DOI:
10.1039/C9MT00177H
(Paper)
Metallomics, 2019,
11, 1805-1819
A metalloproteomic analysis of interactions between plasma proteins and zinc: elevated fatty acid levels affect zinc distribution†
Received
9th July 2019
, Accepted 12th September 2019
First published on 15th October 2019
Abstract
Serum albumin is a highly abundant plasma protein associated with the transport of metal ions, pharmaceuticals, fatty acids and a variety of small molecules in the blood. Once thought of as a molecular ‘sponge’, mounting evidence suggests that the albumin-facilitated transport of chemically diverse entities is not independent. One such example is the transport of Zn2+ ions and non-esterified ‘free’ fatty acids (FFAs) by albumin, both of which bind at high affinity sites located in close proximity. Our previous research suggests that their transport in blood plasma is linked via an allosteric mechanism on serum albumin. In direct competition, albumin-bound FFAs significantly decrease the binding capacity of albumin for Zn2+, with one of the predicted consequences being a change in plasma/serum zinc speciation. Using liquid chromatography (LC), ICP-MS and fluorescence assays, our work provides a quantitative assessment of this phenomenon, and finds that in the presence of high FFA concentrations encountered in various physiological conditions, a significant proportion of albumin-bound Zn2+ is re-distributed amongst plasma/serum proteins. Using peptide mass fingerprinting and immunodetection, we identify candidate acceptor proteins for Zn2+ liberated from albumin. These include histidine-rich glycoprotein (HRG), a multifunctional protein associated with the regulation of blood coagulation, and members of the complement system involved in the innate immune response. Our findings highlight how FFA-mediated changes in extracellular metal speciation might contribute to the progression of certain pathological conditions.
Significance to metallomics
Building on previous evidence from single-protein model systems and computational simulations, this work provides a metalloproteomic assessment of serum/plasma zinc speciation, and identifies a significant re-distribution of Zn2+ amongst serum/plasma proteins, as a consequence of increased free fatty acid levels. Fluctuations in both serum free fatty acids and serum zinc have been independently associated with a plethora of diseases. This work now enhances our understanding of how metalloproteomic approaches are vital in order to understand the bio-inorganic mechanisms at play. Moreover, our work also suggests how metal ion speciation might even be affected in normal physiology, such as after the consumption of food or intense aerobic exercise.
|
Introduction
The importance of zinc as an essential micronutrient for all life forms is well recognised. Increases of between 30–600% in crop yields have been achieved solely by zinc fertilisation,1 as well as dietary zinc supplementation being identified as a top priority by the 2008 Copenhagen Consensus Conference for 80% of the world's 140 million malnourished children.2 As a Type II nutrient,3 zinc directly affects multiple physiological processes with deficiency leading to growth retardation, skin lesions, diarrhoea, infertility, and compromised immune4 and cognitive function.5 Milder zinc deficiency is also a risk factor for cardiomyopathy and myocardial infarction.6 The pervasive effects of inadequate zinc supply occur not only because of the essentiality of zinc for the function of hundreds of enzymes and thousands of transcription factors,7,8 but also because zinc is a signalling agent, and mediates and modulates bio-molecular interactions.9
Considering these multiple effects, it is perhaps at first surprising that to plants and animals including humans, excess zinc is not particularly toxic. However, the picture of zinc's apparent biological harmlessness disintegrates once the effects of elevated free Zn2+ concentrations on individual cells are considered, as low micromolar concentrations are sufficient to induce apoptotic or necrotic cell death pathways in a variety of cell types.4,10 Many unicellular organisms are also highly sensitive to Zn2+.11,12 It follows that for higher eukaryotes, zinc toxicity is largely avoided by careful control of gastrointestinal tract absorption and – crucially – regulation of extracellular free Zn2+,13–15 ensuring its concentrations remain below the levels at which it would begin to exert any cytotoxic effects.
It is well known that human blood plasma or serum contain significant amounts of zinc (9.6–31.6 μM),16,17 alongside similar quantities of iron (6.05–26.96 μM)18 and copper (17 ± 6 μM),19 whilst only low-nanomolar amounts of manganese, cobalt and molybdenum are present.20–22 Assuming a typical total amount of body zinc of 2.7 g, and a plasma volume of 2.5 L, it can be estimated that less than 0.2% of total body zinc is in the blood plasma. Despite this minor proportion, and although plasma/serum zinc levels are not reliable indicators of total organismal zinc status,23 plasma zinc speciation and dynamics should not be disregarded, as it may not only influence overall organismal zinc distribution, but also modulate important bio-molecular interactions in the bloodstream.24,25 It is also important to note that although the levels of total plasma zinc are usually maintained at fairly constant levels, there are a number of conditions where they are significantly decreased, including during the acute phase response to infections and inflammation,26,27 and in response to stress, for example during surgery.24 There are also a number of chronic conditions that are associated with low plasma levels of zinc, including asthma and type 2 diabetes.6,25 The molecular causes for the latter observations are not well understood.28
Along with a great many studies on total metal contents of plasma and sera,29 research concerning Zn2+–protein interactions that dominate plasma zinc speciation has been ongoing for several decades.30–34 Serum albumin, a 66 kDa globular protein (Fig. 1), is the most abundant serum protein (0.6 mM), has high-nanomolar to low-micromolar affinity for Zn2+ and is the major carrier of plasma zinc.35,36 Metal binding occurs at an inter-domain site involving tetrahedral metal coordination by His67, His247, Asp249 and a fourth non-protein ligand such as a water molecule (site A; Fig. 1c).37 Under normal conditions, albumin is the dominant Zn2+ chelator, binding between 75–90% of the total plasma zinc.14 Albumin-bound Zn2+ constitutes the largest part of the so-called exchangeable zinc pool (EZP), which comprises up to 90% of the total plasma zinc. Albumin is also known to transport various other small molecules, including pharmaceuticals,38,39 various hormones, bilirubin, and free (non-esterified) fatty acids (FFAs).40 The binding of long-chain FFAs to albumin (Fig. 1b) has been demonstrated to decrease the binding affinity of albumin for Zn2+.41 The underlying molecular mechanism (Fig. 1) has been studied in depth using X-ray crystallography,37,42,43 molecular modelling, NMR spectroscopy,44 and isothermal titration calorimetry,41,45 and is now well-understood in chemical model systems.46 Interdependence of Zn2+ and FFA binding is due to the proximal location of the primary Zn2+ binding site (site A) and one (out of three;47Fig. 1b) high affinity fatty acid binding site (FA2), both situated at the interface of domains I and II of albumin. Upon fatty acid binding, providing the fatty acid is of sufficient chain length, a ‘spring-lock’ allosteric switching mechanism is invoked in albumin, disengaging amino acids involved in Zn2+ coordination at site A, resulting in a significant decrease in metal binding affinity, and hence this otherwise major binding site is no longer available to Zn2+.48 In FA2, FFAs bind with their carboxylate headgroup to Arg257 in domain II. The allosteric switch is elicited by FFAs with 10 or more carbon atoms,42 whilst no analogous X-ray crystal structures with bound octanoate are available. Molecular modelling had suggested that octanoate may fit into the half-pocket in domain II, without the need to align the half-pocket in domain I.41 The affinities of the three high-affinity sites cannot be distinguished; therefore, all three sites become occupied simultaneously.47 Consistent with this finding, our previous work has shown that myristate affects the Zn2+ affinity of both BSA41 and HSA45 already at 1 molar equivalent (mol. eq.). The latter concentration is within a normal physiological range (0.1–2 mol. eq.), but FFA levels can rise to 4–6 mol. eq. in a range of conditions (strenuous exercise, diabetes, cardiovascular disease, non-alcoholic fatty liver disease).47
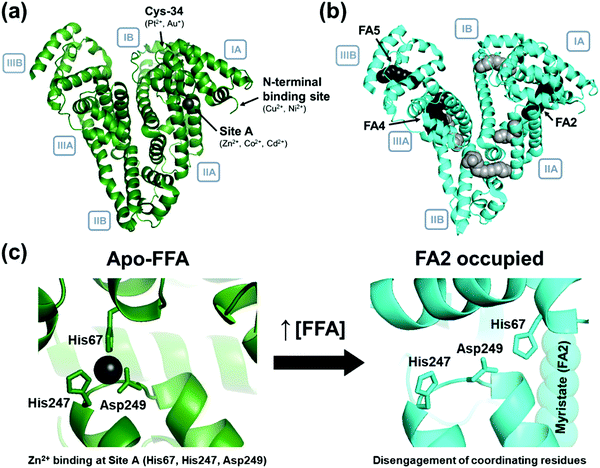 |
| Fig. 1 Serum albumin is a key transporter of metal ions and ‘free’ fatty acids (FFAs) in the blood plasma of all mammals. (a) Four prominent metal ion binding sites have been identified on albumin: site A, Cys34, the N-terminal binding site (NTS) formed by an ATCUN (amino-terminal copper- and nickel-binding) motif, and site B (location unknown); (b) seven free fatty acid (FFA) binding sites have been identified by X-ray crystallographic studies of albumin complexed with palmitic (hexadecanoic) acid (PDB 1E7H). The three high affinity sites47 are identified with arrows. (c) Coordination of a free fatty acid (FFA, myristate; PDB 1BJ5) at site FA2 leads to disengagement of His67, His247 and Asp249 from metal binding at site A (PDB 5IJF), preventing coordination of Zn2+.37,49 | |
As a result of this finding, it has been suggested that fluctuations in the levels of FFAs in the blood may impact zinc speciation, with several downstream effects predicted.14,46,48 Although cellular zinc uptake and compartmentalisation is largely governed by membrane-bound transporters of the ZIP and ZnT families,50,51 it is conceivable that plasma speciation could impact the uptake of Zn2+ by endothelial cells and blood cells, including leukocytes involved in immune function.13 In this regard, the effects of zinc on immune cells are of high relevance, since zinc is required for both innate and adaptive immune response, modulating cytokine secretion,52 and playing roles in T cell maturation53 and B cell response to vaccination.54
For these reasons, it is of interest to study the effect of FFAs on zinc speciation in plasma. The most basic and rapid separation method to address zinc speciation in serum or plasma is ultrafiltration,55–57 which allows for distinction between protein-bound Zn2+ (the high-molecular weight fraction, including serum albumin) from both ‘free’ (aquated) Zn2+ and Zn2+ bound to small ligands, such as free amino acids. The low-molecular weight fraction does usually not exceed 1% of total serum or plasma zinc, for which a typical free Zn2+ concentration has been estimated at around 2–4 nM, using the Zn2+-responsive dye ZnAF-2.58 The high-molecular weight fraction also consists of non-exchangeable zinc, bound firmly to proteins such as α2-macroglobulin (ca. 10–20% of total plasma zinc) and retinol-binding protein.59 It is also worth noting that the concentrations of zinc-dependent enzymes (which are also expected to mostly contain non-exchangeable zinc) present in plasma are so low that so far, they have mostly escaped quantitation.
The baseline numbers given above, both in terms of total plasma or serum zinc as well as in terms of speciation, do not reflect the fairly dramatic changes in overall organismal zinc distribution, or the possibility for re-distribution between different plasma proteins under abnormal conditions. Speciation studies involving metal–protein complexes have recently been encompassed under the term “metalloproteomics”, which aims to combine established proteomic techniques with complementary methods for analytical detection of metal ions. These typically comprise separation (most commonly chromatography or electrophoresis) and identification (often mass spectrometry) steps. Techniques such as SDS-PAGE, western blotting, and more recently native SDS-PAGE,60 have been combined with elemental analysis, using sensitive techniques including inductively-coupled plasma mass spectrometry (ICP-MS) and ICP-OES (optical emission spectroscopy) to identify and quantify metal ions.61,62 Further methodological refinements include the use of laser ablation (LA-ICP-MS) as well as isotope quantification in biological systems.63–66 Hyphenated liquid chromatography methods, including capillary and nanoflow HPLC,63 have attracted attention owing to the relative ease and speed of sample throughput in combination with low-nanomolar quantitation limits associated with ICP-MS. Serum metalloproteomes have been previously investigated using mass spectrometry to identify both metal ions (ICP-MS) and tens of metal-binding serum proteins (LC-MS/MS).67 ICP-MS has also been used to inspect variations in the levels of free metal ions in serum obtained from patients with bipolar disorder undergoing different treatments.68 Other researchers have employed size exclusion chromatography (SEC) paired with ICP-MS/AES to study metal binding to plasma proteins,69–73 and the effect of chelating agents on plasma metal speciation.74,75
In this study, we have also utilised SEC in conjunction with offline ICP-MS analysis to investigate zinc speciation changes in foetal calf serum (FCS) and human blood plasma, upon addition of free fatty acids (FFAs). By administering controlled concentrations of fatty acids to human blood plasma (and a simple model systems), we show how FFAs are able to modulate extracellular zinc speciation in a quantitative manner. Immobilised metal affinity chromatography (IMAC) was employed as a complementary technique to identify key Zn2+-binding proteins in plasma samples which may be involved in such changes in zinc speciation.
Methods
Materials
Ammonium acetate (99.999% trace metals basis), ammonium hydroxide (28% NH3 in H2O, ≥99.99% trace metals basis), bovine serum albumin (low endotoxin, lyophilized powder, BioReagent, suitable for cell culture, ≥98% by agarose gel electrophoresis), sodium myristate and sodium octanoate were purchased from Sigma Aldrich UK. Foetal calf serum (FCS, lot 70428, heat-inactivated, non-USA origin) was purchased from Labtech International UK, and a single lot was used throughout all experiments. Citrated human plasma was purchased from TCS Biosciences UK. Inorganic Ventures ICP-MS standards for Zn (1000 ppm) and Er (1000 ppm) were purchased from Essex Scientific Supplies UK. Nitric acid (72% v/v) was freshly distilled before use. Water used for all experiments was doubly deionised (Type-I) Milli-Q water. All other reagents were purchased from Sigma Aldrich UK and used as received, unless specified otherwise.
Size exclusion chromatography (FPLC)
Experiments were carried out using a GE Healthcare ÄKTA purifier 10 FPLC fitted with a Superdex G-75 16/60 size exclusion column (13 μm average particle size, optimum separation range 3000–70
000, column volume 120 mL), Frac-950 fraction collector, pH/C probe 900, UV900 detector, and a P-900 pump, with ammonium acetate buffer (50 mM, pH 7.8 ± 0.1, adjusted using ammonium hydroxide) mobile phase (277 K). A flow rate of 1 mL min−1 and an injection volume of 0.5 mL was used. All samples were prepared at physiological concentrations (600 μM BSA + 20 μM Zn2+ in 50 mM ammonium acetate buffer pH 7.8, or neat serum), incubated for a minimum of 18 h at 310 K before analysis, and diluted 2-fold in ammonium acetate buffer before filtration using a 0.22 μm syringe filter. Samples were analysed in triplicate. FPLC data were acquired and analysed using UNICORN 5.11 for Windows. Experiments were repeated with the following modifications: (i) bovine serum albumin (BSA) was prepared with 0–5 mol. eq. fatty acid: octanoate (C8) or myristate (C14). Solutions of (ii) foetal calf serum and (iii) human citrated plasma were used in place of BSA, with and without FFAs, but no additional zinc. Natively present zinc was quantified separately using ICP-MS prior to use. Chemical concentrations in final samples were 300 μM BSA (or 50% of FCS or human plasma, as appropriate), 10 μM Zn2+, 0–1.5 mM (0–5 mol. eq.) FFA.
Inductively-coupled plasma mass spectrometry (ICP-MS)
Zinc concentrations were determined using an Agilent 7900 series ICP-MS in He-gas mode with an internal standard of 166Er (Agilent Technologies, USA). Calibration standards (1–1000 ppb) were freshly prepared in either ammonium acetate (50 mM) buffer or 3.6% ultrapure nitric acid, depending on the nature of the samples in order to match the sample matrix. Data were acquired and analysed using MassHunter 4.3 (Agilent Technologies, USA).
Monitoring levels of non-protein bound Zn2+ concentration using FluoZin-3
Briefly, a 96-well plate was washed thoroughly using 50 μL of EDTA solution (50 mM) per well, followed by washing using Milli-Q (Type I) water (8 × 200 μL) and thoroughly dried. For the experiments involving fatty acids, two BSA concentrations were employed (60 and 600 μM). Solutions of de-metalated BSA (pre-treated with Chelex-100 resin to remove any potential traces of Zn2+) were prepared (1.2 mM or 120 μM, 2× final concentration) in ammonium acetate buffer (50 mM, pH 7.8) in the presence and absence of sodium myristate or sodium octanoate (1, 3 or 5 mol. eq.). The pH was adjusted to 7.8 using ammonium hydroxide. After 24 h incubation (310 K), 100 μL aliquots were added per well to a 96-well plate. Separately, an 80 μM (4× final concentration) solution of Zn2+ (from ZnSO4) was prepared in ammonium acetate buffer (Zn2+ concentration determined by ICP-MS). To each BSA-FFA condition was added either 50 μL of Zn2+ (80 μM) or 50 μL of buffer (0 μM Zn2+). Calibration standards for Zn2+ (0.001–40 μM) were separately prepared in 50 mM ammonium acetate buffer (150 μL), in the absence of BSA. To each well FluoZin-3 solution (50 μL; 0.1–2 μM, final concentration) was then added, to give a final volume of 200 μL. Final working concentrations of samples were 60–600 μM BSA, 0–20 μM Zn2+ and 0–3 mM (up to 5 mol. eq.) FFA. Individual compositions are given in the respective Figure captions. All samples were prepared in triplicate. After 30 min incubation at 310 K, fluorescence was measured using a Promega GloMax fluorescence microplate reader (blue optical kit, reagent Ex/Em = 494/516 nm). Data were analysed and processed using Microsoft Excel. Arithmetic means and standard deviations were calculated, and statistical significance was evaluated using a two-tailed t-test assuming non-equal variances (Welch's t-test).
Albumin quantitation using bromocresol green
Albumin quantitation of FCS and human citrated plasma was carried out using a commercial bromocresol green (BCG) colorimetric assay kit (Abcam, ab235628) according to the manufacturer's instructions. Samples were diluted 1
:
100 in assay buffer before analysis and read using a Promega GloMax microplate reader (absorbance at 620 nm).
Determination of fatty acids by GC-MS
Fatty acids were extracted from FCS using chloroform
:
methanol (2
:
1 v/v, 1 mL reagent per 100 μL serum) with vortexing, as described by the Folch method.76 The layers were separated using centrifugation, and the chloroform layer was evaporated to dryness. Lipid samples were dissolved using 90 μL of chloroform
:
hexane (1
:
1, Folch extractant) and derivatized with 10 μL Meth-Prep II (methanolic m-trifluoromethyl-phenyltrimethylammonium hydroxide) at room temperature for 30 min. Samples were analysed using an Agilent Technologies GC-MS (7890B GC – 5977B MS), operated in EI mode (70 eV), fitted with an Agilent 19091S-433, HP-5MS (5% phenyl methyl siloxane) 30 m × 250 μm × 0.25 μm column with helium carrier gas. Temperature gradient: initial: 50 °C (1 min), increased to 280 °C at a rate of 20 °C min−1, then 7.5 min hold time. Total run time: 20 min.
Immobilized metal affinity chromatography (IMAC)
1 mL IMAC FF columns (packed with IMAC Sepharose Fast Flow resin; GE Healthcare, UK) were prepared as described by the manufacturer and either charged with Zn2+ or left un-charged. Columns were equilibrated with 10 mM HEPES (pH 7.2, 0.5 M NaCl) ready for application of plasma. Human citrated plasma was clarified by centrifugation at 12
000 × g for 5 minutes and the supernatant was passed through a 0.22 μm filter. 1 mL of clarified plasma was applied to each column and the flow-through (FT) fraction was collected. Unbound protein was washed through the column using 6 × 1 mL of buffer containing 2 mM imidazole, before bound proteins were eluted using 2 × 1 mL of 20 mM imidazole buffer and 2 × 1 mL of 200 mM imidazole buffer.
SDS-polyacrylamide gel electrophoresis
Samples from SEC and IMAC fractions were mixed with an equal volume of (2×) Laemmli buffer (Sigma-Aldrich) and heated to 80 °C for 5 minutes before loading onto mini-Protean® TGX™ precast gels (4–15% resolving gel percentage; 20–250 kDa separation range; Bio-Rad, UK),77 which were run in standard Tris-glycine buffer following the manufacturer's instructions. Gels were stained using Coomassie brilliant blue R-250 (National Diagnostics, USA).
Western blotting
Proteins were transferred onto a nitrocellulose membrane using a semidry blotting assembly (90 min, 150 mA). Non-fatty milk solution (2.5 g of non-fatty milk powder in 50 mL of Tris-buffered saline with 0.1% Tween 20; TBST) was used as blocking reagent (1 h at 277 K). The membrane was washed with TBST buffer before adding Anti-HRG primary antibody (produced in rabbit, Sigma-Aldrich, 10 μg mL−1 in 20 mL TBST buffer, 1 hour). After further washing with TBST buffer, 20 mL solution of anti-rabbit IgG alkaline phosphatase conjugate secondary antibody (Sigma-Aldrich) was added and incubated for 1 h. The membrane was washed three times with TBST buffer after every 5 min of rocking. Finally, 2 mL of Novex® AP chromogenic substrate (Invitrogen) was added and incubated for 15 min. After rinsing with water, gels were imaged using an ImageQuant LAS4000 Blot Imaging System (GE Healthcare Life Sciences, Pittsburgh, USA).
Peptide mass fingerprinting
Protein bands of interest were cut from SDS-PAGE gels with a scalpel blade and were subjected to in-gel tryptic digestion using a commercially produced kit (Pierce, Thermo Scientific, UK). The masses of the generated peptides were determined by MALDI-TOF MS analysis. Briefly, 2 μL of sample matrix (10 mg mL−1 α-cyano-4-hydroxycinnamic acid in 50% acetonitrile, 0.1% trifluoroacetic acid) was mixed with an equal volume of sample and pipetted onto a steel MALDI-target plate. A Bruker Ultraflex II MALDI-TOF/TOF mass spectrometer (Bruker Daltonics, UK) with a 337 nm laser and operated in reflectron mode was used to measure the peptide masses. Mass calibration was performed with a PEG2000 MALDI-MS standard, and mass accuracy was verified using Bradykinin and Substance P peptide standards. Internal mass accuracy was further confirmed with the presence of the autolytic trypsin peaks of 845.2 and 2211.1 Da. Peptide masses were acquired over the range of 800–3500 Da, and mass lists were generated using Bruker Flex-analysis software with default parameters, and searched against the NCBI database using Mascot (Matrix Science, UK). The following search criteria were selected: fixed modification of carbamidomethyl on cysteine, variable modification of oxidation of methionine, maximum of 1 missed cleavage, <50 ppm mass accuracy, “Homo sapiens” was selected for taxonomy. Only searches giving significant MOWSE (MOlecular Weight SEarch) scores were recorded.
Results and discussion
Zinc speciation in serum and plasma is affected by free fatty acids (FFAs)
Zinc speciation methodology was first optimised using a simplified single-protein system containing bovine serum albumin (BSA), in the absence of FFAs. A Superdex G-75 16/60 FPLC-SEC column was employed for size exclusion chromatography, with the selection of a mobile phase which was compatible with both SEC under near-native conditions and direct elemental analysis by ICP-MS (50 mM ammonium acetate, pH 7.8). Physiological concentrations of BSA (600 μM) and Zn2+ (20 μM) were analysed after 2-fold dilution to ensure sufficiently high concentrations of metal ions were present in the eluent for quantitation by ICP-MS whilst minimizing deposition of non-volatile salts on the sampling cone in the subsequent experiments with serum and plasma. The method was shown to have high reproducibility in both FPLC-SEC (A280
nm) and ICP-MS (66Zn) datasets (Fig. S1 and S2, ESI†), with 109.9% recovery of protein-bound Zn2+ from the original 20 μM sample. Data were then acquired in the presence of two different FFAs, octanoate (C8) and myristate (C14). In both instances, the chromatography traces for protein (A280
nm) did not statistically differ from the FFA-free control, suggesting that BSA elution (or later, serum protein elution) was not significantly affected by the presence of FFAs (Fig. S3, ESI†). However, ICP-MS analysis of SEC fractions determined that 5 mol. eq. of myristate significantly decreased the amount of albumin-bound Zn2+ by 69.9% (from 20 μM to 6.02 μM) relative to the FFA-free control (Fig. 2a). In contrast, 5 mol. eq. of octanoate had no significant effect on the concentration of Zn2+ in albumin-containing fractions (Fig. S4, ESI†). This is in agreement with previous crystallographic72 and molecular modelling studies,41 which suggested that FFAs with a chain length ≤C8 are too short to elicit the allosteric switch on albumin. Previous ITC experiments had confirmed that octanoate indeed did not affect the Zn2+-binding capacity of BSA.41 In contrast, site A in both BSA and HSA45 was demonstrated to be essentially absent in the presence of 5 mol. eq. of myristate. Residual weak Zn2+-binding capacity was still observed from a secondary Zn2+-binding site on both bovine (K2(ITC) ≈ 1.4 × 104 M−1; ionic strength = 95 mM) and human albumin (K2(ITC) ≈ 3 × 103 M−1; ionic strength = 183 mM), assumed to be site B based on previous 111Cd NMR competition studies.41,78 A possible secondary Zn2+ binding site has been observed by crystallography of equine serum albumin, involving His9, Asp13 and Asp254, all of which are fully conserved in HSA and BSA. This, in principle, justifies the observation of a ca. 70% reduction in albumin-bound Zn2+. It must also be considered that the composition of our buffer (50 mM ammonium acetate) has significantly lower ionic strength (<50 mM) than either previously employed experimental conditions or physiological media (>100 mM), which is expected to increase complex stability and hence the proportion of Zn2+ bound to BSA in our model system used for offline LC-ICP-MS.
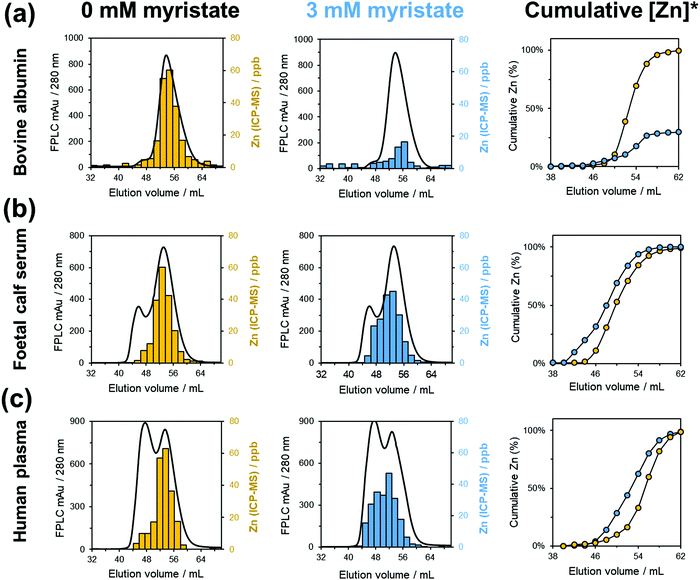 |
| Fig. 2 Offline SEC-ICP-MS (Superdex G-75 16/60 FPLC) analysis demonstrates that sodium myristate (3 mM, 5 mol. eq.) impacted zinc speciation in: (a) bovine serum albumin, where the Zn2+ binding capacity of BSA is significantly reduced, resulting in decreased cumulative protein-bound zinc. For both foetal calf serum (b) and human citrated plasma (c), cumulative protein-bound zinc was not significantly affected, but instead, zinc was re-distributed amongst other plasma/serum proteins with Zn2+ binding capability. *Protein-bound zinc only. The black lines in the chromatograms refer to protein elution, monitored by UV absorption at 280 nm, whilst the yellow and blue bars refer to zinc concentrations measured by ICP-MS. In the right-hand plots, black lines connecting the experimental data are drawn to guide the eye. | |
Foetal calf serum (FCS) is a common component of extracellular media for the culture of in vitro cell lines, and provides a more complex system for chromatographic analysis. First, the albumin content of FCS was quantified using bromocresol green, (345 ± 65 μM). This is lower than physiological albumin levels in adult humans (∼600 μM); however the comparable total zinc concentrations of FCS and plasma (25.8 ± 0.3 μM and 21.4 ± 0.4 μM, respectively) mean that albumin is in excess to zinc in both instances. Size exclusion chromatography experiments were repeated using FCS in place of bovine albumin. In this complex medium, five mol. eq. of myristate did not impact the cumulative recovery of zinc relative to the initial sample (i.e. no ‘free’ Zn2+ released, unlike in the BSA model system). Rather, the presence of myristate invoked a re-distribution of zinc, predominantly to higher molecular weight (lower elution volume) species (Fig. 2b). Data obtained in the presence of sodium octanoate (C8) in FCS confirm that octanoate did not impact either the distribution of serum zinc or cumulative zinc recovery (Fig. S4, ESI†). Thus, for both short-chain and long-chain fatty acids, the system BSA + fatty acid + Zn2+ behaves in a similar way in this complex medium (FCS) as in the pure ternary mixture, with the main difference being the destination of the Zn2+ liberated from albumin.
Direct comparison of data acquired for BSA and FCS in the absence of myristate indicates that Zn2+ in FCS is not solely bound by albumin, with ca. 12.6% eluting together with higher molecular weight proteins. This is in line with expectations, as the known Zn2+-binding protein α2-macroglobulin has been identified in commercial preparations of foetal calf serum.79 It is also important to note that FCS already contains native FFAs. After chloroform:methanol extraction and derivitisation of FFAs using a methanolic solution of m-trifluoromethylphenyltrimethylammonium hydroxide, GC-MS analysis principally identified palmitic (C16:0), stearic (C18:0) and oleic acids (C18:1) in FCS (Fig. S5, ESI†), all of which are known to bind to FA2.42,80 One must therefore consider that the fatty acid binding sites of albumin are likely to be already partially occupied, and this may further contribute to the wider distribution of Zn2+ relative to the pure binary albumin + Zn2+ system. As a further consequence, the redistribution observed after in vitro FFA supplementation may be less dramatic, compared to the model system involving (FFA-free) BSA. However, in FCS, Zn2+ is likely to be bound to other proteins that happen to also elute in the same fractions as albumin, which could not be resolved by the SEC column.
The concentration of non-albumin bound Zn2+ in eluates from the simple BSA + myristate model system was not quantifiable by SEC-ICP-MS because the released Zn2+ did not elute in the small-molecule fraction. However, repeated elution of the Superdex column using EDTA (20 mM) demonstrated significant stationary-phase retention of Zn2+ (Fig. S6, ESI†). The data shown in Fig. 2 were obtained after rigorous pre-cleaning of the column with 20 mM EDTA, which enabled reasonable recovery (110%) of zinc in the absence of myristate. Ideally, speciation analysis should provide quantitation across all species, hence an alternative method to capture non-protein-bound zinc in the various systems was desirable.
Monitoring Zn2+ release from albumin by FluoZin-3
Attempts were made to quantify the proportion of non-albumin bound zinc in presence and absence of myristate using a fluorescent Zn2+ sensing reagent (FluoZin-3; FZ3 in the following discussion). It was hoped that this approach would allow a measure of fatty-acid induced release of Zn2+ with minimal perturbation of the system. FZ3 was chosen as its affinity is relatively close to that of albumin (the conditional dissociation constant of FZ3 at pH 7.4 has recently been refined, Kd = (9.1 ± 0.4) × 10−9 M),81 and FZ3 has high selectivity for Zn2+ over other divalent metal ions such as Ca2+ and Mg2+.82 Since fluorescent sensors are typically employed at concentrations that are much lower than those of surrounding proteins,83–85 the influence of the sensor on the original equilibrium should be relatively small. Other Zn2+ sensors were considered, but rejected based on their documented tendency to form ternary complexes with Zn2+ and proteins.85–87
The response of either 0.1 or 2 μM FZ3 to [Zn2+] was recorded in ammonium acetate buffer using a range of defined Zn2+ concentrations (Fig. S7, ESI†), before exploring the effect of BSA concentration on FZ3 fluorescence (Fig. S8, ESI†). We found that BSA promoted FZ3 fluorescence in the absence of Zn2+; this suggested that BSA had the ability to bind FZ3. Indeed, fluorescein and related xanthene derivatives have previously been shown to bind to albumin;88–90 thus analogous interactions with the structurally similar fluorescent moiety in FZ3 are unsurprising. In the presence of Zn2+, the fluorescence decreased with increasing BSA concentration. This trend was expected, as increasing [BSA] should decrease the amount of free [Zn2+]. However, the fluorescence at 600 μM BSA and 2 μM FZ3 in presence and absence of 20 μM Zn2+ was identical (Table S1, ESI†), indicating that under these conditions, FZ3 was not able to report on Zn2+, free or protein-bound. At lower BSA concentrations, readings also deviated significantly from estimates based on published equilibrium constants, most likely due to the formation of ternary complexes and also FZ3 abstracting BSA-bound zinc, which becomes significant at lower [BSA] (Fig. S9, ESI†). Hence, due to the number and complexity of the various equilibria and processes in operation, the recorded fluorescence response could not be linked in a systematic manner to free [Zn2+]. Our observations are consistent with those of others who have highlighted the pitfalls in quantitation of free [Zn2+] using fluorescent dyes such as Zinquin,86,87 TSQ,86 ZnAF dyes,91 and indeed FluoZin-3.92 Problems have been attributed to ternary complex formation and other interactions with proteins86,87 and low-molecular weight components of physiological media.91,92 Our observations and the latter study suggest that the previously cited low nanomolar free [Zn2+] determined for plasma58 should be revisited.
Although the presence of BSA thus hindered the use of FZ3 to quantify non-protein bound Zn2+ in absolute terms, the effect of fatty acids was studied at lower BSA (60 μM) or FCS (10%) concentrations, employing the higher FZ3 concentration (2 μM), which was found to leave sufficient free FZ3 to respond to liberated Zn2+ (Fig. 3). FFAs were also confirmed to not affect background FZ3 fluorescence (Fig. S10, ESI†). Most importantly, there was a clear trend for myristate-induced Zn2+ release in the simple systems with BSA that was not observed with octanoate, essentially mirroring the observations from offline SEC-ICP-MS (Fig. 2). Myristate-induced Zn2+ release was also observed for the FCS systems. This indicates that FZ3 is able to also compete with the ‘Zn2+ acceptor’ proteins apparent in the SEC data. Given that the FZ3 concentration (2 μM) and affinity for Zn2+ are both expected to be higher than those for most of these proteins, this observation is qualitatively in line with expectations. In FCS, unlike for BSA alone, fluorescence was also increased by the addition of octanoate (Fig. 3). We suggest that this is likely due to the presence of intrinsic FFAs in serum (Fig. S5, ESI†), which may be re-distributed amongst the 7 principal FFA binding sites of albumin, upon addition of further equivalents of FFA. This, in turn, may lead to the (partial) population of site FA2 by longer-chain intrinsic FFAs, leading to the release of Zn2+ from site A. We also note that fluorescence readings for the 10% FCS systems are higher than for the 60 μM BSA systems throughout, in line with the significantly lower BSA concentration in FCS (ca. 35 μM, according to quantitation with bromocresol green; vide supra).
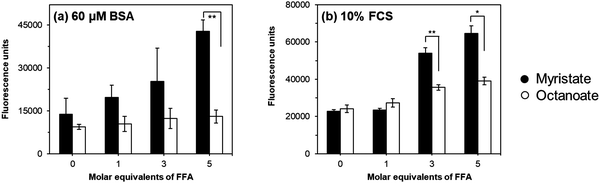 |
| Fig. 3 Measurement of FluoZin-3 (2 μM) fluorescence in the presence of either: (a) 60 μM BSA + 20 μM Zn2+ or (b) 10% FCS; with additional free fatty acids (FFAs). Myristate (black) increases the fluorescence of FluoZin-3, in contrast to the short-chain fatty acid octanoate (white), which had no significant effect on fluorescence. Statistics carried out using a two-tailed t-test assuming unequal sample variance (Welch's t-test), *P < 0.05, **P < 0.01. See ESI† for full statistical analysis. | |
The potential Zn2+ acceptor protein HRG is present in SEC fractions with FFA-promoted increases in [Zn]
Albumins from different species are known to bind Zn2+ at site A with subtle variance in their affinities, and published values concerning the differences in the Zn2+ affinity of human and bovine albumins are often conflicting, though both are generally accepted to be in the sub-micromolar range.93,94 Given the wide-ranging involvement of both zinc and fatty acids in various pathological diseases in humans, not limited to Type 2 diabetes,25 coronary heart disease,6 thrombosis,45 and Alzheimer's disease,95,96 we also investigated the impact of FFAs on zinc speciation in human citrated plasma, containing human serum albumin (HSA), using the previously described methodology. Using SEC and ICP-MS, a similar re-distribution of zinc to larger human plasma proteins with Zn2+-binding capability (smaller elution volume) was clearly apparent, analogous to our findings in FCS (Fig. 2c). It was therefore of interest to identify such proteins, and identify target molecules which (a) display significant Zn2+-binding ability under physiological conditions, and (b) are larger than serum albumin (i.e. have shorter retention times in size exclusion chromatography). Plasma is known to contain tens of thousands of proteins,97 and while the resolution of our SEC ICP-MS method is sufficient to clearly demonstrate a re-distribution of zinc to protein(s) of higher molecular weight, it is not sufficient to directly identify individual (zinc-binding) proteins, as seen by two broad bands in the chromatograms of both FCS and human plasma (Fig. 2). Complementary techniques (SDS-PAGE, western blotting) were therefore employed as a further step of protein identification in the eluent fractions.
Previous work from our team has suggested that such re-distribution may involve human histidine-rich glycoprotein (HRG), which has the capacity to bind up to ten Zn2+ ions (K = 1.63 × 105).45 In human plasma, HRG is present at low micromolar (1.3–2.0 μM) concentrations.98 The ca. 72 kDa serum protein exists as a native dimer and was successfully identified in elution volumes 43–53 mL of human plasma from size exclusion fractions by western blotting and immunodetection using HRG-specific antibodies (Fig. 4; 66 kDa albumin eluted predominantly between 49 and 61 mL). HRG is principally involved with the regulation of blood coagulation, but is also associated with angiogenesis and cell proliferation.98 The histidine-rich region (HRR) of HRG is known to complex Zn2+, and upon Zn2+ coordination, the affinity of the HRG-Zn2+ complex for heparin (a well-known anticoagulant) is known to increase.99 For this reason, we suggest that zinc speciation may be the ‘missing link’ between blood clotting disorders and elevated levels of fatty acids (leading to the re-distribution of Zn2+ from albumin to proteins associated with blood clotting, affecting their function).100 The greater affinity of the Zn2+-HRG complex for heparin prevents heparin from inhibiting clot formation,101 and hence Zn2+ is an important regulator of heparin neutralisation.102 Though HRG detection does coincide with fractions associated with increased [Zn2+] after redistribution of plasma zinc, we emphasise that HRG is only one of potentially hundreds of possible acceptor proteins in the aforementioned fractions. It is quite likely that many other proteins in these fractions also have affinity for Zn2+. Summarily, it is evident that in human plasma, like in FCS, Zn2+ ions liberated from albumin remain largely protein-bound, rather than occurring as ‘free’ aquated ions or in complexes with small molecules such as histidine or glutathione.
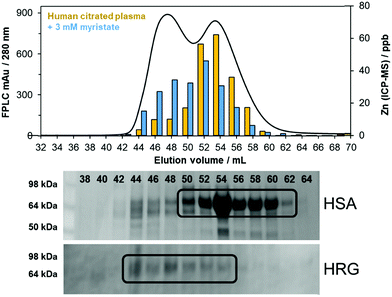 |
| Fig. 4 Human serum albumin (HSA, 66 kDa) identified in fractions 49–61 mL by SDS-PAGE (Coomassie staining), and histidine-rich glycoprotein (HRG, 72 kDa) in fractions 43–53 mL, identified by western blotting and immunodetection using specific HRG antibodies. Data for protein content (A280) and zinc distribution (in the presence and absence of myristate) obtained using SEC-ICP-MS have been overlaid using data from Fig. 2c. | |
Immobilized metal ion affinity chromatography to identify Zn2+ binding proteins
As indicated, 1D-size exclusion chromatography cannot resolve and identify single proteins which may participate in the re-distribution of Zn2+ from albumin. We therefore looked to a complementary chromatographic method: immobilized metal ion affinity chromatography (IMAC). This technique requires surface-exposed metal sites, and so should be ideally suited to capture proteins with sites that are involved in the “exchangeable zinc pool”. Proteins from unfractionated human plasma were captured on a Zn-IMAC column, eluted with imidazole, further separated by SDS-PAGE (Fig. 5a) and identified by peptide mass fingerprinting (Table 1). Plasma was also applied to a column that had not been charged with any metal ions to check for any non-specific binding to the resin (Fig. 5c), and in this case no proteins were found to be retained on the column.
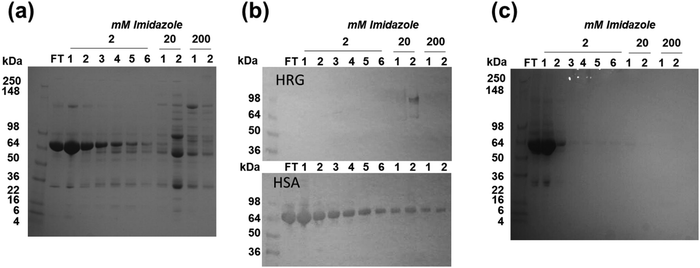 |
| Fig. 5 Fractions from IMAC chromatography on a Zn-charged column (a) analysed using western blotting and immunodetection (b) with antibodies against HSA and HRG. For comparison, fractions from a metal-free IMAC column are shown in (c). FT = flow-through. | |
Table 1
Homo sapiens plasma proteins binding to Zn-IMAC columns, identified by peptide mass fingerprinting using Mascot to search results against the NCBI database. Only searches with significant MOWSE (MOlecular Weight SEarch) scores were recorded
Protein |
Mass/kDa |
Peptides matched |
Sequence coverage (%) |
MOWSE score |
Haemoglobin subunit β |
16.1 |
9 |
76 |
90 |
Immunoglobulin light chain |
28.1 |
10 |
63 |
81 |
Haptoglobin |
38.7 |
14 |
35 |
113 |
Chain B IgG1 Fc fragment |
23.9 |
5 |
37 |
75 |
Immunoglobulin heavy chain |
42.4 |
12 |
30 |
72 |
Albumin |
70.6 |
16 |
31 |
119 |
Complement C3b |
71.2 |
26 |
47 |
240 |
Chain A transferrin |
77.3 |
34 |
48 |
228 |
Chain B complement C4 |
84.8 |
15 |
23 |
67 |
Plasminogen type II |
91.1 |
22 |
34 |
136 |
Complement C3 |
114.2 |
30 |
33 |
210 |
Ceruloplasmin |
116.2 |
14 |
16 |
103 |
α2-Macroglobulin (Zn) |
164.6 |
44 |
39 |
279 |
Inter-alpha inhibitor H1 |
99.8 |
13 |
21 |
81 |
Sensitivity is a clear limitation of a 1D SDS-PAGE approach, however comparison with the uncharged gel demonstrates that several proteins were selectively enriched in the fractions eluted with 20 and 200 mM imidazole. Molecular weights ranged between 16 and 165 kDa (Table 1). Identified proteins also included haemoglobin, likely due to partial lysis of erythrocytes during the plasma manufacturing process. The remaining entries mostly refer to known high-abundance plasma proteins.
A large quantity of the most abundant serum protein, human serum albumin (HSA) was observed in all fractions, evidenced by western blotting and immunodetection using HSA antibodies (Fig. 5b). Interestingly, comparison of the gels for Zn-loaded and Zn-free IMAC demonstrates that the occurrence of HSA across all fractions is not a mere consequence of its high abundance, since the albumin-related bands are very faint in either wash or elution fractions from the metal-free IMAC column (Fig. 5c). In contrast, albumin was present in both wash and elution fractions of the Zn-charged column (Fig. 5a). Nonetheless, most albumin was found in either flow-through or wash fractions, indicating a rather low affinity of HSA for a Zn-IMAC column. This finding can be understood when the locations and structures of the known metal binding sites in HSA are considered: site A (the major Zn2+-binding site) is not a surface site, but is partially buried between domains IA and IIA (Fig. 1). The interaction of this site with immobilised Zn2+ is therefore sterically unlikely. It might be envisioned that partial unfolding of albumin might lead to exposure of the two half-sites, but since these are only composed of either one (His67) or two (His247 and Asp249) residues, this would not generate a strong interaction with the column either. Another known binding site is the N-terminal ATCUN motif, which is the primary binding site for Cu2+ and Ni2+. Although Zn2+-binding has been demonstrated for short peptide mimics of this motif, the affinity of this site for Zn2+ at neutral pH is rather weak.37,103 Furthermore, calorimetric and crystallographic evidence of a secondary Zn2+ site of equine albumin, involving His9, Asp13 and Asp254 (fully conserved in HSA),37 might present a possible candidate for the secondary Zn2+ binding site (site B). This site is more exposed than site A, therefore its contribution to the IMAC affinity of albumin cannot be ruled out. However, its affinity for Zn2+ is almost 2 orders of magnitude weaker than site A.45 In conclusion, neither of the well-defined metal sites in HSA are expected to display strong interaction with a Zn-IMAC column, although Cu- and Ni-IMAC columns may display stronger HSA binding.67 The two most abundant Fe- and Cu-binding plasma proteins, respectively transferrin and ceruloplasmin, were also amongst the captured proteins. It is unknown whether their capture on the Zn-IMAC column involves their known metal-binding sites.
Amongst the strongly enriched proteins were immunoglobulin light and heavy chains (Table 1). Several components of the complement system were also detected, including complement C3 and C4. These proteins, as well as further complement components, are in fact already known to interact with Zn2+. In particular, Zn2+ inhibits factors I and H, and promotes protein–protein interactions between C3 and factor H.104,105 Many of these proteins are expected to elute in the SEC fractions with fatty-acid mediated increases in zinc. Another protein with higher molecular weight, α2-macroglobulin, is partially homologous to complement proteins.106 As previously explained, α2-macroglobulin harbours the largest proportion of non-exchangeable zinc in plasma,48 but the site(s) responsible for this fact are likely to differ from those responsible for the affinity to the IMAC column, as metal sites with reasonably fast exchange kinetics are prerequisite for binding to an IMAC column. Furthermore, isotope-exchange experiments using 65Zn indicated that α2-macroglobulin does not exchange, or bind extra Zn2+ under relevant conditions,107 rendering it an unlikely candidate in the re-distribution of Zn2+ from albumin.
Though histidine-rich glycoprotein (HRG) was not amongst the proteins identified by peptide mass fingerprinting of SDS-PAGE bands, western blotting and immunodetection revealed the presence of HRG in the second IMAC elution fraction (20 mM imidazole), confirming the capacity of this protein for binding (partially complexed) Zn2+ (Fig. 5b). This has been reported previously,108–110 and as mentioned, many HRG activities are accepted to be Zn2+-dependent.110,111 This is in agreement with our earlier detection of HRG in size exclusion chromatography (SEC) fractions with increased Zn2+ after metal re-distribution in the presence of FFAs.
Given the association between elevated plasma fatty acid levels and the incidence of thrombotic disease,112 a possible Zn2+ re-distribution from albumin to an important coagulation-regulating protein suggests that the described allosteric switch, linking plasma FFA levels and Zn2+ distribution, may have significant implications in the progression of various cardiovascular disorders. Physiologically-relevant long-chain saturated FFAs such as palmitate (C16) and stearate (C18) have even higher affinity for albumin,45,113 and so it is likely that such an effect would be at least as apparent, if not more pronounced, in vivo. Furthermore, HRG has a comparatively high abundance (1–2 μM), relative to many other plasma proteins. Considering the affinities of HSA (K1(ITC) = 1.35 × 105 M and K2(ITC) = 3 × 103 M for site B) and HRG (KITC 8.06 × 104 M) for Zn2+,45 we suggest that HRG might be a likely candidate acceptor protein during serum zinc re-distribution, with abundant proteins of the complement system (C3, C3b, C4, factor H1) also being likely candidates. It is well-known that zinc dynamics play roles in coagulation and immune response, and therefore there must be conditions under which associated proteins become populated with Zn2+. Since albumin (the major Zn2+ binding plasma protein) is always present, it too must be involved in such dynamic networks. Furthermore, by virtue of the allosteric switch, changes in FFA levels are hence predicted to impact on both blood coagulation and the immune system, as a result of Zn2+ speciation changes in blood plasma.
Conclusions
SEC combined offline with ICP-MS for the analysis of extracellular fluids has provided insight into changes in Zn2+ speciation, induced by the presence of myristate, a long-chain (C14:0) non-esterified fatty acid. This is a consequence of a now well-established allosteric mechanism on serum albumin, the key Zn2+ carrier protein in the bloodstream. The operation of this molecular mechanism and its immediate consequences were for the first time observed in complex biological systems by chromatographic techniques, with complementary evidence provided by the use of a Zn2+-sensing fluorescent reagent, FluoZin-3. In pure albumin systems (i.e. in the absence of any other Zn2+ acceptors), any Zn2+ displaced from albumin was scavenged by the SEC resin. In foetal calf serum and human plasma, Zn2+ liberated from albumin was overwhelmingly re-distributed to other plasma proteins that were largely of higher molecular weight than albumin. One highly likely candidate Zn2+ acceptor protein is HRG, a multifunctional protein whose activities are modulated by zinc binding. Further potential Zn2+ acceptor plasma proteins were identified by capture on an IMAC column, followed by SDS-PAGE and peptide mass fingerprinting, and include several proteins of the complement system.
From this, we infer that the decreased Zn2+ binding capacity of albumin in the presence of FFAs may have significant implications, for both normal and disease-altered physiology. Whilst numerous pathological diseases are associated with elevated levels of free fatty acids, not limited to cardiovascular diseases (including thrombosis),114–116 diabetes,117–119 and neurodegenerative diseases,120–122 FFA levels also fluctuate during periods of intense exercise, fasting and after the consumption of food.123–125 A deeper understanding of the downstream consequences of FFA-mediated Zn2+ re-distribution is now required to comprehend the wider impact this may have on organismal physiology.
Conflicts of interest
There are no conflicts to declare.
Acknowledgements
This work was supported by the Leverhulme Trust (grant ref. RPG-2017-214) and BBSRC (grant ref. BB/J006467/1). Some equipment used in this research was obtained through Birmingham Science City with support from Advantage West Midlands and the European Regional Development Fund. We thank Dr Lijiang Song for excellent assistance in mass spectrometry, and Phil Aston and Deogratias Ikemere for their contributions to method development.
References
- I. Cakmak, Enrichment of fertilizers with zinc: an excellent investment for humanity and crop production in India, J. Trace Elem. Med. Biol., 2009, 23, 281–289 CrossRef CAS PubMed.
- A. S. Prasad, Discovery of human zinc deficiency: its impact on human health and disease, Adv. Nutr., 2013, 4, 176–190 CrossRef CAS PubMed.
- J. C. King, Zinc: an essential but elusive nutrient, Am. J. Clin. Nutr., 2011, 94, 679–684 CrossRef PubMed.
- L. M. Plum, L. Rink and H. Haase, The essential toxin: impact of zinc on human health, Int. J. Environ. Res. Public Health, 2010, 7, 1342–1365 CrossRef CAS PubMed.
- A. Takeda, M. Nakamura, H. Fujii and H. Tamano, Synaptic Zn2+ homeostasis and its significance, Metallomics, 2013, 5, 417–423 RSC.
- M. Soinio, J. Marniemi, M. Laakso, K. Pyörälä, S. Lehto and T. Rönnemaa, Serum zinc level and coronary heart disease events in patients with type 2 diabetes, Diabetes Care, 2007, 30, 523–528 CrossRef CAS PubMed.
- J. D. Molkentin, The zinc finger-containing transcription factors GATA-4, -5, and -6: ubiquitously expressed regulators of tissue-specific gene expression, J. Biol. Chem., 2000, 275, 38949–38952 CrossRef CAS PubMed.
- K. A. McCall, C.-C. Huang and C. A. Fierke, Function and mechanism of zinc metalloenzymes, J. Nutr., 2000, 130, 1437–1446 CrossRef PubMed.
- T. Hirano, M. Murakami, T. Fukada, K. Nishida, S. Yamasaki and T. Suzuki, Roles of zinc and zinc signaling in immunity: zinc as an intracellular signaling molecule, Adv. Immunol., 2008, 97, 149–176 CAS.
- R. A. Bozym, F. Chimienti, L. J. Giblin, G. W. Gross, I. Korichneva, Y. Li, S. Libert, W. Maret, M. Parviz, C. J. Frederickson and R. B. Thompson, Free zinc ions outside a narrow concentration range are toxic to a variety of cells in vitro, Exp. Biol. Med., 2010, 235, 741–750 CrossRef CAS PubMed.
- P. T. S. Wong and Y. K. Chau, Zinc toxicity to freshwater algae, Environ. Toxicol. Water Qual., 1990, 5, 167–177 CrossRef CAS.
- A. Magdaleno, C. G. Vélez, M. T. Wenzel and G. Tell, Effects of cadmium, copper and zinc on growth of four isolated algae in a highly polluted Argentina river, Bull. Environ. Contam. Toxicol., 2014, 92, 202–207 CrossRef CAS PubMed.
- H. Haase, S. Hebel, G. Engelhardt and L. Rink, The biochemical effects of extracellular Zn2+ and other metal ions are severely affected by their speciation in cell culture media, Metallomics, 2015, 7, 102–111 RSC.
- J. P. C. Coverdale, S. Khazaipoul, S. Arya, A. J. Stewart and C. A. Blindauer, Crosstalk between zinc and free fatty acids in plasma, Biochim. Biophys. Acta, Mol. Cell Biol. Lipids, 2019, 1864, 532–542 CrossRef CAS PubMed.
- N. F. Krebs, Overview of zinc absorption and excretion in the human gastrointestinal tract, J. Nutr., 2000, 130, 1374–1377 CrossRef PubMed.
- W. Hussain, A. Mumtaz, F. Yasmeen, S. Q. Khan and T. Butt, Reference range of zinc in adult population (20–29 years) of Lahore, Pakistan, Pak. J. Med. Sci., 2014, 30, 545–548 Search PubMed.
- A. Ghasemi, S. Zahediasl, F. Hosseini-Esfahani and F. Azizi, Reference values for serum zinc concentration and prevalence of zinc deficiency in adult Iranian subjects, Biol. Trace Elem. Res., 2012, 149, 307–314 CrossRef CAS PubMed.
- J. Jiao, H. Guo, Y. He, J. Wang, J. Yuan and W. Hu, Meta-analysis of the association between serum iron levels and Parkinson's disease: evidence from 11 publications, Brain Res., 2016, 1646, 490–493 CrossRef CAS PubMed.
- I. H. Garba, G. A. Ubom and N. B. Ejiogu, Serum copper concentration in adults with acute, uncomplicated Falciparum malaria infection, Biol. Trace Elem. Res., 2006, 113, 125–130 CrossRef CAS PubMed.
- Z. Nahar, M.
A. K. Azad, M. A. Rahman, M. A. Rahman, W. Bari, S. N. Islam, M. S. Islam and A. J. B. T. E. R. Hasnat, Comparative analysis of serum manganese, zinc, calcium, copper and magnesium level in panic disorder patients, Biol. Trace Elem. Res., 2010, 133, 284–290 CrossRef CAS PubMed.
- P. Kajic, I. Milosev, B. Pihlar and V. Pisot, Determination of trace cobalt concentrations in human serum by adsorptive stripping voltammetry, J. Trace Elem. Med. Biol., 2003, 17, 153–158 CrossRef CAS.
- W. R. Keyes and J. R. Turnlund, Determination of molybdenum and enriched Mo stable isotope concentrations in human blood plasma by isotope dilution ICP-MS, J. Anal. At. Spectrom., 2002, 17, 1153–1156 RSC.
- F. T. Wieringa, M. A. Dijkhuizen, M. Fiorentino, A. Laillou and J. Berger, Determination of zinc status in humans: which indicator should we use?, Nutrients, 2015, 7, 3252–3263 CrossRef CAS PubMed.
- R. J. Cousins, Toward a molecular understanding of zinc metabolism, Clin. Physiol. Biochem., 1986, 4, 20–30 CAS.
- J. Jansen, W. Karges and L. Rink, Zinc and diabetes – clinical links and molecular mechanisms, J. Nutr. Biochem., 2009, 20, 399–417 CrossRef CAS PubMed.
- R. Milanino, M. Marrella, R. Gasperini, M. Pasqualicchio and G. Velo, Copper and zinc body levels in inflammation: an overview of the data obtained from animal and human studies, Agents Actions, 1993, 39, 195–209 CrossRef CAS PubMed.
- D. C. McMillan, D. Maguire and D. Talwar, Relationship between nutritional status and the systemic inflammatory response: micronutrients, Proc. Nutr. Soc., 2019, 78, 56–67 CrossRef CAS PubMed.
- C. Devirgiliis, P. Zalewski, G. Perozzi and C. Murgia, Zinc fluxes and zinc transporter genes in chronic diseases, Mutat. Res., 2007, 622, 84–93 CrossRef CAS PubMed.
- E. R. Verni, F. Moyano, L. D. Martinez, A. V. Lapierre and R. A. Gil, Handling spectral interferences and matrix effects in DRC-ICP-MS to assess the elemental profile in human serum samples after dissolution with formic acid, J. Anal. At. Spectrom., 2013, 28, 1655–1659 RSC.
- S. R. Himmelhoch, H. A. Sober, B. L. Vallee, E. A. Peterson and K. Fuwa, Spectrographic and chromatographic resolution of metalloproteins in human serum, Biochemistry, 1966, 5, 2523–2530 CrossRef CAS PubMed.
- J. W. Foote and H. T. Delves, Distribution of zinc amongst human serum proteins determined by affinity chromatography and atomic-absorption spectrophotometry, Analyst, 1983, 108, 492–504 RSC.
- D. C. Chilvers, J. B. Dawson, M.-H. Bahreyni-Toosi and A. Hodgkinson, Identification and determination of copper-and zinc-protein complexes in blood plasma after chromatographic separation on DEAE-Sepharose CL-6B, Analyst, 1984, 109, 871–876 RSC.
- L. Ebdon, S. Hill and P. Jones, Application of directly coupled flame atomic absorption spectrometry-fast protein liquid chromatography to the determination of protein-bound metals, Analyst, 1987, 112, 437–440 RSC.
- E. L. Giroux, M. Durieux and P. J. Schechter, A study of zinc distribution in human serum, Bioinorg. Chem., 1976, 5, 211–218 CrossRef CAS PubMed.
- J. T. Wu, S. M. Monir-Vaghefi and F. Clayton, Human alpha-fetoprotein and albumin: differences in zinc binding, Clin. Physiol. Biochem., 1987, 5, 85–94 CAS.
- J. Lu, A. J. Stewart, P. J. Sadler, T. J. T. Pinheiro and C. A. Blindauer, Albumin as a zinc carrier: properties of its high-affinity zinc-binding site, Biochem. Soc. Trans., 2008, 36, 1317–1321 CrossRef CAS PubMed.
- K. B. Handing, I. G. Shabalin, O. Kassaar, S. Khazaipoul, C. A. Blindauer, A. J. Stewart, M. Chruszcz and W. Minor, Circulatory zinc transport is controlled by distinct interdomain sites on mammalian albumins, Chem. Sci., 2016, 7, 6635–6648 RSC.
- A. I. Ivanov, J. Christodoulou, J. A. Parkinson, K. J. Barnham, A. Tucker, J. Woodrow and P. J. Sadler, Cisplatin binding sites on human albumin, J. Biol. Chem., 1998, 273, 14721–14730 CrossRef CAS PubMed.
- L. Galantini, C. Leggio, P. V. Konarev and N. V. Pavel, Human serum albumin binding ibuprofen: a 3D description of the unfolding pathway in urea, Biophys. Chem., 2010, 147, 111–122 CrossRef CAS PubMed.
- D. Z. Zvetanka, Studies on drug – human serum albumin binding: the current state of the matter, Curr. Pharm. Des., 2015, 21, 1817–1830 CrossRef PubMed.
- J. Lu, A. J. Stewart, D. Sleep, P. J. Sadler, T. J. T. Pinheiro and C. A. Blindauer, A molecular mechanism for modulating plasma Zn speciation by fatty acids, J. Am. Chem. Soc., 2012, 134, 1454–1457 CrossRef CAS PubMed.
- A. A. Bhattacharya, T. Grüne and S. Curry, Crystallographic analysis reveals common modes of binding of medium and long-chain fatty acids to human serum albumin, J. Mol. Biol., 2000, 303, 721–732 CrossRef CAS PubMed.
- S. Curry, Lessons from the crystallographic analysis of small molecule binding to human serum albumin, Drug Metab. Pharmacokinet., 2009, 24, 342–357 CrossRef CAS PubMed.
- C. A. Blindauer, I. Harvey, K. E. Bunyan, A. J. Stewart, D. Sleep, D. J. Harrison, S. Berezenko and P. J. Sadler, Structure, properties, and engineering of the major zinc binding site on human albumin, J. Biol. Chem., 2009, 284, 23116–23124 CrossRef CAS PubMed.
- O. Kassaar, U. Schwarz-Linek, C. A. Blindauer and A. J. Stewart, Plasma free fatty acid levels influence Zn2+ dependent histidine-rich glycoprotein–heparin interactions via an allosteric switch on serum albumin, J. Thromb. Haemostasis, 2015, 13, 101–110 CrossRef CAS PubMed.
- C. A. Blindauer, S. Khazaipoul, R. Yu and A. J. Stewart, Fatty acid-mediated inhibition of metal binding to the multi-metal site on serum albumin: implications for cardiovascular disease, Curr. Top. Med. Chem., 2016, 16, 3021–3032 CrossRef CAS PubMed.
- J. R. Simard, P. A. Zunszain, J. A. Hamilton and S. Curry, Location of high and low affinity fatty acid binding sites on human serum albumin revealed by NMR drug-competition analysis, J. Mol. Biol., 2006, 361, 336–351 CrossRef CAS PubMed.
- J. P. Barnett, C. A. Blindauer, O. Kassaar, S. Khazaipoul, E. M. Martin, P. J. Sadler and A. J. Stewart, Allosteric modulation of zinc speciation by fatty acids, Biochim. Biophys. Acta, Gen. Subj., 2013, 1830, 5456–5464 CrossRef CAS PubMed.
- S. Curry, H. Mandelkow, P. Brick and N. Franks, Crystal structure of human serum albumin complexed with fatty acid reveals an asymmetric distribution of binding sites, Nat. Struct. Biol., 1998, 5, 827 CrossRef CAS PubMed.
- R. J. Cousins, J. P. Liuzzi and L. A. Lichten, Mammalian zinc transport, trafficking, and signals, J. Biol. Chem., 2006, 281, 24085–24089 CrossRef CAS PubMed.
- T. Fukada and T. Kambe, Molecular and genetic features of zinc transporters in physiology and pathogenesis, Metallomics, 2011, 3, 662–674 RSC.
- M. Foster and S. Samman, Zinc and regulation of inflammatory cytokines: implications for cardiometabolic disease, Nutrients, 2012, 4, 676–694 CrossRef CAS PubMed.
- H. J. Blewett and C. G. Taylor, Dietary zinc deficiency in rodents: effects on T-cell development, maturation and phenotypes, Nutrients, 2012, 4, 449–466 CrossRef CAS PubMed.
- M. Maares and H. Haase, Zinc and immunity: an essential interrelation, Arch. Biochem. Biophys., 2016, 611, 58–65 CrossRef CAS PubMed.
- J. W. Foote and H. T. Delves, Determination of non-protein-bound zinc in human serum using ultrafiltration and atomic absorption spectrometry with electrothermal atomisation, Analyst, 1988, 109, 709–711 RSC.
- H. Faure, A. Favier, M. Tripier and J. Arnaud, Determination of the major zinc fractions in human serum by ultrafiltration, Biol. Trace Elem. Res., 1990, 24, 25–37 CrossRef CAS PubMed.
- T.-H. Lin and S.-Y. Cheng, Determination of zinc fractions in human blood and seminal plasma by ultrafiltration and atomic absorption spectrophotometry, Biol. Trace Elem. Res., 1996, 51, 267–276 CrossRef CAS PubMed.
- E. Kelly, J. Mathew, J. E. Kohler, A. L. Blass and D. I. Soybel, Redistribution of labile plasma zinc during mild surgical stress in the rat, Transl. Res., 2011, 157, 139–149 CrossRef CAS PubMed.
- J. W. Foote and H. T. Delves, Albumin bound and alpha 2-macroglobulin bound zinc concentrations in the sera of healthy adults, J. Clin. Pathol., 1984, 37, 1050–1054 CrossRef CAS PubMed.
- A. B. Nowakowski, W. J. Wobig and D. H. Petering, Native SDS-PAGE: high resolution electrophoretic separation of proteins with retention of native properties including bound metal ions, Metallomics, 2014, 6, 1068–1078 RSC.
- N. Jakubowski, R. Lobinski and L. Moens, Metallobiomolecules. The basis of life, the challenge of atomic spectroscopy, J. Anal. At. Spectrom., 2004, 19, 1–4 RSC.
- J. Szpunar, Bio-inorganic speciation analysis by hyphenated techniques, Analyst, 2000, 125, 963–988 RSC.
- J. Szpunar, Advances in analytical methodology for bioinorganic speciation analysis: metallomics, metalloproteomics and heteroatom-tagged proteomics and metabolomics, Analyst, 2005, 130, 442–465 RSC.
- A. Sussulini and J. S. Becker, Combination of PAGE and LA-ICP-MS as an analytical workflow in metallomics: state of the art, new quantification strategies, advantages and limitations, Metallomics, 2011, 3, 1271–1279 RSC.
- M. Montes-Bayón and J. Bettmer, The use of stable isotopic tracers in metallomics studies, Adv. Exp. Med. Biol., 2018, 1055, 111–137 CrossRef PubMed.
- A. Sussulini, J. S. Becker and J. S. Becker, Laser ablation ICP-MS: Application in biomedical research, Mass Spectrom. Rev., 2017, 36, 47–57 CrossRef CAS PubMed.
- F. Wang, C. Chmil, F. Pierce, K. Ganapathy, B. B. Gump, J. A. MacKenzie, Y. Mechref and K. Bendinskas, Immobilized metal affinity chromatography and human serum proteomics, J. Chromatogr. B: Anal. Technol. Biomed. Life Sci., 2013, 934, 26–33 CrossRef CAS PubMed.
- A. Sussulini, C. E. M. Banzato and M. A. Z. Arruda, Exploratory analysis of the serum ionomic profile for bipolar disorder and lithium treatment, Int. J. Mass Spectrom., 2011, 307, 182–184 CrossRef CAS.
- K. L. Pei and J. Gailer, Probing the interaction of arsenobetaine with blood plasma constituents in vitro: an SEC-ICP-AES study, Metallomics, 2009, 1, 403–408 RSC.
- M. Sooriyaarachchi, A. Narendran and J. Gailer, Comparative hydrolysis and plasma protein binding of cis-platin and carboplatin in human plasma in vitro, Metallomics, 2011, 3, 49–55 RSC.
- S. A. Manley and J. Gailer, Analysis of the plasma metalloproteome by SEC-ICP-AES: bridging proteomics and metabolomics, Expert Rev. Proteomics, 2009, 6, 251–265 CrossRef CAS PubMed.
- M. H. M. Klose, A. Schoberl, P. Heffeter, W. Berger, C. G. Hartinger, G. Koellensperger, S. M. Meier-Menches and B. K. Keppler, Serum-binding properties of isosteric ruthenium and osmium anticancer agents elucidated by SEC-ICP-MS, Monatsh. Chem., 2018, 149, 1719–1726 CrossRef CAS PubMed.
- M. C. Linder, Ceruloplasmin and other copper binding components of blood plasma and their functions: an update, Metallomics, 2016, 8, 887–905 RSC.
- M. Sooriyaarachchi and J. Gailer, Removal of Fe3+ and Zn2+ from plasma metalloproteins by iron chelating therapeutics depicted with SEC-ICP-AES, Dalton Trans., 2010, 39, 7466–7473 RSC.
- M. A. Garcia-Sevillano, T. Garcia-Barrera, F. Navarro, J. Gailer and J. L. Gomez-Ariza, Use of elemental and molecular-mass spectrometry to assess the toxicological effects of inorganic mercury in the mouse Mus musculus, Anal. Bioanal. Chem., 2014, 406, 5853–5865 CrossRef CAS PubMed.
- J. Folch, M. Lees and G. H. Sloane Stanley, A simple method for the isolation and purification of total lipides from animal tissues, J. Biol. Chem., 1957, 226, 497–509 CAS.
- U. K. Laemmli, Cleavage of structural proteins during the assembly of the head of bacteriophage T4, Nature, 1970, 227, 680–685 CrossRef CAS PubMed.
- A. J. Stewart, C. A. Blindauer, S. Berezenko, D. Sleep and P. J. Sadler, Interdomain zinc site on human albumin, Proc. Natl. Acad. Sci. U. S. A., 2003, 100, 3701–3706 CrossRef CAS PubMed.
- L. J. Dangott and L. W. Cunningham, Residual alpha 2-macroglobulin in fetal calf serum and properties of its complex with thrombin, Biochem. Biophys. Res. Commun., 1982, 107, 1243–1251 CrossRef CAS PubMed.
- I. Petitpas, T. Grune, A. A. Bhattacharya and S. Curry, Crystal structures of human serum albumin complexed with monounsaturated and polyunsaturated fatty acids, J. Mol. Biol., 2001, 314, 955–960 CrossRef CAS PubMed.
- I. Marszalek, A. Krezel, W. Goch, I. Zhukov, I. Paczkowska and W. Bal, Revised stability constant, spectroscopic properties and binding mode of Zn(II) to FluoZin-3, the most common zinc probe in life sciences, J. Inorg. Biochem., 2016, 161, 107–114 CrossRef CAS PubMed.
- M. J. Devinney, I. J. Reynolds and K. E. Dineley, Simultaneous detection of intracellular free calcium and zinc using fura-2FF and FluoZin-3, Cell Calcium, 2005, 37, 225–232 CrossRef CAS PubMed.
- J. Zhao, B. A. Bertoglio, K. R. Gee and A. R. Kay, The zinc indicator FluoZin-3 is not perturbed significantly by physiological levels of calcium or magnesium, Cell Calcium, 2008, 44, 422–426 CrossRef CAS PubMed.
- D. W. Domaille, E. L. Que and C. J. Chang, Synthetic fluorescent sensors for studying the cell biology of metals, Nat. Chem. Biol., 2008, 4, 168–175 CrossRef CAS PubMed.
- W. Maret, Analyzing free zinc(II) ion concentrations in cell biology with fluorescent chelating molecules, Metallomics, 2015, 7, 202–211 RSC.
- A. B. Nowakowski, J. W. Meeusen, H. Menden, H. Tomasiewicz and D. H. Petering, Chemical–biological properties of zinc sensors TSQ and zinquin: formation of sensor-Zn-protein adducts versus Zn(sensor)2 complexes, Inorg. Chem., 2015, 54, 11637–11647 CrossRef CAS PubMed.
- A. B. Nowakowski and D. H. Petering, Reactions of the fluorescent sensor, zinquin, with the zinc-proteome: adduct formation and ligand substitution, Inorg. Chem., 2011, 50, 10124–10133 CrossRef CAS PubMed.
- Y. Zhang and H. Görner, Photoprocesses of xanthene dyes bound to lysozyme or serum albumin, Photochem. Photobiol., 2009, 85, 677–685 CrossRef CAS PubMed.
- S. Fatima, T. Anwar, N. Ahmad, A. Islam and P. Sen, Non-enzymatic glycation enhances human serum albumin binding capacity to sodium fluorescein at room temperature: a spectroscopic analysis, Clin. Chim. Acta, 2017, 469, 180–186 CrossRef CAS PubMed.
- N. Barbero, E. Barni, C. Barolo, P. Quagliotto, G. Viscardi, L. Napione, S. Pavan and F. Bussolino, A study of the interaction between fluorescein sodium salt and bovine serum albumin by steady-state fluorescence, Dyes Pigm., 2009, 80, 307–313 CrossRef CAS.
- A. Staszewska, E. Kurowska and W. Bal, Ternary complex formation and competition quench fluorescence of ZnAF family zinc sensors, Metallomics, 2013, 5, 1483–1490 RSC.
- I. Marszalek, W. Goch and W. Bal, Ternary Zn(II) complexes of FluoZin-3 and the low molecular weight component of the exchangeable cellular zinc pool, Inorg. Chem., 2018, 57, 9826–9838 CrossRef CAS PubMed.
- J. Masuoka and P. Saltman, Zinc(II) and copper(II) binding to serum albumin. A comparative study of dog, bovine, and human albumin, J. Biol. Chem., 1994, 269, 25557–25561 CAS.
- E. Ohyoshi, Y. Hamada, K. Nakata and S. Kohata, The interaction between human and bovine serum albumin and zinc studied by a competitive spectrophotometry, J. Inorg. Biochem., 1999, 75, 213–218 CrossRef CAS PubMed.
- D. C. Bode, H. F. Stanyon, T. Hirani, M. D. Baker, J. Nield and J. H. Viles, Serum albumin's protective inhibition of amyloid-β fiber formation is suppressed by cholesterol, fatty acids and warfarin, J. Mol. Biol., 2018, 430, 919–934 CrossRef CAS PubMed.
- D. De Mel and C. Suphioglu, Fishy business: effect of omega-3 fatty acids on zinc transporters and free zinc availability in human neuronal cells, Nutrients, 2014, 6, 3245–3258 CrossRef PubMed.
-
J. Schaller, S. Gerber, U. Kämpfer, S. Lejon and C. Trachsel, Human blood plasma proteins: structure and function, John Wiley & Sons, 2018 Search PubMed.
- A. L. Jones, M. D. Hulett and C. R. Parish, Histidine-rich glycoprotein: a novel adaptor protein in plasma that modulates the immune, vascular and coagulation systems, Immunol. Cell Biol., 2005, 83, 106–118 CrossRef CAS PubMed.
- D.-B. Borza and W. T. Morgan, Histidine-proline-rich glycoprotein as a plasma pH sensor: modulation of its interaction with glycosaminoglycans by pH and metals, J. Biol. Chem., 1998, 273, 5493–5499 CrossRef CAS PubMed.
- A. J. Stewart, C. A. Blindauer and P. J. Sadler, Plasma fatty acid levels may regulate the Zn2+-dependent activities of histidine-rich glycoprotein, Biochimie, 2009, 91, 1518–1522 CrossRef CAS PubMed.
- J. C. Hoak, E. D. Warner and W. E. Connor, Platelets, fatty acids and thrombosis, Circ. Res., 1967, 20, 11–17 CrossRef CAS PubMed.
- A. I. S. Sobczak, S. J. Pitt and A. J. Stewart, Influence of zinc on glycosaminoglycan neutralisation during coagulation, Metallomics, 2018, 10, 1180–1190 RSC.
- H. Lakusta and B. Sarkar, Equilibrium studies of zinc(II) and cobalt(II) binding to tripeptide analogues of the amino terminus of human serum albumin, J. Inorg. Biochem., 1979, 11, 303–315 CrossRef CAS.
- R. Nan, S. Tetchner, E. Rodriguez, P.-J. Pao, J. Gor, I. Lengyel and S. J. Perkins, Zinc-induced self-association of complement C3b and factor H: implications for inflammation and age-related macular degeneration, J. Biol. Chem., 2013, 288, 19197–19210 CrossRef CAS PubMed.
- D. Smailhodzic, F. van Asten, A. Blom, F. C. Mohlin, A. den Hollander, J. P. H. van de Ven, R. Huet, J. M. M. Groenewoud, Y. Tian, T. T. J. M. Berendschot, Y. Lechanteur, S. Fauser, C. de Bruijn, M. Daha, G. Wilt, C. B. Hoyng and B. Jeroen Klevering, Zinc supplementation inhibits complement activation in age-related macular degeneration, PLoS One, 2014, 9, 112682 CrossRef PubMed.
- N. Doan and P. G. W. Gettins, Human α-macroglobulin is composed of multiple domains, as predicted by homology with complement component C3, Biochem. J., 2007, 407, 23–30 CrossRef CAS.
- A. F. Parisi and B. L. Vallee, Isolation of a zinc α2-macroglobulin from human serum, Biochemistry, 1970, 9, 2421–2426 CrossRef CAS PubMed.
- T.-T. Yip and T. W. Hutchens, Metal ion affinity adsorption of a Zn(II)-transport protein present in maternal plasma during lactation: Structural characterization and identification as histidine-rich glycoprotein, Protein Expression Purif., 1991, 2, 355–362 CrossRef CAS.
- S. L. Guthans and W. T. Morgan, The interaction of zinc, nickel and cadmium with serum albumin and histidine-rich glycoprotein assessed by equilibrium dialysis and immunoadsorbent chromatography, Arch. Biochem. Biophys., 1982, 218, 320–328 CrossRef CAS.
- C. B. Peterson, W. T. Morgan and M. N. Blackburn, Histidine-rich glycoprotein modulation of the anticoagulant activity of heparin. Evidence for a mechanism involving competition with both antithrombin and thrombin for heparin binding, J. Biol. Chem., 1987, 262, 7567–7574 CAS.
- M. Failla, M. van de Veerdonk, W. T. Morgan and J. C. Smith, Characterization of zinc-binding proteins of plasma in familial hyperzincemia, J. Lab. Clin. Med., 1983, 100, 943–952 Search PubMed.
- J. C. Hoak, J. C. Poole and D. S. Robinson, Thrombosis associated with mobilization of fatty acids, Am. J. Pathol., 1963, 43, 987–998 CAS.
- A. A. Spector, Fatty acid binding to plasma albumin, J. Lipid Res., 1975, 16, 165–179 CAS.
- S. Pilz and W. Marz, Free fatty acids as a cardiovascular risk factor, Clin. Chem. Lab. Med., 2008, 46, 429–434 CAS.
- L. Djoussé, D. Benkeser, A. Arnold, J. R. Kizer, S. J. Zieman, R. N. Lemaitre, R. P. Tracy, J. S. Gottdiener, D. Mozaffarian, D. S. Siscovick, K. J. Mukamal and J. H. Ix, Plasma free fatty acids and risk of heart failure: the cardiovascular health study, Circ.: Heart Failure, 2013, 6, 964–969 Search PubMed.
- M. Mathew, E. Tay and K. Cusi, Elevated plasma free fatty acids increase cardiovascular risk by inducing plasma biomarkers of endothelial activation, myeloperoxidase and PAI-1 in healthy subjects, Cardiovasc. Diabetol., 2010, 9, 9–18 CrossRef.
- F. Karpe, J. R. Dickmann and K. N. Frayn, Fatty acids, obesity, and insulin resistance: time for a re-evaluation, Diabetes, 2011, 60, 2441–2449 CrossRef CAS.
- G. Boden, Free fatty acids, insulin resistance, and type 2 diabetes mellitus, Proc. Assoc. Am. Physicians, 1999, 111, 241–248 CrossRef CAS.
- G. Boden and G. I. Shulman, Free fatty acids in obesity and type 2 diabetes: defining their role in the development of insulin resistance and β-cell dysfunction, Eur. J. Clin. Invest., 2002, 32, 14–23 CrossRef CAS.
- Y. Cui, X. Chen, L. Liu, W. Xie, Y. Wu, Q. Wu and D. Wang, Gas chromatography-mass spectrometry analysis of the free fatty acids in serum obtained from patients with Alzheimer's disease, Biomed. Mater. Eng., 2015, 26, 2165–2177 Search PubMed.
- S. G. Snowden, A. A. Ebshiana, A. Hye, Y. An, O. Pletnikova, R. O’Brien, J. Troncoso, C. Legido-Quigley and M. Thambisetty, Association between fatty acid metabolism in the brain and Alzheimer disease neuropathology and cognitive performance: a nontargeted metabolomic study, PLoS Med., 2017, 14, 1002266 CrossRef PubMed.
- K. Jensen Majken, P. Buzkova, L. Fitzpatrick Annette, W. T. Longstreth, H. Kuller Lewis, L. Lopez Oscar, S. Siscovick David, R. Kizer Jorge, J. H. Ix, L. Djoussé and J. Mukamal Kenneth, Plasma free fatty acids and risk of dementia: the cardiovascular health study, Circ.: Heart Failure, 2018, 137, 24 CrossRef.
- M. D. Jensen, K. Ekberg and B. R. Landau, Lipid metabolism during fasting, Am. J. Physiol.: Endocrinol. Metab., 2001, 281, 789–793 CrossRef.
- R. Bahr, A. T. Hostmark, E. A. Newsholme, O. Gronnerod and O. M. Sejersted, Effect of exercise on recovery changes in plasma levels of FFA, glycerol, glucose and catecholamines, Acta Physiol. Scand., 1991, 143, 105–115 CrossRef CAS.
- K. Rodahl, H. I. Miller and B. Issekutz, Plasma free fatty acids in exercise, J. Appl. Physiol., 1964, 19, 489–492 CrossRef CAS.
Footnotes |
† Electronic supplementary information (ESI) available. See DOI: 10.1039/c9mt00177h |
‡ These authors contributed equally to this work. |
|
This journal is © The Royal Society of Chemistry 2019 |