DOI:
10.1039/C8NA00233A
(Paper)
Nanoscale Adv., 2019,
1, 772-780
Rh-doped MoSe2 as a toxic gas scavenger: a first-principles study
Received
22nd September 2018
, Accepted 9th November 2018
First published on 9th November 2018
Abstract
Using first-principles theory, we investigated the most stable configuration for the Rh dopant on a MoSe2 monolayer, and the interaction of the Rh-doped MoSe2 (Rh-MoSe2) monolayer with four toxic gases (CO, NO, NO2 and SO2) to exploit the potential application of the Rh-MoS2 monolayer as a gas sensor or adsorbent. Based on adsorption behavior comparison with other 2D adsorbents and desorption behavior analysis, we assume that the Rh-MoSe2 monolayer is a desirable adsorbent for CO, NO and NO2 storage or removal given the larger adsorption energy (Ead) of −2.00, −2.56 and −1.88 eV, respectively, compared with other materials. In the meanwhile, the Rh-MoSe2 monolayer is a good sensing material for SO2 detection according to its desirable adsorption and desorption behaviors towards the target molecule. Our theoretical calculation would provide a first insight into the TM-doping effect on the structural and electronic properties of the MoSe2 monolayer, and shed light on the application of Rh-MoSe2 for the sensing or disposal of common toxic gases.
1. Introduction
Sensing toxic gases, especially detection of the ones at sub-ppm levels, is quite imperative with respect to environmental standards and agricultural pollution monitoring. In this regard, researchers have always contributed to finding novel sensing materials for potential application as chemical sensors with a rapid response, high sensitivity and low cost. In the past few decades, semiconducting metal oxide nanowire,1–3 carbon nanotube,4–6 and graphene7–9 based sensors have successively caught the attention of researchers, arousing considerable interest from the research community. Nevertheless, they are never satisfied by these achievements, persisting to pursue some new materials that possess more fascinating sensing behavior than the previous ones.
After the successful application of graphene as a gas sensor, scholars have turned their attention to two-dimensional (2D) materials that have large surface–volume ratios and tunable electronic properties due to their unique structural configuration. Materials such as hexagonal boron nitride,10 antimonene,11 phosphorene12 and silicene13 have become the focus of the sensing field, in order to find candidate materials having the advantages of graphene such as high carrier mobility and strong chemical activity for gas interaction,14,15 as well as semiconducting properties. In the meanwhile, group III–V nitrides, particularly AlN and InN,16,17 have been regarded as promising structures for gas sensing,18,19 and the experimental breakthrough in the synthesis of InN20 makes it possible to be used as a substitute for graphene with inherent bandgap characteristics.21
Very recently, 2D transition metal dichalcogenides (TMDs), especially MoS2 monolayers,22–24 have attracted much attention as alternative materials to conventional metal oxides for chemical sensing devices. Moreover, surface doping with transition metal (TM) atoms has been demonstrated to provide a monolayer with enhanced adsorption and sensing performance for gas molecules25–28 due to the improved chemical activity and electron mobility induced by the TM dopant,29 opening up a novel insight into the sensor family. Other than that, MoSe2, as a new emerging semiconducting material, has been investigated as well for its application as a sensor. While Late et al. first reported the high sensing performance of the MoSe2 monolayer for ppm-level NH3 gas,30 Baek et al. developed a MoSe2 multilayer based field-effect transistor for NO2 detection.31 However, the potential applications of MoSe2 based materials need to be further explored and broadened after their successful synthesis by chemical vapor deposition.32 This inspires us to implement a first-principles calculation to study the adsorption performance of TM-MoSe2 monolayers towards four industrial exhaust gases including CO, NO, NO2 and SO2 to put forward a novel material for toxic gas sensing. Among numerous TM atoms, rhodium (Rh) is the one with strong electron mobility and catalytic performance for gas interaction, previously demonstrated by carbon nanotube,33 graphene,34 and MoS2 monolayer systems35 where Rh was proposed as a dopant to functionalize the proposed surfaces. We assumed that it would be interesting and necessary to investigate the adsorption and sensing behaviors of the Rh-doped MoSe2 (Rh-MoSe2) monolayer towards toxic gases to exploit the novel material for toxic gas detection or removal. The results indicate that the Rh-MoSe2 monolayer possesses quite strong adsorption behavior towards CO, NO and NO2 molecules that gives rise to chemisorption in these systems, while physisorption could be determined for the Rh-MoSe2/SO2 system. Through adsorption behavior comparison with other 2D adsorbents and desorption behavior analysis, we assume that Rh-MoSe2 is a desirable adsorbent for CO, NO and NO2 storage or removal while being a good sensing material for SO2 detection. To the best of our knowledge, this would be the first report investigating the potential application of Rh-MoSe2 for the removal of four toxic species in a theoretical manner.
2. Computational details
In this work, spin-polarized calculations were implemented in the Dmol3 package36 of Materials Studio. The Perdew–Burke–Ernzerhof (PBE) functional with a generalized gradient approximation (GGA) was employed to deal with the electron exchange and correlation,37 and to obtain the optimized structures. The Grimme method was employed38 for better understanding the van der Waals interaction. We selected double numerical plus polarization (DNP) as the atomic orbital basis set,26 while the DFT semi-core pseudopotential (DSSP) method was employed to dissolve the relativistic effect of the TM atom.39 The k-point sample of the Monkhorst–Pack grid was sampled to 5 × 5 × 1 of the Brillouin zone for geometry optimization and to 7 × 7 × 1 for electronic structure calculations.40 The energy tolerance accuracy, maximum force, and displacement were selected as 10−5 Ha, 2 × 10−3 Ha Å−1, and 5 × 10−3 Å,41 respectively. For static electronic structure calculations, a self-consistent loop energy of 10−6 Ha, global orbital cut-off radius of 5.0 Å and smearing of 0.005 Ha were employed to ensure the accurate results of total energy.42 For basis set superposition errors (BSSE), little impact could be caused in the Dmol3 package,43 and thus we would not analyze it in the following part.
A 4 × 4 × 1 MoSe2 monolayer supercell including 16 Mo and 32 Se atoms with a vacuum region of 15 Å was established and relaxed to its most stable configuration. A previous report has proved that a 4 × 4 supercell would be large enough to conduct the gas adsorption process while a 15 Å slab would be appropriate to prevent the interaction between adjacent units.44 The lattice constant calculated here was 3.30 Å, which is in agreement with other theoretical studies (3.31 Å (ref. 45)).
The adsorption energy (Ead) of each gas adsorption process was calculated by the following equation:23
| Ead = ERh-MoSe2/gas − ERh-MoSe2 − Egas | (1) |
where the
ERh-MoSe2/gas,
ERh-MoSe2 and
Egas represent energies of the adsorbed system, isolated Rh-MoSe
2 and gas molecule, respectively. To analyze the charge transfer (
QT) between the target molecule and adsorbent surface, the Mulliken population analysis was considered, characterized by the carried electron value by gas molecules after adsorption. Only the most favorable configuration for gas adsorption would be plotted and analyzed in the following parts.
3. Results and discussion
3.1 Geometric and electronic structure of Rh-MoSe2
We determined Rh atom adsorption onto a bare MoSe2 monolayer Rh-MoSe2, where four possible sites were considered, traced as TH (above the center of the hexagonal ring of MoSe2), TMo (at the top of the Mo atom), TSe (at the top of the Se atom) and BS–S (the bridge site between two Se atoms), respectively. The binding energy (Eb) for Rh adsorption onto the most favorable doping site is determined through the formula: | Ed = ERh-MoSe2 − ERh − EMoSe2 | (2) |
where ERh-MoSe2, ERh, and EMoSe2 represent the energies of the Rh-MoSe2, Rh atom and pure MoSe2, respectively.
After optimization, the most stable configuration of Rh-MoSe2 in line with relevant deformation charge density (DCD) is shown in Fig. 1. One can see that the Rh atom tends to be adsorbed onto the MoSe2 monolayer through the TMo site, with three Rh–Se bond lengths of 2.383, 2.383 and 2.354 Å, respectively. The geometry of MoSe2 undergoes a little deformation after Rh doping due to the binding force of Rh–Se bonds.46 The QT between the Rh dopant and the MoSe2 monolayer is found to be −0.239 e, indicating that the Rh dopant acts as an electron acceptor while the MoSe2 monolayer acts as a donor. This is in line with the strong electron withdrawing properties of the Rh atom,33 thereby leading to high electron localization around the Rh atom. In addition, we can find that the Mo atoms are mainly the electron accepting centers while the Se atoms are the electron donating centers for the optimized Rh-MoSe2 system.
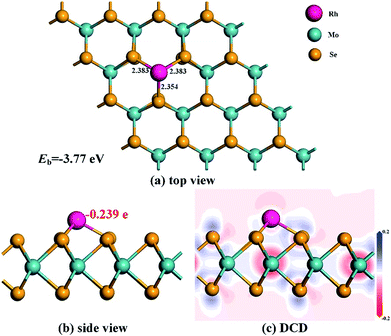 |
| Fig. 1 Geometric structures (a) and (b) and DCD (c) of the Rh-MoSe2 monolayer. The black values are the atom-to-atom distance while the red value is the electron amount carried by Rh dopant. | |
To further elucidate the electronic behavior of the Rh doping effect on the MoSe2 monolayer compared with its pure counterpart, density of states (DOS) analysis is performed, as depicted in Fig. 2. It can be seen in the total DOS distribution of Fig. 2(a) that there is a big band gap near the Fermi level in the DOS curve of intrinsic MoSe2, confirming its semiconducting properties well. With the doping of the Rh atom, the gap gets narrowed obviously due to the upward shifted Fermi level which was originally identified as the valence band maximum in the Dmol3 package, caused by the Rh contribution. It is interesting to note that the DOS spin up and down of the Rh-MoSe2 monolayer are shown to be asymmetric in comparison with those in the pure MoSe2 system that shows good symmetry, which could be ascribed to the total magnetic moment of 1.0 μB in this system induced by the Rh dopant. Moreover, due to the electron-donating behavior of the MoSe2 monolayer that results in an improved effective coulombic potential,47 the DOS curve of the Rh-MoSe2 system is found to left shift towards a lower region consequently compared with that of its intrinsic counterpart. In Fig. 1(b), the DOS of the Rh 4d orbital is largely overlapped with that of the Se 4p orbital, demonstrating strong hybridization of the Rh atom onto the MoSe2 monolayer. Apart from that, it reveals that the highest occupied molecular orbitals (HOMOs) are mainly located at the Se atom while the lowest unoccupied molecular orbitals are at the Rh atom, which confirms the results of DCD that charge is accumulated around the Rh dopant.48
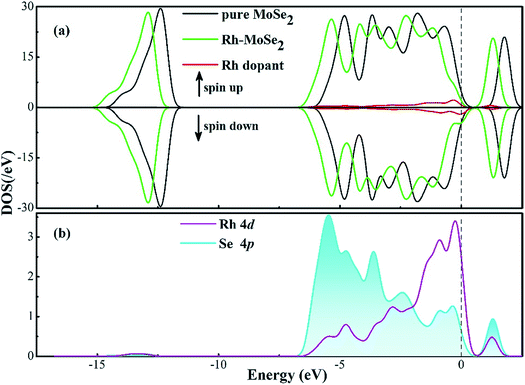 |
| Fig. 2 DOS distribution of Rh-MoSe2. The dashed line is the Fermi level. | |
3.2 CO adsorption
Fig. 3 shows the most stable configuration and related DCD for CO adsorption on the Rh-MoSe2 monolayer. One can see that the C atom is trapped by the Rh dopant with a Rh–C bond length of 1.892 Å after the CO molecule is adsorbed on the surface. It would be worth noting that such a value of atom-to-atom distance is even shorter than the sum of the corresponding covalent radii (2.00 Å for Rh–C49) indicating some chemisorption in this system.50 In fact, the large enough Ead of −2.00 eV here could not only confirm the strong adsorption performance of Rh-MoSe2 towards the CO molecule, but also indicate its chemical nature with strong spontaneity for this interaction. In the meanwhile, three Rh–Se bonds, after CO adsorption, are elongated to 2.432, 2.448 and 2.399 Å, respectively, and the C–O bond of the CO molecule is prolonged to 1.164 Å from 1.142 Å in an isolated molecule. These findings suggest the activation behavior for the CO molecule when interacted with the monolayer. The DCD shows that the Rh-MoSe2 monolayer acting as an electron acceptor withdraws 0.131 e from the adsorbed CO molecule. This, combined with the Mulliken population analysis for the Rh dopant that carries 0.467 e after adsorption, indicates that the Rh dopant is an electron localization center accepting charge from both gas molecules and the MoSe2 surface.
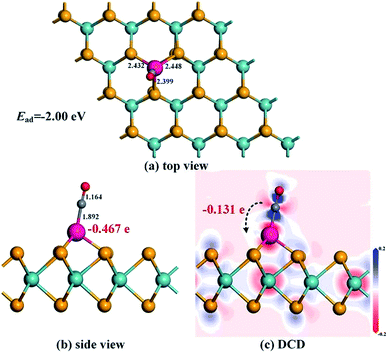 |
| Fig. 3 Adsorption configuration (a) and (b) and DCD (c) of the Rh-MoSe2/CO system. | |
Fig. 4 shows the total and partial DOS distributions for the CO adsorption system. Based on the comparison between the total DOS for the pure Rh-MoSe2 system and the one for the adsorbed system, we can find that they are basically overlapped except for the area near the Fermi levels where the Rh dopant contributes a lot due to its strong electron activity, and the areas at 6.5 and 8.5 eV where the adsorbed CO molecule makes a great contribution for the DOS of the whole system. Besides, the deformations for the DOS between the isolated CO molecule and the adsorbed one confirm that the CO molecule is activated during gas adsorption. Moreover, the large overlaps between DOS of Rh 4d and C 2p orbitals manifest strong hybridization between Rh and C atoms, which would explain the strong binding force of the Rh–C bond that leads to the short atom-to-atom distance.
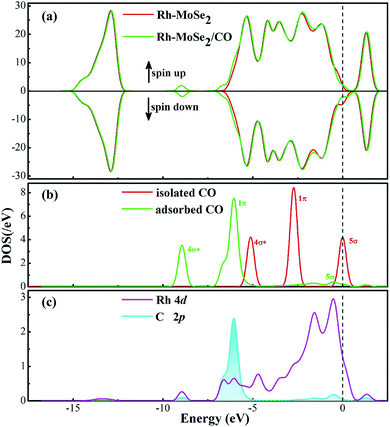 |
| Fig. 4 DOS distribution of the Rh-MoSe2/CO system. The dashed line is the Fermi level. In the gas DOS, the red value shows the orbitals of isolated molecules while the green one shows the orbitals of adsorbed molecules. | |
3.3 NO adsorption
The most stable adsorption configuration and related DCD for the Rh-MoSe2/NO system are exhibited in Fig. 5. One can observe that the structure for NO adsorption onto the Rh-MoSe2 monolayer, with N atoms captured, is somewhat similar to that of the CO system. However, the largely elongated Rh–Se bonds with lengths of 2.445, 2.453 and 2.449 Å in the NO system suggest a stronger binding force of Rh on the NO molecule than the CO molecule. Similarly, the elongated N–O bond of 1.178 Å in the adsorbed NO molecule in comparison with its isolated counterpart (1.164 Å) indicates the activation behavior of the NO molecule for interaction with the Rh-MoSe2 monolayer.35 The shorter length of the Rh–N bond (1.911 Å) compared with the sum of relevant covalent radii (1.96 Å for Rh–N49) shows the nature of chemisorption. In fact, all these results could be well supported by the large Ead of −2.56 eV and QT of −0.322 e calculated in this system as they are large enough to confirm the strong interaction between gas molecules and the adsorbent surface.51
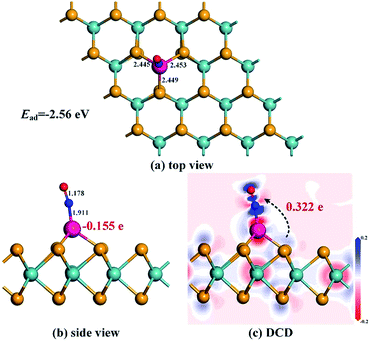 |
| Fig. 5 Adsorption configuration (a) and (b) and DCD (c) of the Rh-MoSe2/NO system. | |
From the DOS distribution for the NO system as portrayed in Fig. 6, the electronic behavior for NO adsorption on the Rh-MoSe2 monolayer could be elucidated clearly. In the total DOS distribution, we can see that the DOS of the NO system transforms slightly above the Fermi level compared with that of the pure Rh-MoSe2 system. In detail, two novel peaks appear at around −7.5 and −8.5 eV while one peak at the Fermi level disappears after gas adsorption, which could be attributed to the DOS deformation of the adsorbed NO molecule. It could be seen that the DOS peak of the isolated NO molecule at the Fermi level splits into two small peaks after NO adsorption that weakens the contribution to the total DOS of the whole system, while the slightly weakened peak at −8.5 eV and the enhanced peak at −7.5 eV accord with the novel peaks in DOS for the adsorbed system. Based on the partial DOS analysis, we could see that every peak of the N 2p orbital is overlapped with that of the Rh 4d orbital, implying that the N atom is strongly hybridized with the Rh dopant, thus giving rise to a strong binding force for the Rh–N bond.
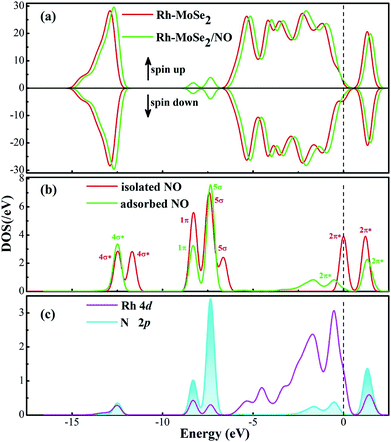 |
| Fig. 6 DOS distribution of the Rh-MoSe2/NO system. The dashed line is the Fermi level. In the gas DOS, the red value shows the orbitals of isolated molecules while the green one shows the orbitals of adsorbed molecules. | |
3.4 NO2 adsorption
In terms of NO2 adsorption onto the Rh-MoSe2 monolayer, one can see from Fig. 7 that the NO2 molecule prefers to adsorb two O atoms trapped with a shorter Rh–O distance of 2.113 Å. Three Rh–Se bonds of Rh-MoSe2 in line with the N–O bond of the NO2 molecule undergo some elongation after adsorption, due to the binding force of Rh on the NO2 molecule. The Mulliken population analysis indicates that 0.361 e transfers from the Rh-MoSe2 monolayer to the NO2 molecule with an electron loss of 0.086 for the Rh dopant. That is to say, unlike in the CO system, the Rh dopant acts as an electron donor releasing the electron to the gas molecule, which could be found in NO and SO2 systems as well. This finding manifests the strong electron mobility and chemical activity of TM when interacting with gas molecules.52 Moreover, the large QT associated with the relatively large Ead suggests the kind of chemisorption for the Rh-MoSe2/NO2 system.
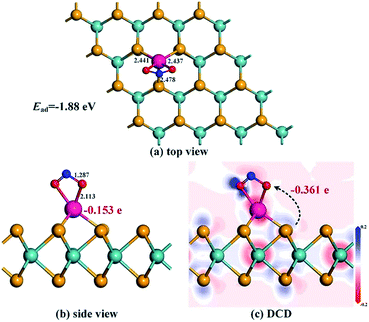 |
| Fig. 7 Adsorption configuration (a) and (b) and DCD (c) of the Rh-MoSe2/NO2 system. | |
Fig. 8 shows the DOS distributions for the NO2 adsorption system. One can find from the total DOS configurations that the DOS curve transforms dramatically around the Fermi level after adsorption of the NO2 molecule. This may be attributed to the considerable electron-transfer between gas molecules and the adsorbent surface that results in electron redistribution for the whole system, thus leading to the deformation of electronic states at the Fermi level.53 Furthermore, the novel emerged peaks at −7.5, −8.5 and −11.5 eV are contributed by the adsorbed NO2 molecule which is activated during adsorption. Similarly, the hybridization between Rh and O atoms is identified through the phenomenon of overlaps between Rh 4d and O 2p orbitals.
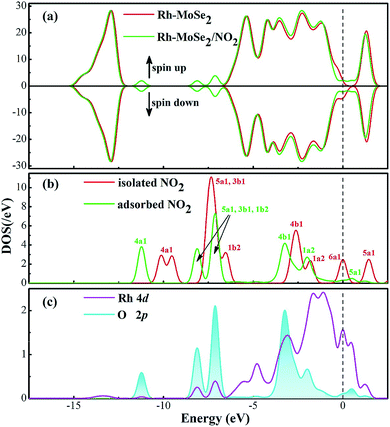 |
| Fig. 8 DOS distribution of the Rh-MoSe2/NO2 system. The dashed line is the Fermi level. In the gas DOS, the red value shows the orbitals of isolated molecules while the green one shows the orbitals of adsorbed molecules. | |
3.5 SO2 adsorption
From Fig. 9 where the most stable configuration for SO2 adsorption on the Rh-MoSe2 monolayer is displayed, we can see that, similar to the NO2 adsorption configuration, the SO2 molecule is adsorbed on the Rh-MoSe2 monolayer with two O atoms oriented. However, the SO2 molecule here stands a little far from the Rh dopant, with the nearest atom-to-atom distance measured to be 2.275 Å, which indicates a weak interaction in the Rh-MoSe2/SO2 system. The tiny prolongation for Rh–Se bonds inner Rh-MoSe2 to 2.423, 2.404 and 2.392 Å, along with S–O bond inner SO2 to 1.550 Å by 0.019 Å can confirm the weak binding force between the Rh dopant and the SO2 molecule as well. The Ead obtained here is −0.89 eV and the Mulliken population analysis shows a QT of 0.285 e from the Rh-MoSe2 monolayer to the SO2 molecule. All these findings suggest physisorption for SO2 adsorption on the Rh-MoSe2 monolayer.51
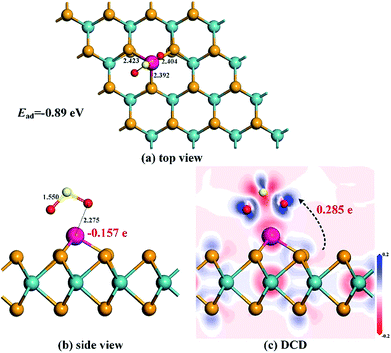 |
| Fig. 9 Adsorption configuration (a) and (b) and DCD (c) of the Rh-MoSe2/SO2 system. | |
DOS distributions shown in Fig. 10 could give a clear explanation for the electronic behavior in this system. The novel peaks around −11.5 and −7 and the Fermi level in total DOS of the adsorbed system are from the contribution of the adsorbed SO2 molecule that is somewhat activated after adsorption. At the same time, the overlaps between DOS of Rh 4d and O 2p orbitals, although not as large as the NO2 system, prove the orbital interaction, to some extent, between the Rh dopant and the SO2 molecule.
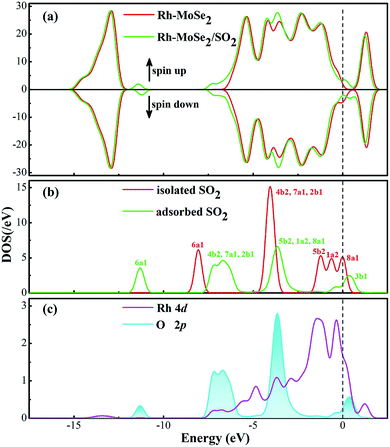 |
| Fig. 10 DOS distribution of the Rh-MoSe2/SO2 system. The dashed line is the Fermi level. | |
3.6 Application of Rh-MoSe2 to toxic gas scavenging
We first investigated the potential application of Rh-MoSe2 as a resistance-type sensor for these gases. According to our previous analysis, it could be found that the Rh-MoSe2 monolayer has quite strong adsorption performance for three species namely CO, NO and NO2 molecules, giving rise to chemisorption in these systems, while having relatively weaker performance towards SO2 adsorption that gives rise to physisorption instead. In other words, it would be difficult for these three gas molecules to desorb from the Rh-MoSe2 monolayer once they are adsorbed onto the surface, except for the SO2 molecule which may be desorbed through annealing at high temperature or irradiation with ultraviolet light.50,54
To confirm this assumption, the recovery time (τ) analysis based on transition state theory and van't-Hoff–Arrhenius expression55 was implemented, and expressed as:
where
A is the attempt frequency determined as 10
12 s
−1 according to a previous report,
56T is the temperature and
KB is the Boltzmann constant (8.318 × 10
−3 kJ (mol K)
−1). Given the inverse processes between adsorption and desorption, we assume the value of
Ead as the potential barrier (
Ea) for desorption. It would be obvious that a larger
Ead would lead to a harder process for gas desorption, and the increase of temperature can accelerate that process effectively. According to the obtained
Ead in our calculations as shown in
Table 1, we plotted the recovery time for the desorption of various gases at various temperatures as portrayed in
Fig. 11. One can conclude that CO, NO and NO
2 desorption from the Rh-MoSe
2 monolayer would be extremely unrealistic at room temperature, and even at 798 K, it would take more than 4 hours for NO desorption from the surface. Although remarkably enhanced behavior for CO and NO
2 desorption could be achieved at 798 K, the considerable heat loss and the safety of the devices would be another issue. Therefore, it would be inappropriate to use the Rh-MoSe
2 monolayer as the sensing material for CO, NO or NO
2 detection, because the one-off operation for gas sensors would be a waste of money and reduce work-efficiency. On the other hand, we assume that Rh-MoSe
2 is suitable for SO
2 sensing given its good adsorption performance for detection and desorption performance with appropriate recovery time at ambient temperature for recycle use. Moreover, the sensing mechanism of the Rh-MoSe
2 monolayer towards SO
2 would be dependent on the increased conductivity due to the narrowed energy gap from 1.033 eV for the isolated Rh-MoSe
2 monolayer to 0.227 eV for the SO
2 system
57 as seen in
Table 1.
Table 1 Adsorption parameters for various Rh-MoSe2/gas systems
Gas systems |
E
ad (eV) |
D (Å) |
Q
T (e) |
E
g (eV) |
CO |
−2.00 |
1.892 |
−0.131 |
0.202 |
NO |
−2.56 |
1.911 |
0.322 |
1.210 |
NO2 |
−1.88 |
2.113 |
−0.361 |
0.520 |
SO2 |
−0.89 |
2.275 |
0.285 |
0.227 |
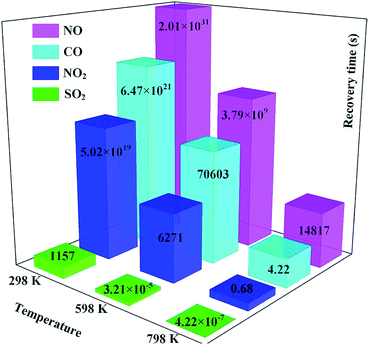 |
| Fig. 11 Recovery time for four species at various temperatures. | |
Second, the large Ead in CO, NO and NO2 systems allows the excellent performance of Rh-MoSe2 as a gas adsorbent for their storage or removal from specific environments. For this purpose, we summarize in Fig. 12 the calculated Ead values of recently reported 2D adsorbents for four gases, in order to find out whether Rh-MoSe2 could be an alternative for scavenging toxic gases. From the figure, we can find that the Rh-MoSe2 monolayer possesses better adsorption performance for CO, NO and NO2 molecules compared with the pure MoS2 monolayer,23 the pure MoSe2 monolayer,58 pure InN,19 pure C3N,57 pure penta-graphene,59 and some other TM-doped MoS2 monolayers.22,40,60,61 That is to say, the Rh-MoSe2 monolayer has strong potential to be an adsorbent candidate for CO, NO or NO2 storage and removal. Conversely, it is not a desirable SO2 adsorbent due to its weaker performance than pure InN,19 penta-graphene,59 the Au-MoS2 monolayer,40 the Pt-MoS2 monolayer40 and the Ni-MoS2 monolayer,60 although it has better performance than the pure MoS2 monolayer,23 the pure MoSe2 monolayer58 and pure C3N,57 except for the Cu-MoS2 monolayer which is not studied for SO2 adsorption in ref. 22 and 61. In addition, we would introduce the application of borophene as a potential SO2 adsorbent given its strong ability and capacity whereby up to seven SO2 molecules could be chemisorbed on its one side, with a weight percentage of 82.88%, for SO2 adsorption.62
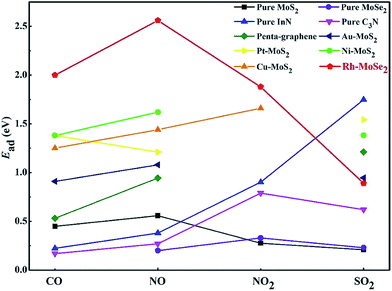 |
| Fig. 12
E
ad comparison of Rh-MoSe2 for four species with recent reports. | |
4. Conclusions
In this work, we implemented a first-principles theory to study the adsorption performance of the Rh-MoSe2 monolayer towards four toxic gases, including CO, NO, NO2 and SO2. Desorption behavior analysis and adsorption behavior comparison with other 2D materials towards these four species were conducted in order to exploit the potential application of our proposed monolayer. The results indicated that the Rh-MoSe2 monolayer possesses quite strong adsorption behavior towards CO, NO and NO2 molecules that gives rise to chemisorption in these systems, while physisorption could be determined for the Rh-MoSe2/SO2 system. In that regard, based on the adsorption behavior comparison with other adsorbents and desorption behavior analysis, we assume that Rh-MoSe2 is a desirable adsorbent for CO, NO and NO2 storage or removal while it is a good sensing material for SO2 detection. Our theoretical calculation would provide a first insight into the TM-doping effect on the structural and electronic properties of the MoSe2 monolayer, and shed light on the application of Rh-MoSe2 for the sensing or disposal of common toxic gases.
Author contributions
Xiaoxing Zhang conceived and designed the research, Hao Cui performed the research and wrote this manuscript while Guozhi Zhang and Ju Tang helped analyze the data.
Conflicts of interest
There are no conflicts to declare.
Acknowledgements
We acknowledge the financial support from the Fundamental Research Funds for the Central University (No. 2018CDYJSY0055), the China Scholarship Council (No. 201806050143) and the Natural Science Foundation of China (No. 51537009).
References
- X. Li, Z. Gu, J. H. Cho, H. Sun and P. Kurup, Tin–copper mixed metal oxide nanowires: synthesis and sensor response to chemical vapors, Sens. Actuators, B, 2011, 158(1), 199–207 CrossRef CAS.
- H. S. Woo, W. N. Chan and J. H. Lee, Design of Highly Selective Gas Sensors via Physicochemical Modification of Oxide Nanowires: Overview, Sensors, 2016, 16(9), 1531 CrossRef PubMed.
- C. Sun, G. Maduraiveeran and P. Dutta, Nitric oxide sensors using combination of p- and n-type semiconducting oxides and its application for detecting NO in human breath, Sens. Actuators, B, 2013, 186(18), 117–125 CrossRef CAS.
- M. Mittal and A. Kumar, Carbon nanotube (CNT) gas sensors for emissions from fossil fuel burning, Sens. Actuators, B, 2014, 203, 349–362 CrossRef CAS.
- T. Zhang, S. Mubeen, N. V. Myung and M. A. Deshusses, Recent progress in carbon nanotube-based gas sensors, Nanotechnology, 2008, 19(33), 332001 CrossRef PubMed.
- C. Gao, Z. Guo, J. H. Liu and X. J. Huang, The new age of carbon nanotubes: an updated review of functionalized carbon nanotubes in electrochemical sensors, Nanoscale, 2012, 4(6), 1948–1963 RSC.
- Y. Tang, W. Chen, C. Li, L. Pan, X. Dai and D. Ma, Adsorption behavior of Co anchored on graphene sheets toward NO, SO2, NH3, CO and HCN molecules, Appl. Surf. Sci., 2015, 342, 191–199 CrossRef CAS.
- M. Zhou, Y. H. Lu, Y. Q. Cai, C. Zhang and Y. P. Feng, Adsorption of gas molecules on transition metal embedded graphene: a search for high-performance graphene-based catalysts and gas sensors, Nanotechnology, 2011, 22(38), 385502 CrossRef PubMed.
- Y. Liu, X. Dong and P. Chen, ChemInform Abstract: Biological and Chemical Sensors Based on Graphene Materials, Chem. Soc. Rev., 2012, 41(6), 2283–2307 RSC.
- T. A. Abtew, W. Gao, X. Gao, Y. Y. Sun, S. B. Zhang and P. Zhang, Theory of Oxygen-Boron Vacancy Defect in Cubic Boron Nitride: A Diamond NV− Isoelectronic Center, Phys. Rev. Lett., 2014, 113(13), 136401 CrossRef PubMed.
- S. Zhang, Z. Yan, Y. Li, Z. Chen and H. Zeng, Atomically thin arsenene and antimonene: semimetal–semiconductor and indirect–direct band-gap transitions, Angew. Chem., 2015, 54(10), 3112 CrossRef CAS PubMed.
- S. Zhang, S. Guo, Z. Chen, Y. Wang, H. Gao, J. Gómez-Herrero, P. Ares, F. Zamora, Z. Zhu and H. Zeng, Recent progress in 2D group-VA semiconductors: from theory to experiment, Chem. Soc. Rev., 2018, 47(3), 982–1021 RSC.
- H. F. Dai, P. Xiao and Q. Lou, Application of SnO2/MWCNTs nanocomposite for SF6 decomposition gas sensor, Phys. Status Solidi, 2011, 208(7), 1714–1717 CrossRef CAS.
- S. V. Morozov, K. S. Novoselov, M. I. Katsnelson, F. Schedin, D. C. Elias, J. A. Jaszczak and A. K. Geim, Giant intrinsic carrier mobilities in graphene and its bilayer, Phys. Rev. Lett., 2008, 100(1), 016602 CrossRef CAS PubMed.
- Y. H. Zhang, Y. B. Chen, K. G. Zhou, C. H. Liu, J. Zeng, H. L. Zhang and Y. Peng, Improving gas sensing properties of graphene by introducing dopants and defects: a first-principles study, Nanotechnology, 2009, 20(18), 185504 CrossRef PubMed.
- C. Bungaro, K. Rapcewicz and J. Bernholc,
Ab initio phonon dispersions of wurtzite AlN, GaN, and InN, Phys. Rev. B: Condens. Matter Mater. Phys., 2000, 61(10), 6720–6725 CrossRef CAS.
- C. Stampfl, C. G. de Walle Van, D. Vogel, P. Krüger and J. Pollmann, Native defects and impurities in InN: first-principles studies
using the local-density approximation and self-interaction and relaxation-corrected pseudopotentials, Phys. Rev. B: Condens. Matter Mater. Phys., 2000, 61(12), 52–56 CrossRef.
- S. F. Rastegar, A. A. Peyghan, H. R. Ghenaatian and N. L. Hadipour, NO2 detection by nanosized AlN sheet in the presence of NH3: DFT studies, Appl. Surf. Sci., 2013, 274(5), 217–220 CrossRef CAS.
- X. Sun, Q. Yang, R. Meng, C. Tan, Q. Liang, J. Jiang, H. Ye and X. Chen, Adsorption of gas molecules on graphene-like InN monolayer: a first-principle study, Appl. Surf. Sci., 2017, 404, 291–299 CrossRef CAS.
- H. Y. Xu, Z. Liu, X. T. Zhang and S. K. Hark, Synthesis and optical properties of InN nanowires and nanotubes, Appl. Phys. Lett., 2007, 90(11), R1 CrossRef.
- C. E. P. Villegas and A. R. Rocha, Elucidating the Optical Properties of Novel Heterolayered Materials Based on MoTe2–InN for Photovoltaic Applications, J. Phys. Chem. C, 2015, 119(21), 150513134853008 CrossRef.
- S. Archana, Anu, M. Shahid Khan, M. Husain, Md. Shahzad Khan and A. Srivastava, Sensing of CO and NO on Cu-doped MoS2 Monolayer Based Single Electron Transistor: A First Principles Study, IEEE Sens. J., 2018,(99), 1 Search PubMed.
- A. Shokri and N. Salami, Gas sensor based on MoS2 monolayer, Sens. Actuators, B, 2016, 236, 378–385 CrossRef CAS.
- D. Ma, Q. Wang, T. Li, C. He, B. Ma, Y. Tang, Z. Lu and Z. Yang, Repairing sulfur vacancies in the MoS2 monolayer by using CO, NO and NO2 molecules, J. Mater. Chem. C, 2016, 4(29), 7093–7101 RSC.
- D. Ma, W. Ju, T. Li, X. Zhang, C. He, B. Ma, Z. Lu and Z. Yang, The adsorption of CO and NO on the MoS2 monolayer doped with Au, Pt, Pd, or Ni: a first-principles study, Appl. Surf. Sci., 2016, 383, 98–105 CrossRef CAS.
- D. Zhang, J. Wu, L. Peng and Y. Cao, Room-temperature SO2 gas-sensing properties based on a metal-doped MoS2 nanoflower: an experimental and density functional theory investigation, J. Mater. Chem. A, 2017, 5(39), 20666–20677 RSC.
- L. Zhansheng, P. Lv, D. Ma, X. Yang, S. Li and Z. Yang, Detection of gas molecules on single Mn adatom adsorbed graphyne: a DFT-D study, J. Phys. D: Appl. Phys., 2018, 51(6), 065109 CrossRef.
- D. Ma, W. Ju, T. Li, G. Yang, C. He, B. Ma, Y. Tang, Z. Lu and Z. Yang, Formaldehyde molecule adsorption on the doped monolayer MoS2: a first-principles study, Appl. Surf. Sci., 2016, 371, 180–188 CrossRef CAS.
- D. Ma, W. Ju, T. Li, X. Zhang, C. He, B. Ma, Y. Tang, Z. Lu and Z. Yang, Modulating electronic, magnetic and chemical properties of MoS2 monolayer sheets by substitutional doping with transition metals, Appl. Surf. Sci., 2016, 364, 181–189 CrossRef CAS.
- D. J. Late, T. Doneux and M. Bougouma, Single-layer MoSe2 based NH3 gas sensor, Appl. Phys. Lett., 2014, 105(23), 25 CrossRef.
- J. Baek, D. Yin, N. Liu, I. Omkaram, C. Jung, I. Healin, S. Hong, S. M. Kim, Y. K. Hong, J. Hur, Y. Yoon and S. Kim, A highly sensitive chemical gas detecting transistor based on highly crystalline CVD-grown MoSe2 films, Nano Res., 2017, 10(8), 2904 CrossRef CAS.
- S. Y. Choi, Y. Kim, H. S. Chung, A. R. Kim, J. D. Kwon, J. Park, Y. L. Kim, S. H. Kwon, M. G. Hahm and B. Cho, Effect of Nb Doping on Chemical Sensing Performance of Two-Dimensional Layered MoSe2, ACS Appl. Mater. Interfaces, 2017, 9(4), 3817–3823 CrossRef CAS PubMed.
- S. Li and J. Jiang, Adsorption behavior analyses of several small gas molecules onto Rh-doped single-walled carbon nanotubes, Appl. Phys. A: Mater. Sci. Process., 2017, 123(10), 669 CrossRef.
- M. Giovanni, H. L. Poh, A. Ambrosi, G. Zhao, Z. Sofer, F. Šaněk, B. Khezri, R. D. Webster and M. Pumera, Noble metal (Pd, Ru, Rh, Pt, Au, Ag) doped graphene hybrids for electrocatalysis, Nanoscale, 2012, 4(16), 5002–5008 RSC.
- Y. Fan, J. Zhang, Y. Qiu, Z. Jia, Y. Zhang and G. Hu, A DFT study of transition metal (Fe, Co, Ni, Cu, Ag, Au, Rh, Pd, Pt and Ir)-embedded monolayer MoS2 for gas adsorption, Comput. Mater. Sci., 2017, 138, 255–266 CrossRef CAS.
- B. Delley, From molecules to solids with the DMol3 approach, J. Chem. Phys., 2000, 113(18), 7756–7764 CrossRef CAS.
-
H. Cui, X. Zhang, D. Chen, J. Tang. Adsorption mechanism of SF6 decomposed species on pyridine-like PtN3 embedded CNT: a DFT study. Applied Surface Science, 2018, 447.
- A. Tkatchenko, R. Di Stasio Jr, M. Head-Gordon and M. Scheffler, Dispersion-corrected Møller–Plesset second-order, J. Chem. Phys., 2009, 131(9), 171 CrossRef PubMed.
- B. Delley, Hardness conserving semilocal, Phys. Rev. B: Condens. Matter Mater. Phys., 2002, 66(15), 155125 CrossRef.
- D. Chen, X. Zhang, J. Tang, H. Cui and Y. Li, Noble metal (Pt or Au)-doped monolayer MoS2 as a promising adsorbent and gas-sensing material to SO2, SOF2 and SO2F2: a DFT study, Appl. Phys. A: Mater. Sci. Process., 2018, 124(2), 194 CrossRef.
- H. Cui, X. Zhang, J. Zhang and J. Tang, Adsorption behaviour of SF6 decomposed species onto Pd4-decorated single-walled CNT: a DFT study, Mol. Phys., 2018,(53), 1–7 Search PubMed.
- W. Ju, T. Li, X. Su, H. Li, X. Li and D. Ma, Au cluster adsorption on perfect and defective MoS2 monolayers: structural and electronic properties, Phys. Chem. Chem. Phys., 2017, 19, 20735–20748 RSC.
- S. G. Lee, J. Il Choi, W. Koh and S. S. Jang, Adsorption of β-D-glucose and cellobiose on kaolinite surfaces: density functional theory (DFT) approach, Appl. Clay Sci., 2013, 71, 73–81 CrossRef CAS.
- P. Wu, N. Yin, P. Li, W. Cheng and M. Huang, The adsorption and diffusion behavior of noble metal adatoms (Pd, Pt, Cu, Ag and Au) on a MoS2 monolayer: a first-principles study, Phys. Chem. Chem. Phys., 2017, 19(31), 20713–20722 RSC.
- Y. Ma, Y. Dai, M. Guo, C. Niu, J. Lu and B. Huang, Electronic and magnetic properties of perfect, vacancy-doped, and nonmetal adsorbed MoSe2, MoTe2 and WS2 monolayers, Phys. Chem. Chem. Phys., 2011, 13(34), 15546 RSC.
- Y. Li, X. Zhang, D. Chen, S. Xiao and J. Tang, Adsorption behavior of COF2 and CF4 gas on the MoS2 monolayer doped with Ni: a first-principles study, Appl. Surf. Sci., 2018, 443 Search PubMed.
- X. Zhang, H. Cui, D. Chen, X. Dong and J. Tang, Electronic structure and H2S adsorption property of Pt3 cluster decorated (8, 0) SWCNT, Appl. Surf. Sci., 2018, 428 Search PubMed.
- M. D. Ganji, N. Sharifi, M. Ardjmand and G. A. Morteza, Pt-decorated graphene as superior media for H2S adsorption: a first-principles study, Appl. Surf. Sci., 2012, 261(3), 697–704 CrossRef CAS.
- P. Pyykkö and M. Atsumi, Molecular single-bond covalent radii for elements 1-118, Chemistry, 2009, 15(1), 186–197 CrossRef PubMed.
- A. J. Yang, D. W. Wang, H. W. Xiao, J. F. Chu, P. L. Lv, Y. Liu and Z. R. Ming, Phosphorene: A Promising Candidate for Highly Sensitive and Selective SF6 Decomposition Gas Sensors, IEEE Electron Device Lett., 2017, 38(7), 963–966 CAS.
- S. W. Han, G. B. Cha, Y. Park and S. C. Hong, Hydrogen physisorption based on the dissociative hydrogen chemisorption at the sulphur vacancy of MoS2 surface, Sci. Rep., 2017, 7(1), 7152–7158 CrossRef PubMed.
- W. Wei, Y. Dai and B. Huang, In-plane interfacing effects of two-dimensional transition-metal dichalcogenide heterostructures, Phys. Chem. Chem. Phys., 2016, 18(23), 15632–15638 RSC.
- H. Cui, Q. Li, G. Qiu and J. Wang, Carbon-chain inserting effect on electronic behavior of single-walled carbon nanotubes: a density functional theory study, MRS Commun., 2018, 8(1), 189–193 CrossRef CAS.
- F. Schedin, A. K. Geim, S. V. Morozov, E. W. Hill, P. Blake, M. I. Katsnelson and K. S. Novoselov, Detection of individual gas molecules adsorbed on graphene, Nat. Mater., 2007, 6(9), 652–655 CrossRef CAS PubMed.
- Y. H. Zhang, Y. B. Chen, K. G. Zhou, C. H. Liu, J. Zeng, H. L. Zhang and Y. Peng, Improving gas sensing properties of graphene by introducing dopants and defects: a first-principles study, Nanotechnology, 2009, 20(18), 185504 CrossRef PubMed.
- S. Peng, K. Cho, P. Qi and H. Dai,
Ab initio study of CNT NO2 gas sensor, Chem. Phys. Lett., 2004, 387(4), 271–276 CrossRef CAS.
- H. Cui, K. Zheng, Y. Zhang, H. Ye and X. Chen, Superior Selectivity and Sensitivity of C3N Sensor in Probing Toxic Gases NO2 and SO2, IEEE Electron Device Lett., 2018, 39(2), 284–287 CAS.
- M. Sharma, P. Jamdagni, A. Kumar and P. K. Ahluwalia, Interactions of gas molecules with monolayer MoSe2: a first principle study, AIP Conf. Proc., 2016, 52903 Search PubMed.
- H. Qin, F. Chuang, X. Luan and D. Yang, First-principles investigation of adsorption behaviors of small molecules on penta-graphene, Nanoscale Res. Lett., 2018, 13(1), 264 CrossRef PubMed.
- H. Wei, Y. Gui, J. Kang, W. Wang and C. Tang, A DFT Study on the Adsorption of H2S and SO2 on Ni Doped MoS2 Monolayer, Nanomaterials, 2018, 646–657 CrossRef PubMed.
- B. Zhao, C. Y. Li, L. L. Liu, B. Zhou, Q. K. Zhang, Z. Q. Chen and Z. Tang, Adsorption of gas molecules on Cu impurities embedded monolayer MoS2: a first-principles study, Appl. Surf. Sci., 2016, 382, 280–287 CrossRef CAS.
- H. Cui, X. Zhang and D. Chen, Borophene: a promising adsorbent material with strong ability and capacity for SO2 adsorption, Appl. Phys. A: Mater. Sci. Process., 2018, 124(9), 636 CrossRef.
|
This journal is © The Royal Society of Chemistry 2019 |