DOI:
10.1039/C8NA00034D
(Paper)
Nanoscale Adv., 2019,
1, 696-705
Development of an efficient phenolic sensor based on facile Ag2O/Sb2O3 nanoparticles for environmental safety†
Received
12th June 2018
, Accepted 24th October 2018
First published on 9th November 2018
Abstract
The facile hydrothermal method was used to prepare low-dimensional doped Ag2O/Sb2O3 nanoparticles (NPs) at low temperature in alkaline medium. The calcined NPs were characterized in detail by FTIR, UV/vis, FESEM, XPS, EDS, and XRD. A thin layer of Ag2O/Sb2O3 NPs was deposited onto a glassy carbon electrode (GCE) using Nafion (5% Nafion suspension in ethanol) conducting binder, which formed the working electrode of the selective 3-methoxyphenol electrochemical sensor probe. The proposed chemical sensor exhibits high sensitivity, long-term stability, and enhanced electrochemical responses towards 3-methoxyphenol. Response to 3-methoxyphenol is linear over the concentration range (LDR) of 0.09 nM to 0.09 mM. The analytical parameters of the sensor such as sensitivity, stability, response time, linearity, LDR, robustness, selectivity etc. were evaluated by an electrochemical approach. The sensor probe fabricated with Ag2O/Sb2O3 NPs seems to be a promising candidate for effective and reliable electrochemical detection of hazardous and carcinogenic chemicals in the environment and health care fields in large scales.
Introduction
Phenol and its derivatives are responsible for the toxic contamination of water (ground and underground).1 Due to the high environmental toxicity of phenol including humans, animals, plants, aquatic life and other living organisms,2–4 phenolic compounds are considered as priority environmental pollutants by the United States Environmental Protection Agency (USEPA) and the European Commission. These pollutants generally come into the environment as waste effluent from various industries such as paper, dyes, detergent, resins, herbicides, pharmaceuticals, pesticides, explosives, plastics, coal mining and crude oil refining.5,6 There are some traditional methods to detect phenolic compounds such as capillary electrophoresis, decent gas or liquid chromatography, spectrophotometry, fluorimetry, and mass spectrometry. However, these processes have the limitations of high cost, time consumption and complicated detection procedure.7–10 In contrast, the electrochemical method (I–V) has great advantages such as low cost, simplicity of procedure, rapid and short response time, selectivity and good sensitivity.11 Semi-conductive metal oxides with a wide band gap energy (eV) such as TiO2, ZnO, SnO2, Fe2O3, Ag2O, and Sb2O3 have been exclusively investigated as sensing elements to assemble electrochemical sensors.12–14 According to Rahman et al., the 4-methoxyphenol sensor based on NiS2/CNT nanocomposites exhibited good sensitivity (0.632 μA μM−1 cm−2) with a low detection limit (30.0 pM).15 Another report claimed that the phenolic sensor using 3D graphene micro-pillar showed a sensitivity of 3.9 nA mM−1 cm−2 and a detection limit of 50.0 nM.16 In the last decade, conducting polymer–carbon nanotubes (CNT) composited with various transition metal oxides achieved great attention as sensing material to fabricate chemical sensors. A 3-methoxyphenol chemical sensor based on poly (o-anisidine)/silverized multiwall carbon nanotube (POAS/Ag/MWCNT) nanocomposite exhibited higher sensitivity with a low detection limit.17
Since, 3-methoxyphenol is highly toxic and has serious risk of illness, an urgent initiative should be taken for its significant detection using a consistent and reliable technique. Herein, we report a 3-methoxyphenol chemical sensor based on Ag2O/Sb2O3 nanoparticles by electrochemical method. A thin layer of synthesized NPs was deposited onto a GCE electrode with conducting binder to fabricate the working electrode of the 3-methoxyphenol chemical sensor. This working electrode detected the 3-methoxyphenol by a reliable electrochemical technique. It should be noted that the proposed chemical sensor is exceptional and newly developed based on Ag2O/Sb2O3 NPs/binder/GCE. It is noted that the proposed chemical sensor is exceptional and newly developed sensor totally based on Ag2O/Sb2O3 NPs/binder/GCE by electrochemical method.
Experimental sections
Materials and methods
Analytical grade chemicals such as ammonium hydroxide, Nafion (5% Nafion suspension in ethanol), acetone, 2-nitrophenol, 3-methoxyphenol, 4-aminophenol, 4-methoxyphenol, bisphenol A, ethanol, hydrazine, methanol, p-nitrophenol, monosodium phosphate and disodium phosphate were purchased from Sigma-Aldrich (USA). These were used as received. The maximum visible light absorption (λmax) and the corresponding band gap energy (Ebg) of the synthesized Ag2O/Sb2O3 NPs were investigated by UV/vis spectrophotometer and Thermo Scientific NICOLET iS50 FTIR spectrometer (Madison, WI, USA) was used to record FTIR spectrum. To quantify the binding energies (eV) of O, Ag and Sb, XPS was performed on the prepared Ag2O/Sb2O3 NPs using K-α spectrometer (K-α1 1066, Germany). Elemental and morphological analyses of the Ag2O/Sb2O3 NPs were examined by of FESEM (JEOL, JSM-7600F, Japan) analysis equipped with EDS. The phase crystallinity of the nanoparticles was explored by XRD patterns obtained at atmospheric condition. The current vs. potential technique was employed to detect 3-methoxyphenol at the required (0 to +1.5 V) range of applied electrical potential using a Keithley electrometer (6517A, USA). Ag2O/Sb2O3 NPs were deposited on a GCE with the help of Nafion, a conducting binder, to construct the desired working electrode of the 3-methoxyphenol electrochemical sensor. The assembled chemical sensor was applied for successive detection of 3-methoxyphenol in phosphate buffer medium.
Preparation of Ag2O/Sb2O3 NPs by hydrothermal process
Silver nitrate (AgNO3), antimony tetrachloride (SbCl3) and ammonium hydroxide (NH4OH) were used as the reacting precursors to prepare Ag2O/Sb2O3 NPs by hydrothermal method. The hydrothermal technique is a common system for the synthesis of doped nanomaterials with smaller grain size and phase formation. Following this technique, a conical flask (250.0 mL) was filled with 100 mL distilled water and measured amounts of AgNO3 and SbCl3 were dissolved in this conical flask with continuous magnetic stirring. To adjust the pH of the solution at 10.5, NH4OH was added to the resultant solution and was kept inside a hydrothermal system at 150 °C for several hours. As stated in reactions (i) to (iv), AgNO3 and SbCl3 were hydrolyzed to form Ag+ and Sb+3 and subsequently, the metal ions were co-precipitated in the form of metal hydroxides due to increase in pH. At pH 10.5, all the metal ions had precipitated quantitatively as Ag(OH)·Sb(OH)3·nH2O. The metal hydroxide formation is completely analogous with the reported articles.18,19 | NH4OH(s) → NH4(aq)+ + OH(aq)− | (i) |
| AgNO3(s) → Ag(aq)+ + NO(aq)3− | (ii) |
| SbCl3(s) → Sb(aq)3+ + 3Cl(aq)− | (iii) |
| Sb(aq)3+ + Ag(aq)+ + 4OH(aq)− + nH2O ⇆ Sb(OH)3·Ag(OH)·nH2O(aq) | (iv) |
Finally, the resultant precipitate was separated from the reaction medium, washed with de-ionized water and acetone. After that, it was placed inside an oven at 110 °C overnight.
The produced metal hydroxides were calcined in a furnace (Barnstead Thermolyne, 6000 Furnace, USA) at 500 °C for around 6 hours. In the muffle furnace, the metal hydroxides were oxidized in the presence of atmospheric oxygen. According to the Ostwald-ripening method of nanoparticles growth mechanism, Ag2O/Sb2O3 NPs nuclei are initiated by self- and mutual-aggregation and the nanocrystals are re-aggregated again at higher temperatures to form the Ag2O/Sb2O3 nanocrystals. The molecular arrangement inside of the nanocrystals is based on the van der-Waals forces between each other's counterparts. The reaction in the muffle furnace is supposed to occur as below (v).
| 2Ag(OH)·Sb(OH)3 + O2 → Ag2O–Sb2O3(s)↓ + 4H2O(aq) | (v) |
The growth mechanism of the doped nanoparticles of Ag2O/Sb2O3 is similar to the growth patterns reported previously.20,21 The formation mechanism of Ag2O/Sb2O3 NPs is presented in Scheme 1. This is the first time calcined Ag2O/Sb2O3 NPs were applied to detect 3-methoxyphenol, and there is no report available regarding this.
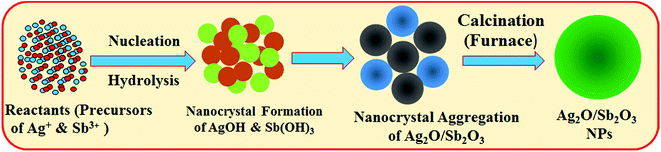 |
| Scheme 1 Growth mechanism of Ag2O/Sb2O3 NPs produced by the low temperature hydrothermal method. | |
Fabrication of GCE with Ag2O/Sb2O3 NPs
A round rod-shaped glassy carbon nanotube (GCE) was used to fabricate the working electrode by coating Ag2O/Sb2O3 NPs using the conducting binder 5% Nafion. 0.1 M phosphate buffer (PBS-solution) at pH 7.0 was prepared by mixing equimolar concentrations of 0.2 M Na2HPO4 and 0.2 M NaH2PO4 solutions in 100.0 mL de-ionized water at ambient conditions. The diameter of the tip of the working electrode is 1.0 mm. To fabricate the working electrode from GCE, a slurry of Ag2O/Sb2O3 NPs was prepared in ethanol and used to coat on GCE at ambient conditions. For the improvement of the binding strength between the glassy carbon electrode and the Ag2O/Sb2O3 NPs, a drop of Nafion (5% Nafion suspension in ethanol) was added on to the modified GCE. The slurry of NPs was prepared in ethanol and coated as thin uniform layer onto GCE. After drying, a drop of Nafion was added as chemical-glue to bind the material and improve the binding strength as well as electron transfer rate. Then, the electrode was placed inside an oven at 35.0 °C temperature until the conducting film was dried completely. An electrochemical cell was assembled with Ag2O/Sb2O3 NPs/binder/GCE and Pt-wire (dia., 1.5 mm), where Ag2O/Sb2O3 NPs/binders/GCE was acting as the working electrode and Pt-wire was the counter electrode. A range of 3-methoxyphenol solutions based on concentration (full concentration range: 0.9 mM to 0.09 nM) was prepared. The sensitivity of the modified working electrode based on Ag2O/Sb2O3 NPs was measured from the slope of the calibration curve (the ratio of current versus concentration). In the same way, the detection limit (DL) and the linear dynamic range (LDR) were calculated from the ratio of 3N/S (ratio of noise × 3 vs. sensitivity). The utilized electrometer is a simple two-electrode system and was supplied with a constant voltage for I–V measurement. The amount of 0.1 M PBS-solution was kept constant in the beaker as 10.0 mL throughout the chemical investigations.
Results and discussions
Choice of materials
Facile hydrothermally prepared Ag2O/Sb2O3 NPs have employed a great deal of consideration due to their chemical, structural, physical, and optical properties in terms of large-active surface area, high-stability, high porosity, and permeability, which directly depend on their structural morphology. Ag2O/Sb2O3 NPs were prepared by using the reactant precursors (AgNO3 & SbCl3) in a basic medium at low-temperature. They were synthesized by hydrothermal method using NH4OH reducing agents at ambient conditions. This technique has several advantages including facile preparation, accurate control of reactant temperature, ease of handling, one-step reaction, and high-porosity. Optical, morphological, electrical, and chemical properties of the Ag2O/Sb2O3 NPs are of huge significance from the scientific aspect compared to other undoped materials. Non-stoichiometry, mostly oxygen vacancies, makes it conducting in the nanomaterials. The formation energy of oxygen vacancies and metal interstitials in semiconductors is very low, and thus, these defects are formed easily resulting in experimentally elevated conductivity of the Ag2O/Sb2O3 NPs compared to other undoped materials such as Ag2O, Sb2O3, CuO, NiO, etc. Ag2O/Sb2O3 NP materials have also attracted considerable interest owing to their potential applications in opto-electronics, electro-analytics, selective detection of bioassays, biological devices, hybrid-composites, electron-field emission sources for emission exhibits, biochemical detection, and surface-enhanced Raman properties. Doped Ag2O/Sb2O3 NPs display improved performance due to the large-active surface area, which increases the conductivity and current responses of the Ag2O/Sb2O3 NPs/Nafion/GCE assembly during electrochemical investigations.
Structural analyses
To assess the photosensitivity of the prepared Ag2O/Sb2O3 NPs, UV/vis spectroscopy analysis was carried out. Absorption spectrum of the Ag2O/Sb2O3 NPs was measured as a function of wavelength as presented in Fig. 1(a). The observed spectrum shows an intense and wide absorption band at 307 nm identical to the transition of valence band electron of Ag2O/Sb2O3 NPs from lower to higher energy level.14,22 According to eqn (vi), the calculated band gap energy is 4.04 eV.23
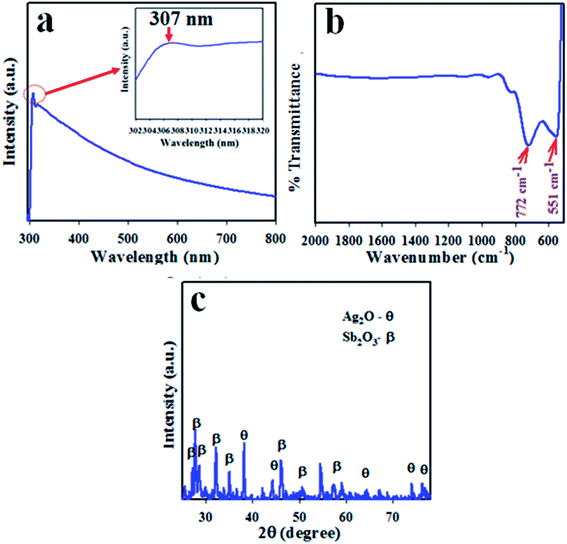 |
| Fig. 1 Optical and morphological evaluation by (a) UV spectrum, (b) FTIR and (c) XRD pattern of the Ag2O/Sb2O3 nanoparticles. | |
The synthesized Ag2O/Sb2O3 NPs were subjected to FTIR investigation to determine the functional properties of the atom or molecule from the corresponding atomic and molecular vibrations. FTIR was performed in the range of 450–4000 cm−1 as illustrated in Fig. 1(b). From the observed FTIR spectrum, the peaks were obtained at 551 and 772 cm−1. The existence of major peaks at 551 and 772 cm−1 resulted from the stretching vibration of metallic (Ag–O & Sb–O) oxygen bonds respectively.22,24 To investigate the structural properties and phase (crystallinity) of the synthesized Ag2O/Sb2O3 NPs, XRD was implemented. The resultant XRD pattern illustrated in Fig. 1(c) conveys that the synthesized Ag2O/Sb2O3 NPs consist of distinct phases for Ag2O and Sb2O3. According to the XRD diffraction pattern, the reflected peaks of Ag2O denoted as θ are (111), (200), (220), (222) and (311), which are analogous with previous reports on bulk Ag2O25–27 and JCPDS card no. 4-0783. In addition, the several peaks of Sb2O3 indicated as β represent the phases (012), (110), (210), (410), (503), and (602) and matched well with those reported in the literature.28,29 The crystal size of the nanomaterial can be measured from its XRD diffraction pattern by implementation of the Scherrer equation, which is presented in eqn (vii).
| D = 0.9λ/β cos θ | (vii) |
Here,
λ is the wavelength (X-ray radiation = 1.5418 Å) and
β is the full width at half maximum, corresponding to highest intense peak, and
θ is the diffraction angle.
30 The average calculated cross sectional diameter of Ag
2O/Sb
2O
3 NPs is 21.38 nm.
Morphological and elemental analyses
FESEM imaging was performed to study the morphology of the synthesized Ag2O doped Sb2O3 NPs and the resultant representative low and high magnification images of FESEM are presented in Fig. 2(a and b). The average diameter of the doped Ag2O/Sb2O3 NPs is calculated as 35.3 nm, in the range of 30.0 to 40.0 nm. From FESEM investigation, it is confirmed that the produced nano-doped materials have the shape of nanoparticles.31–33 The FESEM investigation has similarities with the argument of EDS analysis illustrated in Fig. 2(c and d) and the structural composition of prepared nanoparticles are authenticated from the EDS investigation. Therefore, the EDS analysis reveals that silver (Ag), oxygen (O), and antimony (Sb) exist in the NPs in the composition O (20.04 wt%), Sb (77.34 wt%), and Ag (2.62 wt%). No other supplementary peaks were detected and the confirmation about the existence of O, Sn and Cd in the prepared nanomaterials is accredited by EDS analysis.14,34
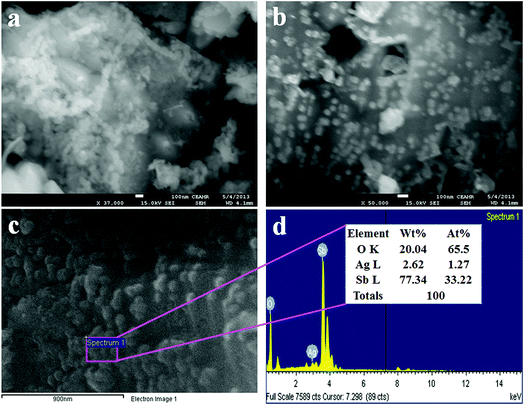 |
| Fig. 2 (a and b) FESEM images from low to high magnification and (c and d) elemental analysis of the Ag2O/Sb2O3 NPs. | |
Binding energy analysis
The quantitative spectroscopic technique of XPS was applied to investigate the nature of species existing in the prepared Ag2O/Sb2O3 NPs. Generally, the atomic composition with corresponding chemical formula and the oxidation state of the species present in the nanomaterial can be scrutinized efficiently using this technique.35 The XPS spectra of the Ag2O/Sb2O3 NPs is shown in Fig. 3. Two main identical peaks of Ag3d orbit of the synthesized Ag2O/Sb2O3 NPs can be observed, as illustrated in Fig. 3(a). Apparently, these two peaks are equal and indicate equal oxidation states of Ag. The observed binding energies of Ag3d5/2 and Ag3d3/2 are 368 and 373 eV, respectively, which are the characteristic values of the oxidation of Ag+ in Ag2O.36,37 The O1s peak of the Ag2O/Sb2O3 NPs could be clearly recognized and is centered at 533 eV as represented in Fig. 3(b). It can be ascribed to the Ag–O bond of Ag2O.38 The XPS spectrum of Sb3d is shown in Fig. 3(c) and two characteristic peaks of Sb3d5/2–O1s and Sb3d3/2 are identified centered at 533 eV and 540 eV, respectively. The peaks significantly confirmed the attribution of Sb3+ state.39,40
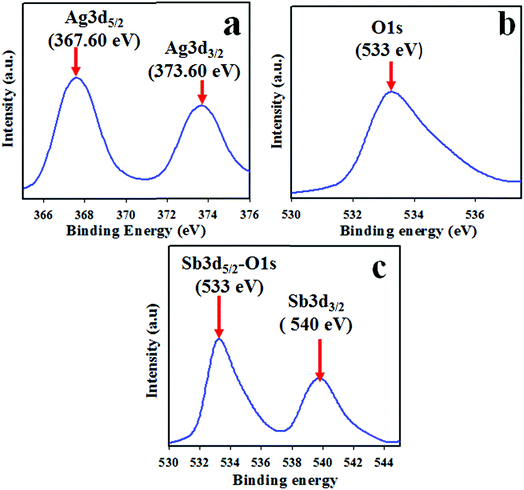 |
| Fig. 3 Binding energy analysis of Ag2O/Sb2O3 NPs by XPS. (a) Spin orbit Ag3d level, (b) O1s, and (c) spin orbit Sb3d level. | |
Applications: detection of 3-methoxyphenol by Ag2O/Sb2O3 NPs
The electrochemical sensor based on Ag2O/Sb2O3 NPs/Nafion/GCE was constructed with the aim to detect the selective toxin (3-methoxyphenol) in phosphate buffer system. The projected chemical sensor has several benefits including high stability in air, inert nature in chemical environment, enhanced electrochemical activity during detection, easy in terms of handling, assembly and fabrication, and above all, safe chemo-characteristics. To prepare the chemical sensor selective to 3-methoxyphenol, a slurry of Ag2O/Sb2O3 NPs was prepared in ethanol, deposited as a uniform thin layer onto the GCE (commercial glassy carbon electrode) and the modified GCE was dried at ambient conditions. To improve the binding strength between the uniform layer of NPs and the GCE, a drop of Nafion (5% Nafion suspension in ethanol) was added and the electrode was placed inside an oven at 35.0 °C temperature until the conducting film was dried completely. Nafion improves the binding strength as well as the electron transfer rate of the modified GCE.41,42 During the sensing performance of 3-methoxyphenol electrochemical sensor in the desired phosphate buffer system, the current vs. potential of thin-film Ag2O/Sb2O3 NPs/binder/GCE electrochemical sensor was assessed. The holding period of the electrometer was set at 1.0 s. The proposed detection (oxidation) mechanism of 3-methoxyphenol is illustrated in Scheme 2. As stated in the proposed oxidation mechanism of 3-methoxyphenol, an enrichment of electron in the buffer medium is observed, which causes the amendment of I–V response. At the beginning of 3-methoxyphenol oxidation, dissolved oxygen is absorbed on the surface of the working electrode and ionized into O− ion, which reacts with 3-methoxyphenol to form p-benzoquinone, methanol and electron as presented in reactions (viii) to (xi). Oxidation of 3-methoxyphenol is similar to other reported articles.15,43 A pictorial representation of the application of the Ag2O/Sb2O3 NPs modified electrode is demonstrated in Scheme 2.
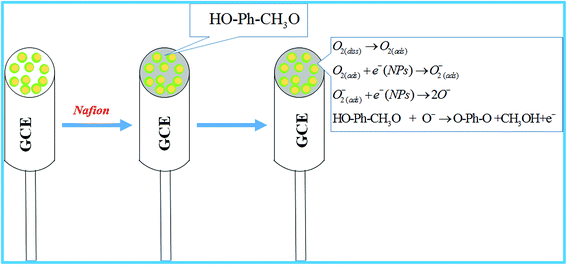 |
| Scheme 2 The expected mechanism for determination of 3-methoxyphenol by Ag2O/Sb2O3 nanoparticles using electrochemical approach. | |
According to the electrochemical oxidation process of 3-methoxyphenol, an electron is released from the conduction band and is responsible for the amendment of I–V response of the Ag2O/Sb2O3 NPs/binder/GCE electrochemical sensor.
| O2(ads) + e−(NPs) → O2(ads)− | (ix) |
| O2(ads)− + e−(NPs) → 2O− | (x) |
| HO–Ph–CH3O + O– → O–Ph–O + CH3OH + e− | (xi) |
The assembled Ag2O/Sb2O3 NPs/binder/GCE electrode is not equally responsive in all buffer media. Therefore, the fabricated electrode was investigated in various phosphate buffer systems (pH of 5.7, 6.5, 7.0, 7.5 and 8.0) to obtain the highest I–V response. As observed from Fig. 4(a), the projected electrochemical sensor is found to give a maximum response in pH 6.5 at an applied potential of 0 to +1.5 V.
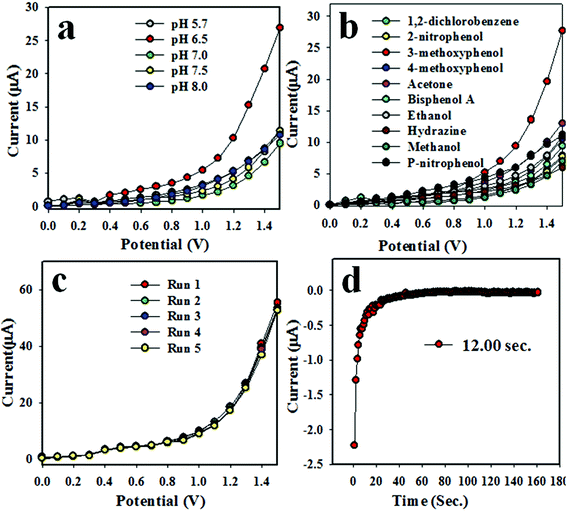 |
| Fig. 4 Optimization of the 3-methoxyphenol sensor with Ag2O/Sb2O3 NPs. (a) pH optimization, (b) selectivity, (c) repeatability, and (d) response time. | |
To investigate the selectivity of the assembled chemical sensor, a number of toxins such as acetone, 2-nitrophenol, 3-methoxyphenol, 4-aminophenol, 4-methoxyphenol, bisphenol A, methanol, ammonium hydroxide, and p-nitrophenol were examined at micro-level concentrations and an applied potential of 0 to +1.5 V in phosphate buffer medium of pH 6.5; the resultant I–V responses are illustrated in Fig. 4(b). As perceived from Fig. 4(b), 3-methoxyphenol exhibited the highest current response among all toxins. As reproducibility is a very important factor in the analytical performance of an electrochemical sensor, the 3-methoxyphenol sensor was assessed at a 0.9 μM concentration of 3-methoxyphenol and an applied potential 0 to +1.5 V in pH 6.5 phosphate buffer medium as demonstrated in Fig. 4(c). The five runs were practically indistinguishable and provides information about the reliability of detection of toxin in real-time in the field. To verify the accuracy of reproducibility in performance, the relative standard deviation (RSD) of current data at applied potential +1.2 V was measured and it was found to be 1.33%. Thus, it can be predicted that the proposed electrochemical sensor based on Ag2O/Sb2O3 NPs/binder/GCE is proficient enough to detect 3-methoxyphenol in real environmental samples. The response time is yet another analytical performance parameter to measure the efficiency of the electrochemical sensor. This test was performed using 0.9 μM concentration of 3-methoxyphenol solution. The excellent result for the response time of 12.0 second is obtained as illustrated in Fig. 4(d). This result might be satisfactory.
The intensity of I–V response of 3-methoxyphenol chemical sensor varies with the concentration of 3-methoxyphenol. Therefore, the I–V responses of Ag2O/Sb2O3 NPs/binder/GCE sensor were measured at various concentrations of 3-methoxyphenol ranging from 0.9 mM to 0.09 nM as shown in Fig. 5(a). This was experimented at an applied potential of 0 to +1.5 V in phosphate buffer medium of pH 6.5. As demonstrated in Fig. 5(a), I–V responses are distinguishable from lower to higher concentrations. Therefore, it can be concluded that I–V responses are amplified with increasing concentration of the electrolyte (3-methoxyphenol). To scale up the analytical limit of the proposed 3-methoxyphenol electrochemical sensor, a linear calibration curve was plotted at an applied potential of +1.2 V from the lower-higher concentration of 3-methoxyphenol solution as shown in Fig. 5(b). The regression coefficient (r2: 0.99), sensitivity (11.67 μA μM−1 cm−2), linear dynamic ranges (LDR: 0.09 nM to 0.09 mM) and the detection limit (LD: 0.08 ± 0.004 nM) were calculated from the resultant calibration curve at signal to noise ratio of 3. The estimated analytical performances including sensitivity, linear dynamic range (LDR) and detection limit (DL) are appreciable.
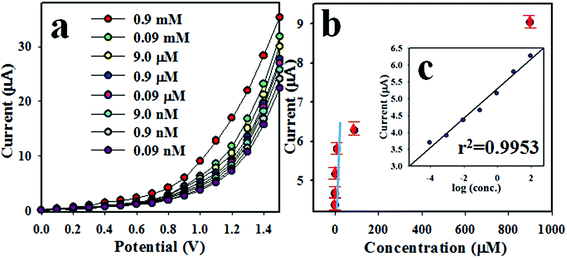 |
| Fig. 5 (a) Concentration variation of 3-methoxyphenol sensor based on Ag2O/Sb2O3 NPs/GCE by I–V method and (b) calibration curve (inset: log[3-methoxyphenol. conc.] vs. current). | |
As seen from Fig. 5(a), I–V responses varied with the corresponding concentration of 3-methoxyphenol. A similar tendency for current vs. potential has been reported by previous authors.15,44,45 At the beginning of 3-methoxyphenol detection performance, the targeted 3-methoxyphenol molecules occupy a small fraction of surface on Ag2O/Sb2O3 NPs/binder/GCE film and the corresponding oxidation reaction of the 3-methoxyphenol starts progressively. With increasing analyte (3-methoxyphenol) concentration in the buffer medium, the reaction on the working electrode surface is increased and a larger surface coverage corresponding to the 3-methoxyphenol molecules is observed. On additional enrichment of the analyte (3-methoxyphenol) concentration, a highly moderate current versus potential response is experimented and the surface coverage by the corresponding analyte on the surface of Ag2O/Sb2O3 NPs/GCE working electrode is maximum. On further enrichment of analyte concentration, the surface coverage by 3-methoxyphenol molecules touches its saturation. As we observed, the proposed 3-methoxyphenol sensor is shows a very short response time of around 12 s, which is required for the steady state saturation. Therefore, it can be summarized that the proposed 3-methoxyphenol chemical sensor based on Ag2O/Sb2O3 NPs/GCE assembly can be engaged for successful detection of the targeted toxin (3-methoxyphenol). As perceived from Fig. 5(b), the current data are constantly distributed along the linear plot in the concentration range of 0.09 nM–0.09 mM, which is characterized as the LDR and this exploration is considered as the reliability of the method. Since, the 3-methoxyphenol chemical sensor exhibited a moderately high sensitivity of 11.67 μA μM−1 cm−2, it can be considered to have super adsorption capacity, active catalytic decomposition ability, and excellent biocompatibility.15,17,45 In terms of selectivity and detection limit, the proposed 3-methoxyphenol chemical sensor based on Ag2O/Sb2O3 NPs has showed a reasonably good performance compared to other electrochemical sensors tested earlier as illustrated in Table 1. To measure the electrochemical activities of individual (Ag2O NPs and Sb2O3 NPS) and combinational (Ag2O/Sb2O3 NPs) metal oxides, a number of experiments were conducted at an applied potential of 0 to +1.5 V and 0.9 μM concentration of 3-methoxyphenol in phosphate buffer medium of pH 6.5 as demonstrated in Fig. S1 in the ESI.† It is observed that the combinational effect of Ag2O/Sb2O3 NPs showed a supreme I–V response. Therefore, it can be mentioned that the fabricated 3-methoxyphenol chemical sensor is simple and efficient in detecting 3-methoxyphenol by the current versus potential method in an electro-chemical approach. The sensing performance of the earlier testified metal oxides based phenolic sensors are associated and summarized in Table 1.15–17,46
Table 1 Performance of 3-methoxyphenol and other phenolic sensors with various nanomaterials or nanocomposites by electrochemical approachesa
Modified electrode |
DL |
LDR |
Sensitivity |
Ref. |
DL (detection limit), LDR (linear dynamic range).
|
NiS2-CNT NCs/GCE |
30 pM |
0.01 nM–10.0 mM |
0.63 μA μM−1 cm−2 |
15
|
3D graphene micropiller/GCE |
50 nM |
50.0 nM–2.0 μM |
3.9 × 10−3 μA μM−1 cm−2 |
16
|
Ag-MWCNT/GCE |
0.36 nM |
0.4 nM–40.0 mM |
3.829 × 10−3 μA μM−1 cm−2 |
17
|
MWCNT/GCE |
90 nM |
0.2–1.6 μM |
7.0 μA μM−1 cm−2 |
44
|
NPG thin-film/GCE |
0.5 μM |
0.5–200 μM |
0.15 μA μM−1 cm−2 |
45
|
Ag2O/Sb2O3 NPs/GCE |
0.08 nM |
0.09 nM–0.09 mM |
11.67 μA μM−1 cm−2 |
This study |
The analysis of real samples
To validate the probe, the 3-methoxyphenol sensor based on Ag2O/Sb2O3 NPs coated onto GCE with conducting binder was used to detect 3-methoxyphenol in various real and extracted environmental samples. We measured 3-methoxyphenol in industrial effluents, PC baby bottle, PC water bottle, and PVC food packaging bag. The results showed that 3-methoxyphenol detection is possible from the environmental samples, which are represented in Table 2.
Table 2 Measured concentration of 3-methoxyphenol analytes in real samplea
Real sample |
Observed current (μA) |
Average (μA) |
Calculated conc. (μM) |
% RSD |
R1 |
R2 |
R3 |
R4 |
R (reading), RSD (relative standard deviation).
|
Industrial effluents |
1.56 |
1.67 |
1.69 |
1.77 |
1.6725 |
0.160853 |
5.18 |
PC baby bottle |
0.509 |
0.512 |
0.457 |
0.468 |
0.4865 |
0.046789 |
5.78 |
Drinking-water-battle |
0.460 |
0.475 |
0.478 |
0.476 |
0.4723 |
0.045423 |
1.75 |
PVC food packaging |
0.860 |
0.879 |
0.853 |
0.733 |
0.8313 |
0.079950 |
7.99 |
Conclusions
In summary, doped Ag2O/Sb2O3 nanoparticles were prepared by a facile hydrothermal process and characterized by SEM, EDS, FTIR, XPS and XRD. A thin layer of Ag2O/Sb2O3 NPs was applied onto a GCE using a conducting binder to prepare sensor probe for 3-methoxyphenol detection. The proposed 3-methoxyphenol chemical sensor has been shown to have excellent performance based on the electrochemical parameters such as detection limit, linear dynamic range, sensitivity, repeatability, robustness, stability, and response time. The calibration plot is linear over a large concentration range. The calculated selectivity and detection limit is 11.67 μA μM−1 cm−2 and 0.08 pM, respectively, at signal to noise ratio of 3. Therefore, this sensor can be used for the detection of the toxic chemical existing in real environmental effluents to ensure ecological and environmental safety.
Conflicts of interest
There are no conflicts to declare.
Acknowledgements
Center of Excellence for Advanced Materials Research (CEAMR) at King Abdulaziz University, Jeddah, Saudi Arabia is highly acknowledged for providing their lab facilities for the fabrication, characterization, and application of the sensors.
References
-
I. G. Vincent, G. Angeletti and A. Bjorseth, Organic Micropollutants in the Aquatic Environment, Kluwer Academic Publishers, Dordrecht, 1991 Search PubMed.
- J. Li, D. Kuang, Y. Feng, F. Zhang, Z. Xu and M. Liu, A graphene oxide-based electrochemical sensor for sensitive determination of 4-nitrophenol, J. Hazard. Mater., 2012, 201, 250–259 CrossRef PubMed.
- P. Deng, Z. Xu, Y. Feng and J. Li, Electrocatalytic reduction and determination of p-nitrophenol on acetylene black paste electrode coated with salicylaldehyde modified chitosan, Sens. Actuators, B, 2012, 168, 381–389 CrossRef CAS.
- R. M. A. Tehrani, H. Ghadimi and S. A. Ghani, Electrochemical studies of two diphenols isomers at graphene nanosheet-poly (4-vinyl pyridine) composite modified electrode, Sens. Actuators, B, 2013, 177, 612–619 CrossRef CAS.
- F. Karim and A. N. M. Fakhruddin, Recent advances in the development of biosensor for phenol: a review, Sci. Biotechnol., 2012, 11, 261–274 CrossRef CAS.
- R. W. Takashima and K. Kaneto, Amperometric Phenol biosensor based pyrrole-copolymer film, Sens. Actuators, B, 2004, 102, 271–277 CrossRef.
- A. Arecchi, M. Scampicchio, S. Drusch and S. Mannino, Nanofibrous membrane based tyrosinase-biosensor for the detection of phenolic compounds, Anal. Chim. Acta, 2010, 659, 133–136 CrossRef CAS PubMed.
- C. D. Chriswell, R. C. Chang and J. S. Fritz, Chromatographic determination of phenols in water, Anal. Chem., 1975, 47, 1325–1329 CrossRef CAS PubMed.
- J. Poerschmann, Z. Zhang, F. D. Kopinke and T. Pawliszyn, Solid phase microextraction for determining the distribution of chemicals in aqueous matrices, Anal. Chem., 1997, 69, 597–600 CrossRef CAS.
- C. Nistor, J. Emnéus, L. Gorton and A. Ciucu, Improved stability and altered selectivity of tyrosinase based graphite electrodes for detection of phenolic compounds, Anal. Chim. Acta, 1999, 387, 309–326 CrossRef CAS.
- D. A. Oriero, I. O. Gyan, B. W. Bolshaw, I. F. Cheng and D. E. Aston, Electrospun biocatalytic hybrid silica–PVA-tyrosinase fiber mats for electrochemical detection of phenols, Microchem. J., 2015, 118, 166–175 CrossRef CAS.
- A. Umar, M. S. Akhtar, G. N. Dar and S. Baskoutas, Low-temperature synthesis of α-Fe2O3 hexagonal nanoparticles for environmental remediation and smart sensor applications, Talanta, 2013, 116, 1060–1066 CrossRef CAS PubMed.
- M. M. Rahman, S. B. Khan, H. M. Marwani and A. M. Asiri, ASnO2–Sb2O3 nanocomposite for selective adsorption of lead ions from water samples prior to their determination by ICP-OES, Microchim. Acta, 2015, 182, 579–588 CrossRef CAS.
- M. M. Rahman, S. B. Khan, A. Jamal, M. Faisal and A. M. Asiri, Fabrication of highly sensitive acetone sensor based on sonochemically prepared as-grown Ag2O nanostructures, Chem. Eng. J., 2012, 192, 122–128 CrossRef CAS.
- M. M. Rahman, J. Ahmed, A. M. Asiri, I. A. Siddiquey and M. A. Hasnat, Development of 4-methoxyphenol chemical sensor based on NiS2 -CNT nanocomposites, J. Taiwan Inst. Chem. Eng., 2016, 64, 157–165 CrossRef CAS.
- F. Liu, Y. Piao, J. S. Choi and T. S. Seo, Three-dimensional graphene micropillar based electrochemical sensor for phenol detection, Biosens. Bioelectron., 2013, 50, 387–392 CrossRef CAS PubMed.
- M. M. Rahman, A. Khan and A. M. Asiri, Chemical sensor development based on poly(oanisidine) silverized–MWCNT nanocomposites deposited on glassy carbon electrodes for environmental remediation, RSC Adv., 2015, 5, 71370–71378 RSC.
- M. M. Rahman, S. B. Khan, H. M. Marwani and A. M. Asiri, A SnO2–Sb2O3nanocomposite for selective adsorption of lead ions from water samples prior to their determination by ICP-OES, Microchim. Acta, 2015, 182, 579–588 CrossRef CAS.
- M. M. Rahman, S. B. Khan, A. Jamal, M. Faisal and A. M. Asiri, Fabrication of highly sensitive acetone sensor based on sonochemically prepared as-grown Ag2O nanostructures, Chem. Eng. J., 2012, 192, 122–128 CrossRef CAS.
- M. M. Rahman, S. B. Khan, A. M. Asiria and A. G. Al-Sehemi, Chemical sensor development based on polycrystalline gold electrode embedded low-dimensional Ag2O nanoparticles, Electrochim. Acta, 2013, 112, 422–430 CrossRef CAS.
- M. M. Rahman, S. B. Khan, H. M. Marwani and A. M. Asiri, Selective Divalent Cobalt Ions Detection Using Ag2O3–ZnO Nanocones by ICP-OES Method for Environmental Remediation, PLoS One, 2014, 9, 114084 CrossRef PubMed.
- K. R. Nemadea and S. A. Waghuley, LPG sensing performance of CuO–Ag2O bimetallicoxide nanoparticles, St. Petersburg Polytechnical University Journal: Physics and Mathematics, 2015, 1, 249–255 CrossRef.
- M. M. Rahmana, S. B. Khana, A. M. Asiri and A. G. Al-Sehemi, Chemical sensor development based on polycrystalline gold electrode embedded low-dimensional Ag2O nanoparticles, Electrochim. Acta, 2013, 112, 422–430 CrossRef.
- F. Chen, Q. Yang, C. Niu, X. Li, C. Zhang and G. Zeng, Plasmonic photocatalyst Ag@AgCl/ZnSn(OH)6: synthesis, characterization and enhanced visiblelight photocatalytic activity in the decomposition of dyes and phenol, RSC Adv., 2015, 5, 63152–63164 RSC.
- P. K. Khanna, N. Singh, S. Charan, V. V. V. S. Subbarao, R. Gokhale and U. P. Mulik, Synthesis and characterization of Ag/PVA nanocomposite by chemical reduction method, Mater. Chem. Phys., 2005, 93, 117–121 CrossRef CAS.
- P. K. Khanna, N. Singh, S. Charan and A. K. Viswanath, Synthesis of Ag/polyaniline nanocomposite via an in situ photo-redox mechanism, Mater. Chem. Phys., 2005, 92, 214–219 CrossRef CAS.
- M. A. S. Sadjadi, B. Sadeghi, M. Meskinfam, K. Zare and J. Azizian, Synthesis and characterization of Ag/PVA nanorods by chemical reduction method, Phys. E, 2008, 40, 3183–3186 CrossRef CAS.
- M. O. G. Perez, J. L. G. Fierro and M. A. Banares, Tunning the Nb addition to Sb–V–O catalysts for an efficient promotion of the ammoxidation of propane to acrylonitrile, Catal. Today, 2006, 118, 366–372 CrossRef.
- S. A. Mahapure, P. K. Palei, L. K. Nikam, R. P. Panmand, J. D. Ambekar, S. K. Apte and B. B. Kale, Novel nanocrystalline zinc silver antimonite (ZnAg3SbO4): an efficient & ecofriendly visible light photocatalyst with enhanced hydrogen generation, J. Mater. Chem. A, 2013, 1, 12835–12840 RSC.
-
R. Jenkins and R. L. Snyder, X-ray Powder Diffractometry. An Introduction, John Wiley & Sons, 1994, vol. 138, pp. 750–950 Search PubMed.
- B. Nithyaja, H. Misha and V. P. N. Nampoori, Synthesis of Silver Nanoparticles in DNA Template and Its Influence on Nonlinear Optical Properties, Nanosci. Nanotechnol., 2012, 2, 99–103 CrossRef CAS.
- N. Krithiga, A. Rajalakshmi and A. Jayachitra, Green Synthesis of Silver Nanoparticles Using Leaf Extracts of Clitoria ternatea and Solanum nigrum and Study of Its Antibacterial Effect against Common Nosocomial Pathogens, J. Nanosci., 2015, 1–8 Search PubMed.
- G. Geoprincy, P. Saravanan, N. N. Gandhi and S. Renganathan, A nobel approach for studying the combined antimicrobial effects of silver nanoparticles and antibiotics through agar over layer method and disk diffusion, Dig. J. Nanomater. Biostruct., 2011, 6, 1557–1565 Search PubMed.
- J. Baharara, F. Namvar, T. Ramezani, N. Hosseini and R. Mohamad, Green Synthesis of Silver Nanoparticles using Achillea biebersteinii Flower Extract and Its Anti-Angiogenic Properties in the Rat Aortic Ring Model, Molecules, 2014, 19, 4624–4634 CrossRef CAS PubMed.
- S. S. Patil, M. G. Mali, M. S. Tamboli, D. R. Patil, M. V. Kulkarni, H. Yoon, H. Kim, S. S. Al-Deyab, S. S. Yoonc, S. S. Kolekarb and B. B. Kale, Green approach
for hierarchical nanostructured Ag–ZnO and their photocatalytic performance under sunlight, Catal. Today, 2016, 260, 126–134 CrossRef CAS.
- W. Fan, S. Jewell, Y. She and M. K. H. Leung, In situ deposition of Ag–Ag2S hybrid nanoparticles onto TiO2 nanotube arrays towards fabrication of photoelectrodes with high visible light photoelectrochemical properties, Phys. Chem. Chem. Phys., 2014, 16, 676–680 RSC.
- Z. Chen, W. Wang, Z. Zhang and X. Fang, High-Efficiency Visible-Light-Driven Ag3PO4/AgI Photocatalysts: Z-Scheme Photocatalytic Mechanism for Their Enhanced Photocatalytic Activity, J. Phys. Chem. C, 2013, 117, 19346–19352 CrossRef CAS.
- F. H. Cincotto, T. C. Canevari, A. M. Campos, R. Landers and S. A. S. Machado, Simultaneous determination of epinephrine and dopamine by electrochemical reduction on the hybrid material SiO2/graphene oxide decorated with Ag nanoparticles, Analyst, 2014, 139, 4634–4640 RSC.
- J. K. Liang, H. L. Su, C. L. Kuo, S. P. Kao, J. W. Cuia, Y. C. Wua and J. C. A. Huang, Structural, Optical and Electrical Properties of Electrodeposited Sb-Doped ZnO Nanorod Arrays, Electrochim. Acta, 2014, 125, 124–132 CrossRef CAS.
- M. Ahmad, C. Pan and J. Zhu, Electrochemical determination of L-Cysteine by an elbow shaped, Sb-doped ZnO nanowire-modified electrode, J. Mater. Chem., 2010, 20, 7169–7174 RSC.
- S. Ren, C. Li, X. Zhao, Z. Wu, S. Wang, G. Sun, Q. Xin and X. Yang, Surface modification of sulfonated poly(ether ether ketone) membranes using nafion solution for direct methanol fuel cells, J. Membr. Sci., 2005, 247, 59–63 CrossRef CAS.
- Z. Wang, G. Liu, L. Zhang and H. Wang, Electrochemical detection of trace cadmium in soil using a Nafion/stannum film-modified molecular wire carbon paste electrodes, Ionics, 2013, 19, 1687–1693 CrossRef CAS.
- H. B. Balkhoyor, M. M. Rahman and A. M. Asiri, Effect of Ce doping into ZnO nanostructures to enhance the phenolic sensor performance, RSC Adv., 2016, 6, 58236 RSC.
- M. M. Rahman, H. B. Balkhoyor and A. M. Asiri, Phenolic sensor development based on chromium oxide-decorated carbon nanotubes for environmental safety, J. Environ. Manage., 2017, 188, 228–237 CrossRef CAS PubMed.
- S. Korkut, M. S. Kilic and E. Erhan, Modified poly(pyrrole) film based biosensors for phenol detection, Int. J. Chem. Mol. Nucl. Mater. Metall. Eng., 2015, 9, 439–442 Search PubMed.
- G. Scandurra, A. Antonella, C. Ciofi, G. Saitta and M. Lanza, Electrochemical detection of p-aminophenol by flexible devices based on multi-wall carbon nanotubes dispersed in electrochemically modified nafion, Sensors, 2014, 14, 8926–8939 CrossRef CAS PubMed.
Footnote |
† Electronic supplementary information (ESI) available. See DOI: 10.1039/c8na00034d |
|
This journal is © The Royal Society of Chemistry 2019 |