.
- V. MišÍk and P. Riesz, Ann. N. Y. Acad. Sci., 2000, 899, 335–348 CrossRef.
- H. Maeda, J. Wu, T. Sawa, Y. Matsumura and K. Hori, J. Am. Chem. Soc., 2000, 65(1–2), 271–284 CAS.
- L. S. Lin, X. Yang, Z. Zhou, Z. Yang, O. Jacobson, Y. Liu, A. Yang, G. Niu, J. Song, H. H. Yang and X. Chen, Adv. Mater., 2017, 29, 1606681 CrossRef PubMed.
Footnote |
† Electronic supplementary information (ESI) available: TEM images, counting statistics and DLS measurements of the hybrid systems and corresponding reference samples. See DOI: 10.1039/c8nh00340h |
|
This journal is © The Royal Society of Chemistry 2019 |