DOI:
10.1039/C9FO01046G
(Paper)
Food Funct., 2019,
10, 4505-4521
Gut microbiota might be a crucial factor in deciphering the metabolic benefits of perinatal genistein consumption in dams and adult female offspring†
Received
17th May 2019
, Accepted 12th July 2019
First published on 26th July 2019
Abstract
Adverse early-life exposures program an increased risk of chronic metabolic diseases in adulthood. However, the effects of genistein consumption in early life on metabolic health are unclear. Our objective was to investigate whether perinatal genistein intake could mitigate the deleterious effects of a high-fat diet (HF) on metabolism in dams and female offspring and to explore the role of the gut microbiota in mediating the transgenerational effects. C57BL/6 female mice were fed a HF, HF with genistein (0.6 g kg−1 diet) or normal control diet for 3 weeks before mating and throughout pregnancy and lactation. The offspring had free access to normal diet from weaning to 24 weeks of age. A glucose tolerance test was performed and the levels of serum insulin and lipid were measured. The cecal contents were collected for 16s rDNA sequencing. The results showed that perinatal genistein intake could not only significantly reduce blood glucose levels, insulin and free fatty acids (FFA) in dams, but also improve glucose tolerance, insulin sensitivity and serum lipid profiles in adult female offspring. Significant enrichment of short-chain fatty acid (mainly butyrate)-producing bacteria might play crucial roles in deciphering the metabolic benefits of perinatal genistein intake in dams. The obvious decrease in harmful microorganisms and increase in Erysipelotrichaceae_incertae_sedis were associated with the protective effects of maternal genistein intake on female offspring. In addition, Bifidobacterium might be an important factor for deciphering the metabolic improvement in both dams and female offspring by dietary genistein. Overall, perinatal genistein intake attenuated the harmful effects of HF on metabolism in both dams and female offspring, and the protective effects were associated with the alterations in the gut microbiota, which provides new evidence and targets for mitigating the poor effects of adverse early-life exposures on metabolic health in later life.
1. Introduction
The global prevalence of metabolic disturbances, including glucose intolerance, insulin resistance and obesity, has brought unprecedented challenges. It is traditionally accepted that chronic metabolic diseases arise at the interface of genetics and environmental factors, including physical inactivity, smoking and high-calorie diet. In recent years, a large number of observational studies and experimental animal models have consistently highlighted the importance of the early-life environment in determining disease trajectories in later life.1,2 More specifically, adverse intrauterine or postnatal exposures, including maternal obesity and gestational diabetes mellitus (GDM), programmed elevated risks of developing chronic metabolic diseases in adult life, such as obesity and glucose intolerance.3 Thus, the hypothesis of developmental origins of health and disease (DOHaD) has been put forward, which proposes that environmental exposures during critical windows of development program permanent changes in later life. Although epigenetics, microbiome and metabolome were all considered the potential mechanisms, the specific mechanism of the DOHaD still remains unclear.4 Substantial amounts of research and our previous studies both showed that maternal high-fat diet during pregnancy and lactation not only resulted in metabolic disorders per se, but significantly increased susceptibility to glucose intolerance, insulin resistance and lipid disorders in adult offspring.5–7 Therefore, the perinatal period might be a critical window for preventing the transgenerational transmission of metabolic diseases. Effective measures should be taken to reset the trajectories of chronic metabolic disease.
Over the last few decades, increasing research has provided evidence to support the beneficial role of bioactive dietary compounds in the prevention and treatment of metabolic disorders.8 Genistein, as one of the major components in soy isoflavone, has a similar structure to 17β-estradiol and is also known as a phytoestrogen. The protective effects of genistein on cancer,9 bone metabolic disease10 and cardiovascular disease11 have been extensively researched. The safety of genistein consumption has been confirmed in both animals and humans.12 In terms of metabolic diseases, several large epidemiological studies preliminarily showed that the dietary intake of soy isoflavone was negatively correlated with the risk of type 2 diabetes mellitus (T2DM), especially in obese population.13,14 Subsequently, emerging clinical trials and animal experiments verified the benefits of genistein on glucose intolerance, insulin sensitivity and lipid profile disorders.15,16 It has been indicated that the potential mechanisms deciphering the protective effects of genistein against metabolic disorders included directly enhancing the proliferation of β-cells, inhibiting apoptosis, promoting insulin secretion, epigenetic modifications, regulating the cAMP/PKA signaling pathway, and modulating the gut microbiota.12,17 However, studies exploring the effects and mechanism of perinatal genistein consumption on transgenerational metabolic health are lacking.
The gut microbiota, as an environmental factor, plays crucial roles in the metabolic health. Limited studies have found that genistein intake could significantly modify the imbalance of the gut microbiota induced by a high-fat diet or in type 1 diabetic mice.17,18 Thus, the role of the intestinal microbial community in the effects of genistein intake on metabolism still needs further exploration. In addition, recent research evidence challenged the traditional view of the intrauterine sterile environment, since the microbiota was detected in amniotic fluid, cord blood and placenta during normal pregnancy.19 Poor perinatal exposures could impair the balance of the gut microbiota in both mothers and offspring.20 Therefore, increasing studies have suggested that the gut microbiota might play a key role in the transgenerational effects of adverse early-life exposures on metabolism in later life. However, whether the genistein intake experienced by mothers could regulate the gut microbiota in both dams and offspring is unclear.
Therefore, we aimed to investigate the effects of perinatal genistein intake for 3 weeks prior to mating, and throughout pregnancy and lactation on glucose and lipid metabolism in both dams and female offspring. In addition, we further explored whether the gut microbiota plays vital roles in the transgenerational effects of genistein consumption on metabolic health.
2. Materials and methods
2.1 Animals and study design
C57BL/6 mice used in this experiment were obtained from the National Institutes for Food and Drug Control (Beijing, China, SYXK-2018-0019). The mice were kept in a controlled environment of room temperature at 22 ± 2 °C with a 12 h light/dark cycle. All mice had ad libitum access to food and sterile water during the study. After one week of acclimation, five-week-old female mice were randomly divided into three groups: the control group (MC, n = 8), which received a standard rodent diet (AIN-93G) (15.8% of the calories as fat); high-fat group (MHF, n = 8), which received a high-fat diet (60% of the calories as fat); and high-fat with genistein group (MHFG, n = 7), which received a high-fat diet with genistein (CAS: 466-72-0, G0272, TCI Development Co., Ltd) (0.6 g kg−1 diet). The ingredients are shown in ESI Table S1.† Females were fed these diets for 3 weeks prior to mating and throughout pregnancy and lactation.
After the first three weeks of intervention, the female mice were mated with normal control C57BL/6 males. At birth, the litters were adjusted to 5 pups for each dam to ensure that there was no nutritional bias between litters. The offspring were weaned at 3 weeks of age. At weaning, all dams were sacrificed, and female offspring from the three groups (FC, FHF and FHFG) (n = 7–8 per group) were given ad libitum access to a standard chow diet until 24 weeks of age. At the end of the experiment, one female offspring from different litters (n = 7–8 per group) was sacrificed. Blood samples were collected from the intraorbital retrobulbar plexus after 10 h of fasting from anesthetized mice, and the inguinal subcutaneous adipose tissue (SAT) and perirenal visceral adipose tissue (VAT) were removed and weighed; the cecal contents were quickly removed, snap frozen in liquid nitrogen, and then stored at −80 °C for further analysis in both dams and female offspring. All of the procedures were approved by the animal care and use committee of the Peking Union Medical College Hospital (Beijing, China, SYXC-2014-0029). All of the animal operations were conducted in compliance with the National Institutes of Health guide for the care and use of laboratory animals.
2.2 Body weight, gestational weight gain (GWG) and birth weight
The body weights of dams and female offspring were measured per week. The gestational weight gain was calculated from the body weight before delivery (6th week of intervention) minus that before mating (3rd week of intervention). At birth, the litters were weighed and the values were recorded as birth weights.
2.3 Fasting blood glucose (FBG) levels at the first week of pregnancy
To detect the effects of genistein intake on maternal blood glucose levels during pregnancy, we only measured the FBG levels at the first week of pregnancy based on concerns over safety. The dams were fasted for 6 h. The FBG levels were measured from tail vein blood using a Contour TS glucometer (ACCU-CHEK Mobile, Beijing, China).
2.4 Oral glucose tolerance tests (OGTT)
The OGTT were performed in dams at weaning and female offspring at 4, 12 and 24 weeks of ages. The mice were fasted for 6 h and given a glucose load (2 g per kg of body weight) by gavage. Blood glucose (BG) levels were measured at 0, 30, 60, and 120 min after the gavage also from the tail vein blood. The area under the curve (AUC) of the OGTT was calculated as previously described.21
2.5 Serum biochemical parameter measurement
The blood samples collected from dams at weaning and female offspring at 24 weeks of age were centrifuged at 3000g for 10 min at 4 °C, and the serum was stored in aliquots at −80 °C. The serum insulin concentrations, total cholesterol (TC), triacylglycerol (TG), high-density lipoprotein cholesterol (HDL-C), low-density lipoprotein cholesterol (LDL-C) and free fatty acids (FFA) were measured as previously described.22 Insulin sensitivity was assessed using the homeostasis model assessment of insulin resistance (HOMA-IR). The HOMA-IR was calculated as previously described.21
2.6 Gut microbiota analysis
To determine the effects of genistein consumption on the maternal and female offspring gut microbiota, we performed the 16s rDNA sequencing. Microbial DNA was extracted from the cecal contents of dams at weaning and female offspring at 24 weeks of age using a QIAamp DNA Stool Mini Kit (Qiagen, Hilden, Germany) according to manufacturer's protocols. The V3–V4 regions of the 16S rRNA genes were amplified using the primers 341F 5′-CCTACGGGRSGCAGCAG-3′ and 806R, 5′-GGACTACVVGGGTATCTAATC-3′. Amplicons were purified using an AxyPrep DNA Gel Extraction Kit (Axygen Biosciences, Union City, CA, U.S.) and quantified using Qubit ®2.0 (Invitrogen, U.S.). The tags were sequenced on the Illumina HiSeq platform (Illumina, Inc., CA, USA).
After merging paired-end reads, reads were performed by quality filtering. High quality reads were clustered into operational taxonomic units (OTUs) with a 97% similarity using UPARSE software (version 7.0.1001),23 and representative sequences for each OTU were screened using QIIME software (version 1.7.0, Quantitative Insights into Microbial Ecology).24 Then, the GreenGene Database25 was used to annotate taxonomic information based on the RDP classifier version 2.2 algorithm.26 Alpha and beta diversity analyses were performed using QIIME software (Version 1.7.0) and R software (Version 2.15.3). For alpha diversity, Chao1, observed_species and PD_whole_tree were analyzed. For beta diversity, principal coordinates analysis (PCoA), Adonis, and analysis of similarities (ANOSIM) were performed using unweighted UniFrac. In addition, linear discriminant analysis (LDA) of the effect size (LEfSe) was used to determine differences among the groups.
2.7 Statistical analysis
The data were expressed as mean ± standard error of the mean (S.E.M). The statistics were analyzed by one-way ANOVA and two-way ANOVA, with Tukey post hoc analyses. The differences in the relative abundances of the gut microbiota were analyzed by the Kruskal–Wallis test, with Benjamini and Hochberg post hoc analyses. Correlation analyses between the relative abundances of bacterial taxa at genus levels and metabolic parameters were performed by the Spearman correlation coefficient test. A p value < 0.05 was considered statistically significant. Prism version 7.0 (GraphPad Software Inc., San Diego, CA, USA) was used for statistical analysis.
3. Results
3.1 The effects of high-fat diet and genistein consumption on body weight as well as glucose and lipid metabolism in dams
As shown in Fig. 1a, there was no difference in the body weights among the three groups at the baseline and before the 6th week of intervention. Subsequently, the body weights of the dams in the MHF group were significantly higher than those in the MC group from the time of delivery (6th week of intervention) (p < 0.05) to weaning (9th week of intervention) (p < 0.0001). However, the GWG among the three groups were not significantly different. To determine the effects of high-fat diet and genistein consumption on glucose metabolism during pregnancy, we detected the FBG levels at the first week of pregnancy. After 4 weeks of intervention, HF fed dams had dramatically elevated FBG than that in the MC group (p < 0.0001), whereas genistein intake significantly reduced the FBG levels compared with those in the MHF group (p < 0.0001). At weaning, 9 weeks of HF led to significantly elevated BG levels at 30 min (p < 0.01), 60 min (p < 0.01) and 120 min (p < 0.05) during the OGTT and larger AUC values (p < 0.01) compared to those fed the control diet. However, perinatal genistein consumption did not alter the BG levels induced by HF in dams at weaning (Fig. 1d and e). In order to explore the effects of diet intervention on insulin sensitivity in dams, we measured the fasting serum insulin levels and calculated the HOMA-IR index. The results showed that perinatal 9 weeks of HF significantly elevated the serum insulin levels (p < 0.001) and the HOMA-IR index (p < 0.05) in the dams of the MHF group compared with those in the MC group, whereas genistein intake tended to reduce the fasting insulin levels induced by HF in dams at weaning (p = 0.05) (Fig. 1f and g).
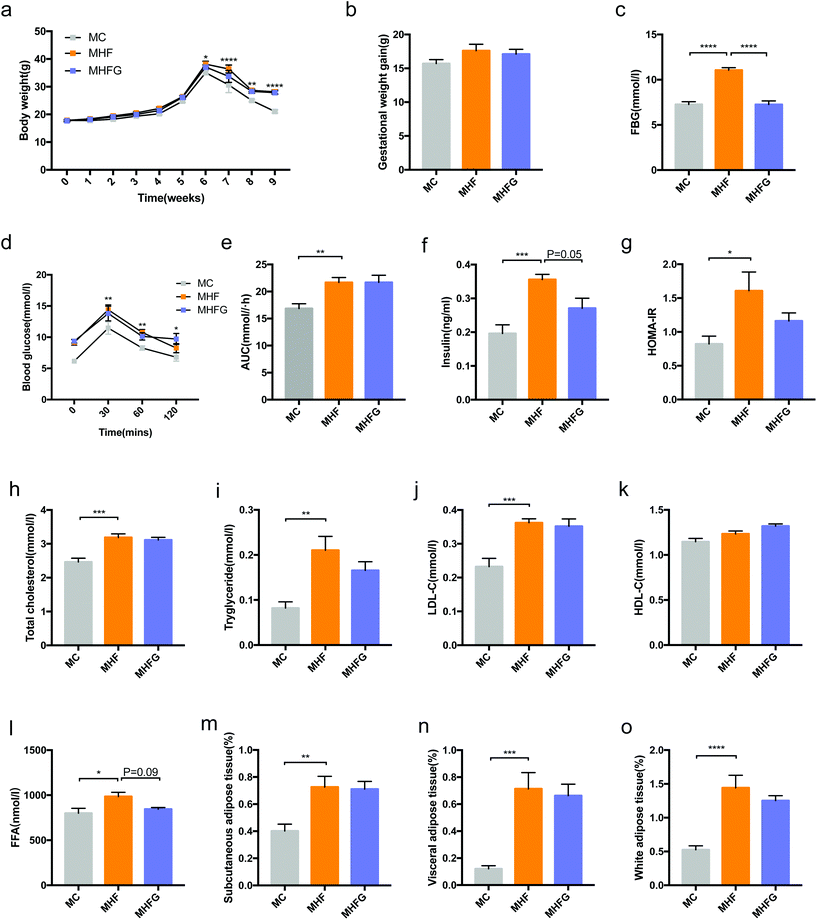 |
| Fig. 1 Metabolic parameters in dams. (a) Body weight changes between interventions; (b) GWG; (c) FBG at the first week of pregnancy; (d) OGTT; (e) AUC; (f) serum insulin levels; (g) HOMA-IR; (h) TC; (i) TG; (j) LDL-C; (k) HDL-C; (l) FFA; (m) relative content of subcutaneous adipose tissue; (n) relative content of visceral adipose tissue; and (o) relative content of white adipose tissue. MC, normal control diet in dams; MHF, high-fat diet in dams; MHFG, high-fat diet with genistein in dams; GWG, gestational weight gain; FBG, fasting glucose level; OGTT, oral glucose tolerance test; AUC, area under the curve; HOMA-IR, homeostasis model assessment of insulin resistance; TC, total cholesterol; TG, triacylglycerol; HDL-C, high-density lipoprotein cholesterol; LDL-C, low-density lipoprotein cholesterol; and FFA, free fatty acid. Data are expressed as means ± S.E.M. (n = 7–8 per group). Mean values were significantly different between the MC group and the MHF group in body weight and during the OGTT: *; mean values were significantly different between the groups: *p < 0.05, **p < 0.01, ***p < 0.001, ****p < 0.0001. | |
In addition to glucose metabolism, we further analyzed the effects of 9 weeks of diet intervention on lipid metabolism and fat mass in dams at weaning. As shown in Fig. 1h–l, HF fed dams had higher serum levels of TC (p < 0.001), TG (p < 0.01), LDL-C (p < 0.001) and FFA (p < 0.05) than those in the MC group. Perinatal genistein consumption tended to decrease the FFA levels elevated by HF in the MHFG fed dams (p = 0.09). Furthermore, in comparison with the dams of the MC group, perinatal HF exposure also dramatically increased the relative SAT (p < 0.01), VAT (p < 0.001) and WAT (SAT + VAT) (p < 0.0001) mass in the dams of the MHF group. Nevertheless, perinatal 9 weeks of genistein intake did not significantly change the relative fat mass in dams at weaning.
3.2 The changes of the gut microbiota in dams at weaning
To investigate the effects of perinatal diet intervention on the gut microbiota in dams at weaning, we performed the 16s rDNA sequencing for the cecal contents. The sequencing data have been submitted to the Sequence Read Archive (SRA) database (PRJNA543285). Firstly, we assessed the OTUs among the three groups to identify the shared and unique species. A Venn diagram showed that there were 275 shared OTUs, 56 unique OTUs in the dams of the MC group, 2 unique OTUs in HF fed dams and 8 unique OTUs in the dams of the MHFG group (Fig. 2a). Then we evaluated the composition of the gut microbial community in dams. At the phylum level, Firmicutes, Bacteroidetes, Verrucomicrobia and Proteobacteria were the most abundant, whereas the relative abundances of the four phyla were not significantly different among the three groups (Fig. 2b). The abundance of phylum Deferribacteres was dramatically elevated in the HF fed dams than that in the MC group (q < 0.05), and perinatal genistein intake could significantly reduce this phylum (q < 0.01). Fig. 2c shows the relative abundances of the top 20 species at the genus level among the three groups. The abundances of Odoribacter (q < 0.05) and Mucispirillum (q < 0.05) were significantly higher in HF fed dams compared with those in the MC group, and were dramatically decreased (q < 0.05, q < 0.01) in dams fed genistein (MHFG group). In contrast, 9 weeks of HF resulted in lower abundances of Barnesiella (q < 0.05), Clostridium XIVa (q < 0.05), Clostridium IV (q < 0.01), Eubacterium (q < 0.05) and Bifidobacterium (q < 0.001) in the dams of the MHF group compared to those of the MC group. Perinatal addition of genistein to the HF could dramatically increase the abundance of Allobaculum (q < 0.05), Barnesiella (q = 0.07), Clostridium XIVa (q < 0.05) and Eubacterium (q < 0.05) (Fig. 2d). Significantly different species at the genus level among the three groups were summarized in a heatmap (Fig. 2e).
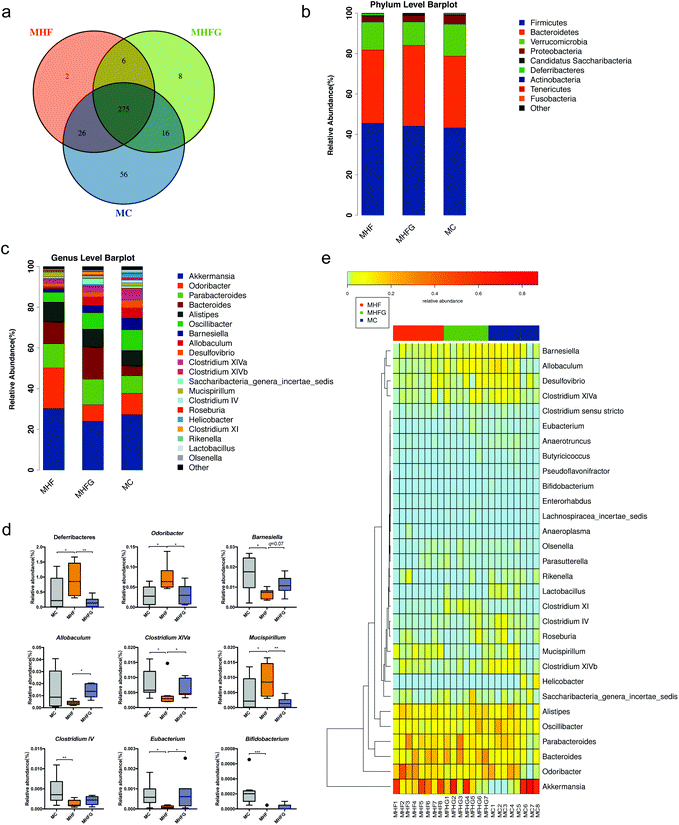 |
| Fig. 2 The changes of the gut microbiota in dams at weaning. (a) Venn diagram of the OUTs; (b) relative abundances of the top ten phyla; (c) relative abundances of the top twenty genera; (d) significantly different germs at the phylum and genus levels; and (e) heat map analysis of the different genera among the three groups. MC, normal control diet in dams; MHF, high-fat diet in dams; and MHFG, high-fat diet with genistein in dams. Data are expressed as means ± S.E.M. (n = 7–8 per group). Mean values were significantly different between the groups: *q < 0.05, **q < 0.01, ***q < 0.001. | |
Then we evaluated the structure of the gut microbiota in dams among the three groups. Alpha diversity analysis showed that HF fed dams had significantly decreased community richness and diversity compared with the mice in the MC group (Fig. 3a–c). No significant differences in alpha diversity were observed between the mice in the MHFG group and the MHF group. PCoA based on unweighted UniFrac distances showed that the gut microbial community was significantly separated in dams among the three groups (Fig. 3d). ANOSIM analysis demonstrated that the differences among the groups were significant (R = 0.686, p = 0.001) (Fig. 3e).
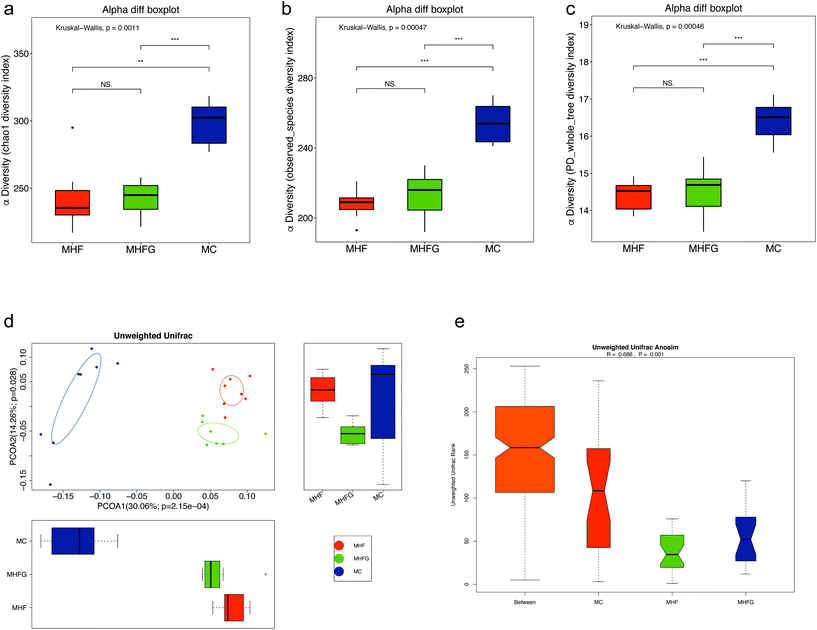 |
| Fig. 3 Alpha-diversity and beta-diversity analyses of the gut microbiota in dams at weaning. (a) Chao1 index; (b) observed_species index; (c) PD_whole_tree index; (d) PCoA plots of gut communities; and (e) unweighted Unifrac ANOSIM analysis between the three groups (n = 7–8 per group). MC, normal control diet in dams; MHF, high-fat diet in dams; and MHFG, high-fat diet with genistein in dams. Data are expressed as means ± S.E.M. (n = 7–8 per group). Mean values were significantly different between the groups: *p < 0.05, **p < 0.01, ***p < 0.001. | |
3.3 The effects of maternal high-fat diet and genistein intake on energy metabolic parameters in female offspring
In addition to the influences of perinatal genistein consumption on dams, we further detected the effects of maternal HF and genistein intake on body weight as well as glucose and lipid metabolism in female offspring. As shown in Fig. 4a, maternal HF led to significantly lower birth weight in offspring compared with that of the control diet fed dams (p < 0.01). However, the body weight was dramatically higher in female offspring at the end of lactation (3rd week) in the FHF group than that in the FC group (p < 0.0001). Thus, a catch-up growth existed in the offspring of the HF fed dams (Fig. 4b). Subsequently, there were no differences in body weights between female offspring in the FHF group and the FC group. Nevertheless, no effects of maternal genistein intake on the birth weight or body weight induced by HF were found in female offspring.
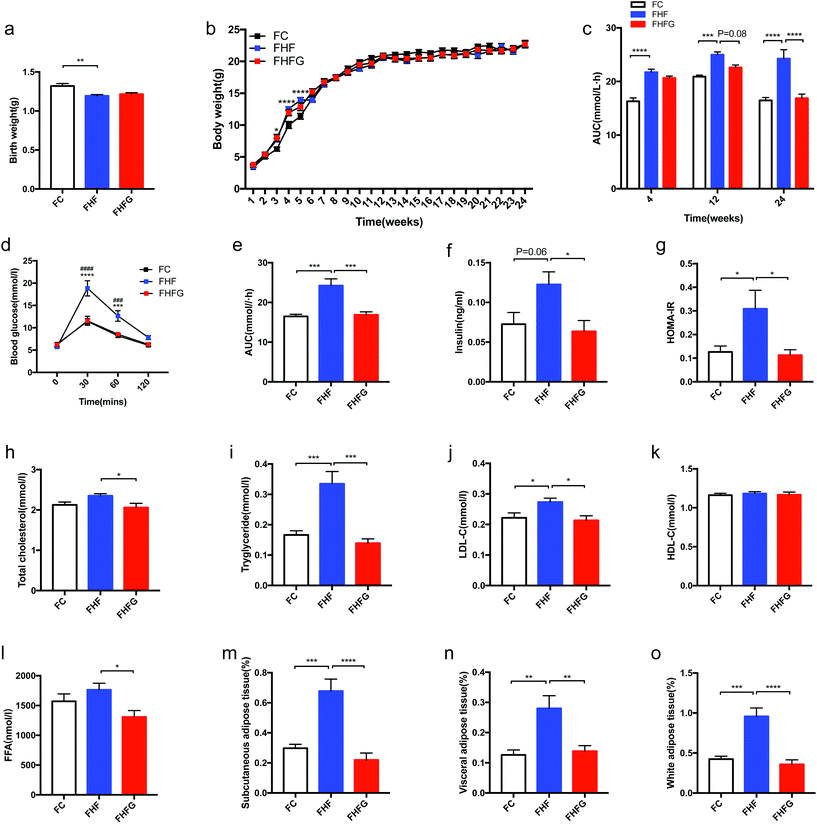 |
| Fig. 4 Metabolic parameters in female offspring. (a) Birth weight; (b) body weight from birth to 24 weeks of age; (c) AUC at 4, 12 and 24 weeks of ages; (d) OGTT; (e) AUC; (f) serum insulin levels; (g) HOMA-IR; (h) TC; (i) TG; (j) LDL-C; (k) HDL-C; (l) FFA; (m) relative content of subcutaneous adipose tissue; (n) relative content of visceral adipose tissue; and (o) relative content of white adipose tissue. FC, female offspring of normal control diet fed dams; FHF, female offspring of high-fat diet fed dams; FHFG, female offspring of high-fat diet with genistein fed dams; OGTT, oral glucose tolerance test; AUC, area under the curve; HOMA-IR, homeostasis model assessment of insulin resistance; TC, total cholesterol; TG, triacylglycerol; HDL-C, high-density lipoprotein cholesterol; LDL-C, low-density lipoprotein cholesterol; and FFA, free fatty acid. Data are expressed as means ± S.E.M. (n = 7–8 per group). Mean values were significantly different between the FC group and the FHF group in body weight and during the OGTT: *; mean values were significantly different between the FHFG group and the FHF group during the OGTT: #. Mean values were significantly different between the groups: *p < 0.05, **p < 0.01, ***p < 0.001, ****p < 0.0001. | |
Maternal HF during 3 weeks before mating and throughout pregnancy and lactation resulted in significantly larger AUC of the OGTT in female offspring from early life (4 weeks of age) (p < 0.0001) to adult life (12 and 24 weeks of age) than that of the C fed dams (p < 0.001, p < 0.0001). Although there were no effects of perinatal genistein intake on glucose tolerance in early life of female offspring, the AUC tended to decrease at 12 weeks of age (p = 0.08) and was significantly reduced when the mice were 24 weeks of age compared with those in the FHF group (p < 0.0001). In the meantime, perinatal genistein consumption reduced the elevated BG levels at 30 min (p < 0.0001) and 60 min (p < 0.001) induced by maternal HF in female offspring. Furthermore, we analyzed the transgenerational effects of diet intervention on insulin sensitivity in female offspring at 24 weeks of age. The results showed that serum insulin levels (p = 0.06) and HOMA-IR (p < 0.05) of offspring in the FHF group were significantly higher than those from the FC group. Maternal dietary genistein prevented the deleterious effects of maternal HF on insulin sensitivity, with significantly lower insulin levels (p < 0.05) and HOMA-IR (p < 0.05) (Fig. 4f and g).
Moreover, the transgenerational effects on lipid metabolism were also assessed. The fasting serum TG (p < 0.001) and LDL-C (p < 0.05) were significantly elevated in female offspring at 24 weeks of age by maternal HF compared with that of the control diet fed dams. Maternal genistein consumption could dramatically reduce the TC (p < 0.05), TG (p < 0.001), LDL-C (p < 0.05) and FFA (p < 0.05) levels in the dams of the FHFG group compared to those of the FHF group (Fig. 4h, i, j and l). No significant differences were observed in serum HDL-C levels among the three groups (Fig. 4k). Although no differences were found in body weights in female offspring at 24 weeks of age, we further detected whether fat mass was influenced. Interestingly, maternal HF significantly increased the relative mass of SAT (p < 0.001), VAT (p < 0.01) and WAT (p < 0.001) in offspring, which were all dramatically reduced by perinatal genistein intake (p < 0.0001, p < 0.01, and p < 0.0001, respectively) (Fig. 4m, n and o).
3.4 The alterations of the gut microbiota in female offspring at 24 weeks of age
Next, we analyzed how maternal genistein intake modulated the gut microbiota in female offspring at 24 weeks of age. A Venn diagram showed that there were 301 shared OTUs among the three groups, 11 unique OTUs in the female offspring of the control diet fed dams, 21 OTUs in the offspring of the FHF group and 7 unique OTUs in the offspring of the genistein fed dams (Fig. 5a). Fig. 5b shows the top 10 phyla among the three groups. The relative abundances of Bacteroidetes (q < 0.01) and Proteobacteria (q < 0.05) were significantly increased in the female offspring of the HF fed dams compared with those of control diet fed dams, and were dramatically reduced by maternal genistein supplementation (q < 0.05, q < 0.001). The top 20 species at the genus level are shown in Fig. 5c. Maternal HF dramatically elevated the abundance of Alloprevotella (q < 0.05) while reducing the abundance of Bifidobacterium (q < 0.01) in female offspring compared with that in the FC group. Perinatal genistein intake significantly decreased the Alloprevotella in female offspring (q < 0.05) (Fig. 5d). The different germs at the genus level in female offspring at 24 weeks of age among the three groups are summarized in Fig. 5e.
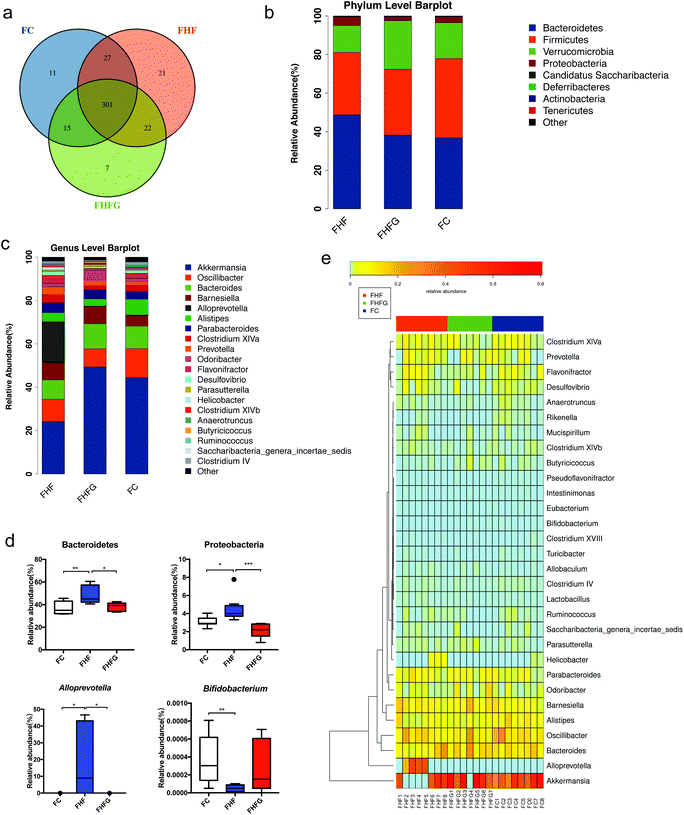 |
| Fig. 5 The changes of the gut microbiota in adult offspring. (a) Venn diagram of the OTUs; (b) relative abundances of the top ten phyla; (c) relative abundances of the top twenty genera; (d) significantly different germs at the phylum and genus levels; and (e) heat map analysis of the different genera among the three groups. FC, female offspring of normal control diet fed dams; FHF, female offspring of high-fat diet fed dams; and FHFG, female offspring of high-fat diet with genistein fed dams. Data are expressed as means ± S.E.M. (n = 7–8 per group). Mean values were significantly different between the groups: *q < 0.05, **q < 0.01, ***q < 0.001. | |
The structure of the gut microbiota in female offspring was also assessed. Alpha diversity analysis showed that there were parallel community richness and diversity among the three groups. PCoA based on unweighted Unifrac distances demonstrated that the microbiota community in female offspring among the groups was dramatically separated, which was supported by ANOSIM analysis (R = 0.42, p = 0.001) (Fig. 6a and b).
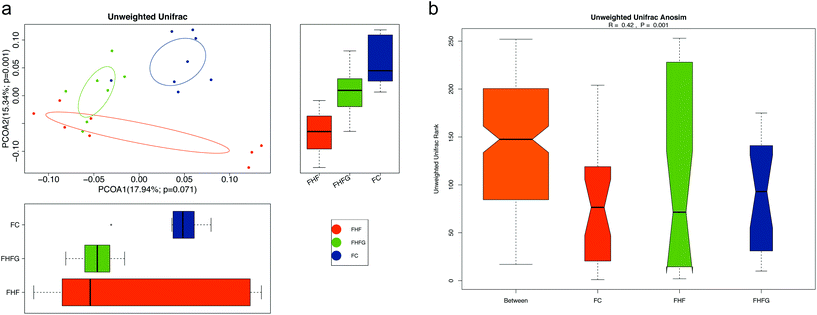 |
| Fig. 6 Beta-diversity analysis of the gut microbiota in the adult female offspring (n = 7–8 per group). (a) PCoA plots of gut communities; and (b) unweighted Unifrac ANOSIM analysis between the three groups. FC, female offspring of normal control diet fed dams; FHF, female offspring of high-fat diet fed dams; and FHFG, female offspring of high-fat diet with genistein fed dams. | |
3.5 The significantly enriched microbiota at different levels in dams and female offspring
We next identified individual microbial species which differed between groups from the phylum level to the genus level in dams and offspring, respectively. At the genus level, maternal high-fat diet significantly enriched Odoribacter from the Odoribacteraceae family and Mucispirillum from the Deferribacteraceae family, while Allobaculum from Erysipelotrichaceae, Eisenbergiella from Lachnospiraceae, and Clostridium IV from Ruminococcaceae, Lactobacillus, and Rikenella were abundant in control diet fed dams. Perinatal genistein consumption enriched the genus Eubacterium and some other germs in dams at weaning (Fig. 7a). As for female adult offspring, Alloprevotella from the Prevotellaceae family was significantly increased in the FHF group. Control diet fed dams resulted in significant enrichment of Parvibacter, Bifidobacterium and Rikenella in female offspring. The genus Erysipelotrichaceae_incertae_sedis from the Erysipelotrichaceae family was significantly enriched in the female offspring of genistein fed dams (Fig. 7b).
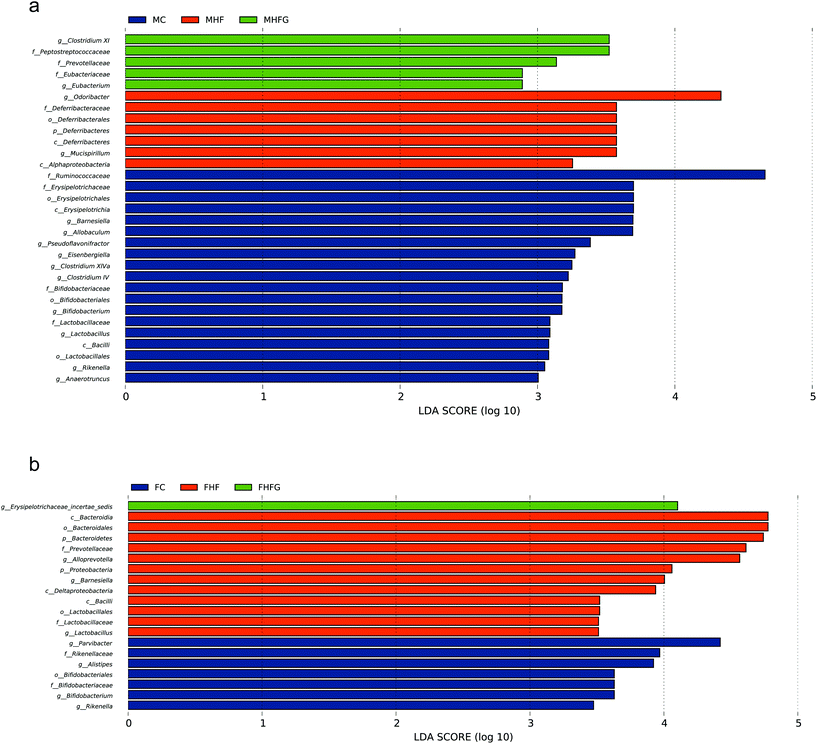 |
| Fig. 7 LEfSe analysis of the different gut microbiota from the phylum level down to the genus level (n = 7–8 per group). (a) Among three groups in dams; and (b) among three groups in female offspring. MC, normal control diet in dams; MHF, high-fat diet in dams; MHFG, high-fat diet with genistein in dams. FC, female offspring of normal control diet fed dams; FHF, female offspring of high-fat diet fed dams; and FHFG, female offspring of high-fat diet with genistein fed dams. | |
3.6 Correlation analysis of the differential bacteria at the genus level and metabolic parameters in dams and female offspring
In order to analyze the relationship between the gut microbiota and metabolism, we performed a correlation analysis between the abundance of significantly altered bacteria at the genus level and metabolic parameters in both dams and female offspring. Fig. 8 shows the relationship between the parameters and gut microbiota in dams. The significantly enriched genus Eubacterium in the MHFG group was negatively correlated with the FBG during pregnancy, BG60 during the OGTT and LDL-C in dams. The relative abundances of Clostridium XIVa, Clostridium IV and Barnesiella, which were elevated by 9 weeks of genistein intake, were all negatively correlated with the FBG during pregnancy, HOMA-IR, and TG and LDL-C levels in dams. In contrast, the significantly reduced Odoribacter and Mucispirillum in the dams of the MHFG group were positively related to the FBG levels during pregnancy and BG60 during the OGTT. As for female offspring, elevated Erysipelotrichaceae_incertae_sedis by maternal genistein intake in adult female offspring was negatively correlated with BG0 during the OGTT, serum insulin levels, and SAT and WAT mass. However, the relative abundance of Alloprevotella, which was dramatically reduced in the female offspring of the genistein fed dams compared with that in the FHF group, was positively correlated with the serum TC levels, and SAT, VAT and WAT mass (Table 1). Interestingly, the relative abundance of Bifidobacterium was significantly decreased in both the dams of the MHF group and the female offspring of the FHF group compared with that in the control diet fed dams. It was negatively correlated with not only the BG levels during the OGTT, insulin levels, HOMA-IR, serum lipid profiles (TG, TC and LDL-C) and fat mass in dams, but also the insulin levels, HOMA-IR, serum LDL-C levels, FFA and fat mass in adult female offspring.
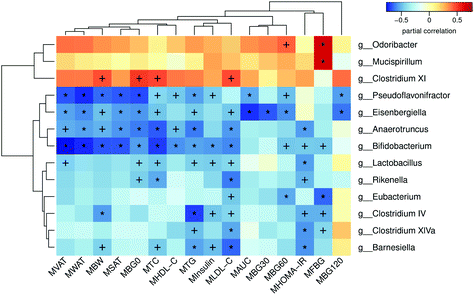 |
| Fig. 8 Heatmap of Spearman correlation analysis between the altered genera and metabolic parameters in dams. MBG0, blood glucose level at 0 min of OGTT; MBG30, blood glucose level at 30 min of OGTT; MBG60, blood glucose level at 60 min of OGTT; MBG120, blood glucose level at 120 min of OGTT; MAUC, area under the curve of the OGTT; MFBG, fasting blood glucose at the first week of pregnancy; MHOMA-IR, the homeostasis model assessment of insulin resistance; MTC, total cholesterol; MTG, triglyceride; MLDL-C, low-density lipoprotein cholesterol; MHDL-C, high-density lipoprotein cholesterol; MSAT, subcutaneous adipose tissue mass; MVAT, visceral adipose tissue mass; MWAT, white adipose tissue mass; MBW, body weight. Values were significantly correlated between the genera and metabolic parameters: +p < 0.05; *p < 0.01. | |
Table 1 Correlation analysis between the relative abundance of altered species at the genus level and metabolic parameters in adult female offspring
Genera |
Parameters |
r
|
p value |
HOMA-IR, homeostasis model assessment of insulin resistance; LDL-C, low-density lipoprotein cholesterol; FFA, free fatty acid; SAT, subcutaneous adipose tissue; WAT, white adipose tissue; BG, blood glucose; TC, total cholesterol; and VAT, visceral adipose tissue. |
Bifidobacterium
|
Insulin |
−0.6450 |
0.0009 |
HOMA-IR |
−0.6359 |
0.0011 |
LDL-C |
−0.5499 |
0.0066 |
FFA |
−0.4947 |
0.0164 |
SAT |
−0.4410 |
0.0352 |
WAT |
−0.4987 |
0.0154 |
Erysipelotrichaceae_incertae_sedis
|
BG0 |
−0.5345 |
0.0086 |
Insulin |
−0.4668 |
0.0247 |
SAT |
−0.4294 |
0.0409 |
WAT |
−0.4283 |
0.0415 |
Alloprevotella
|
TC |
0.5927 |
0.0029 |
SAT |
0.4702 |
0.0236 |
VAT |
0.4413 |
0.0350 |
WAT |
0.4901 |
0.0176 |
4. Discussion
The global prevalence of chronic metabolic diseases has imposed enormous financial burden. Therefore, it is urgent to clarify the pathogenesis and develop new strategies to fight against the development of metabolic disturbances and obesity. In recent years, it has been widely reported that adverse early-life exposures could significantly increase the risks of metabolic disorders in later life.2,27 Consistent with the previous studies, our research also showed that perinatal 9 weeks of a high-fat diet led to significantly higher body weight, increased fat mass, glucose intolerance, insulin resistance and disorders of serum lipid profiles in not only dams at weaning but also adult female offspring. Maternal high-fat diet intervention during 3 weeks prior to mating, and throughout pregnancy and lactation led to an overnutritional early-life environment, which programmed abnormal metabolism in offspring. Thus, the perinatal period might be a critical window for preventing the occurrence and development of metabolic disorders.
The metabolic benefits of bioactive components have been extensively studied. Genistein is one of the major components of soy isoflavone. Both clinical trials and experimental animal models showed the protective effects of genistein supplementation against glucose and lipid metabolic disorders.15,17 However, it is still unclear whether early-life genistein intake could inhibit the transgenerational effects of perinatal overnutrition exposures on metabolism. Based on the proved safety of genistein intake in both animals and humans,12 to the best of our knowledge, this is the first study to investigate whether perinatal addition of genistein could counteract the deleterious effects of maternal high-fat diet on metabolism in both dams and female offspring. Our study showed that perinatal genistein consumption significantly reduced the FBG levels at the first week of pregnancy and decreased the serum insulin and FFA levels induced by high-fat diet in dams at weaning. In addition, there were larger metabolic benefits in adult female offspring. Perinatal addition of genistein to high-fat diet significantly programmed improved glucose tolerance, insulin sensitivity and serum lipid profiles, as well as lower fat mass in female offspring at 24 weeks of age, which were independent of the body weight. Therefore, this study confirmed the transgenerational metabolic benefits of perinatal genistein consumption.
Given the vital role of the gut microbiota in host health, we hypothesized that the intestinal microbial community might play crucial roles in deciphering the transgenerational metabolic benefits of genistein consumption. Indeed, our results showed that perinatal high-fat diet significantly increased the relative abundances of Odoribacter and Mucispirillum in dams at weaning. The genus Odoribacter has been reported to be associated with opportunistic infections, particularly intra-abdominal and systemic infections.28 Walker et al.29 found an unusual class of bacterial sulfonolipids in mice fed the high-fat diet and showed that Odoribacter was responsible for their production. The positive correlation between the abundance of Odoribacter and the adiposity index and visceral fat has been demonstrated in a human study.30 In addition, Granado-Serrano et al.31 showed that men with hypercholesterolemia were characterized by the higher relative abundance of Odoribacter, which was also significantly correlated with the lipid parameters. In contrast, Garcia-Mazcorro et al.32 showed reduced abundance of Odoribacter in obese diabetic db/db mice supplemented with raspberry. Oligosaccharide supplementation could increase the levels of short-chain fatty acids while reducing the abundance of Odoribacter in the feces of mice.33 Consistent with these studies, our research also indicated that Odoribacter was an unfavorable species for metabolism, which was further confirmed by the positively correlation between Odoribacter and maternal blood glucose levels. Mucispirillum is Gram-negative bacteria and is inhabited in the intestinal mucus layer. However, it is an important mucin degrader,34,35 and could influence the intestinal permeability. The relationship between the abundance of Mucispirillum and intestinal inflammation has been confirmed.36,37 Xiao et al. showed that Paederia scandens extracts could improve arthritis through reducing Mucispirillum.38 The beneficial effects of dietary fiber, inulin and probiotic supplementation on metabolism and inflammation in diet-induced obese mice or db/db mice have also been reported to be associated with the decrease of Mucispirillum.39–41 Our study also showed that significantly increased Mucispirillum induced by the high-fat diet was positively correlated with the maternal FBG levels. Nevertheless, perinatal genistein consumption dramatically reduced the unfavorable species elevated by the high-fat diet, which might be associated with the improvement of metabolism in dams.
In addition, this study also showed that perinatal genistein supplementation markedly enriched Allobaculum, Clostridium XIVa, Clostridium IV, Eubacterium and Barnesiella in dams at weaning, which were all reported to be the short-chain fatty acid (SCFA) (mainly butyrate)-producing bacteria. These SCFA producers have been shown to have an important physiological function, especially for metabolism. The benefits of Allobaculum have been shown in several studies. High-fat diet significantly reduced the abundance of Allobaculum. In contrast, prebiotic, berberine, as well as berberine and metformin treatment could significantly increase SCFA-producing Allobaculum and improve obesity induced by the high-fat diet, and accordingly, the intestinal SCFAs were also elevated.42–44 Studies have demonstrated that most of the butyrate-producing species belong to the clostridial clusters XIVa and IV, including Clostridium XIVa, Clostridium IV and Eubacterium.45,46 Evidence from both patients with obesity or T2DM47,48 and animals fed high-fat sucrose diets49 showed that Clostridium XIVa and Clostridium IV were dramatically reduced, while losing weight could elevate the abundance of Clostridium IV.50 Another clinical trial indicated that Eubacterium was significantly reduced in patients with gestational diabetes mellitus.51 In addition, conversion of acetate to butyrate has been reported to be one of the important roles of Eubacterium in improving metabolism.52 As a member of the Barnesiellaceae family, Barnesiella is also a SCFA producer.53 It has recently been reported that multiple bioactive components, such as mannoligosaccharides (MOS) from spent coffee grounds,54 polyphenol-rich cranberry extracts,55Grifola frondosa polysaccharides,56 polyunsaturated fatty acids from Spirulina platensis,57 and decaffeinated green and black tea polyphenols,58 might regulate glucose homeostasis, insulin sensitivity and lipid metabolism as well as modulate body weight through modifying gut microbiota, including increasing the abundance of Barnesiella. Moreover, improving T2DM with a traditional Chinese medicine, Xiexin Tang, was also associated with enriched Barnesiella.53 Anti-inflammation might play an important role in the benefits of Barnesiella.53,59,60 Consistent with these findings, our study showed that the markedly enriched SCFA-producing bacteria in the MHFG group were negatively correlated with the glucose and lipid metabolic parameters as well as body weights in dams.
Therefore, our study indicated that the beneficial effects of perinatal genistein intake on metabolism in dams was associated with the significant enrichment of SCFA (mainly butyrate)-producing bacteria. Most of these bacteria could produce butyrate and play important roles in the host health. Butyrate is one of the SCFAs resulting from the fermentation of dietary fiber in the gastrointestinal tract by the microbiota. Butyrate is not only the main energy supply of the colonic epithelium thus promoting its growth, but it also mitigates inflammatory responses through activation of its target G-protein coupled receptors and the histone deacetylase inhibitor activity, potentially protecting the gut permeability.61 In addition, it could pass through the enterocytes and into the circulation.62 Thus, the butyrate could also reach and affect the peripheral tissues. The benefits of butyrate supplementation against obesity and diabetes were shown to be associated with promoting mitochondrial adaptation by increasing β-oxidation of fatty acids and inhibiting ectopic lipid deposition and inflammation.61 However, in the present study, the levels of butyrate and the specific mechanism that decipher the metabolic benefits of butyrate-producing bacteria are unclear and need further exploration.
The transgenerational metabolic protection of maternal genistein intake against high-fat diet was also accompanied by changed gut microbiota in adult female offspring, including decreased Alloprevotella, while exhibiting enriched Erysipelotrichaceae_incertae_sedis from the Erysipelotrichaceae family. Alloprevotella was considered a harmful species, and weight loss was associated with the decrease of Alloprevotella in obese patients.63 Subsequently, Kang et al.64 also showed that supplementation of dietary fiber-konjac flour could significantly improve metabolism and inflammation, as well as reduce fat mass in high-fat fed mice, and was related to the decreased abundance of Alloprevotella. In this study, we showed that maternal genistein intake transgenerationally programmed an improvement of metabolism and reduction of fat mass in adult female offspring and decreased Alloprevotella, which was positively correlated with the TC and fat mass. Although the function of Erysipelotrichaceae_incertae_sedis is still unclear, this research indicated that it was beneficial bacteria and had negative correlation with the blood glucose levels, insulin levels and fat mass in female offspring. Therefore, this study demonstrated that the significant enrichment of Erysipelotrichaceae_incertae_sedis while a decrease of Alloprevotell might play a crucial role in the transgenerational metabolic benefits of perinatal genistein consumption in adult female offspring.
Furthermore, Bifidobacterium is another genus which plays crucial roles in the metabolic benefits of perinatal genistein consumption in both dams and adult female offspring in this study, and was significantly reduced in the high-fat diet fed mice and was negatively correlated with the glucose and lipid metabolic parameters. Although the abundance of Bifidobacterium is relatively low in adults, it has disproportionally large impacts in the host health. It has been widely accepted that Bifidobacterium has multiple functions, including the production of antioxidants and acids, maturation of the immune system, maintenance of immune homeostasis, and protection of the gut barrier.65 Although the beneficial effects of Bifidobacterium on metabolism have been extensively researched, to our knowledge, this is the first study to show the relationship between the metabolic benefits of genistein consumption and Bifidobacterium, especially the transgenerational effects.
5. Conclusions
Overall, perinatal genistein consumption during 3 weeks prior to mating and throughout pregnancy and lactation significantly counteracted the deleterious effects of high-fat diet on glucose and lipid metabolism in both dams and adult female offspring. Markedly enriched SCFA (mainly butyrate)-producing bacteria and reduced harmful species were obviously associated with the metabolic benefits of perinatal genistein intake in mothers. A significant decrease of Alloprevotella and enrichment of Erysipelotrichaceae_incertae_sedis might play crucial roles in the protective effects of maternal genistein intake on female offspring. In addition, Bifidobacterium was associated with the metabolic improvement in both dams and female offspring. To the best of our knowledge, this is the first study to indicate that gut microbiota might be a crucial factor in deciphering the metabolic benefits of perinatal genistein consumption in dams and adult female offspring. However, there are several limitations in this study. First, the fecal SCFA levels were not detected. Furthermore, the specific mechanism by which the gut microbiota mediates the transgenerational metabolic benefits of genistein supplementation remains largely unknown. Last, it is still unclear how maternal genistein intake programs the changes of the gut microbiome in later life of offspring and whether the maternal gut microbiota could influence the colonization and establishment of the microbial community in offspring, which require further investigation. All in all, our study might provide some evidence and new targets during early life for preventing the occurrence and development of metabolic disorders.
Abbreviations
HF | High-fat diet |
FFA | Free fatty acid |
T2DM | Type 2 diabetes mellitus |
GDM | Gestational diabetes mellitus |
DOHaD | Developmental origins of health and disease |
SAT | Subcutaneous adipose tissue |
VAT | Visceral adipose tissue |
WAT | White adipose tissue |
GWG | Gestational weight gain |
FBG | Fasting glucose level |
OGTT | Oral glucose tolerance test |
BG | Blood glucose |
AUC | Area under the curve |
TC | Total cholesterol |
TG | Triacylglycerol |
HDL-C | High-density lipoprotein cholesterol |
LDL-C | Low-density lipoprotein cholesterol |
HOMA-IR | Homeostasis model assessment of insulin resistance |
OTUs | Operational taxonomic units |
PCoA | Principal coordinates analysis |
ANOSIM | Analysis of similarities |
LEfSe | Linear discriminant analysis of the effect size |
SCFA | Short-chain fatty acid |
Conflicts of interest
There are no conflicts of interest to declare.
Acknowledgements
This work was supported by the grants from the National Natural Science Foundation of China (No. 81170736, 81570715, 81870579, and 81870545). We are very grateful to Realbio Genomics Institute (Shanghai, China) for their technical support with 16s rDNA sequences.
References
- C. Sun, D. P. Burgner, A. L. Ponsonby, R. Saffery, R. C. Huang, P. J. Vuillermin, M. Cheung and J. M. Craig, Effects of early-life environment and epigenetics on cardiovascular disease risk in children: highlighting the role of twin studies, Pediatr. Res., 2013, 73, 523–530 CrossRef CAS PubMed.
- K. Y. Yam, E. F. G. Naninck, M. R. Abbink, S. E. la Fleur, L. Schipper, J. C. van den Beukel, A. Grefhorst, A. Oosting, E. M. van der Beek, P. J. Lucassen and A. Korosi, Exposure to chronic early-life stress lastingly alters the adipose tissue, the leptin system and changes the vulnerability to western-style diet later in life in mice, Psychoneuroendocrinology, 2017, 77, 186–195 CrossRef CAS PubMed.
- E. Zambrano, C. Ibanez, P. M. Martinez-Samayoa, C. Lomas-Soria, M. Durand-Carbajal and G. L. Rodriguez-Gonzalez, Maternal obesity: lifelong metabolic outcomes for offspring from poor developmental trajectories during the perinatal period, Arch. Med. Res., 2016, 47, 1–12 CrossRef PubMed.
- D. J. Hoffman, R. M. Reynolds and D. B. Hardy, Developmental origins of health and disease: current knowledge and potential mechanisms, Nutr. Rev., 2017, 75, 951–970 CrossRef PubMed.
- C. E. Cuthbert, J. E. Foster and D. D. Ramdath, A maternal high-fat, high-sucrose diet alters insulin sensitivity and expression of insulin signalling and lipid metabolism genes and proteins in male rat offspring: effect of folic acid supplementation, Br. J. Nutr., 2017, 118, 580–588 CrossRef CAS PubMed.
- H. Yokomizo, T. Inoguchi, N. Sonoda, Y. Sakaki, Y. Maeda, T. Inoue, E. Hirata, R. Takei, N. Ikeda, M. Fujii, K. Fukuda, H. Sasaki and R. Takayanagi, Maternal high-fat diet induces insulin resistance and deterioration of pancreatic beta-cell function in adult offspring with sex differences in mice, Am. J. Physiol.: Endocrinol. Metab., 2014, 306, E1163–E1175 CrossRef CAS PubMed.
- J. Zheng, X. Xiao, Q. Zhang, M. Yu, J. Xu, Z. Wang, C. Qi and T. Wang, Maternal and post-weaning high-fat, high-sucrose diet modulates glucose homeostasis and hypothalamic POMC promoter methylation in mouse offspring, Metab. Brain Dis., 2015, 30, 1129–1137 CrossRef CAS PubMed.
- J. Sung, C. T. Ho and Y. Wang, Preventive mechanism of bioactive dietary foods on obesity-related inflammation and diseases, Food Funct., 2018, 9, 6081–6095 RSC.
- P. Zhou, C. Wang, Z. Hu, W. Chen, W. Qi and A. Li, Genistein induces apoptosis of colon cancer cells by reversal of epithelial-to-mesenchymal via a Notch1/NF-kappaB/slug/E-cadherin pathway, BMC Cancer, 2017, 17, 813 CrossRef PubMed.
- H. Marini, L. Minutoli, F. Polito, A. Bitto, D. Altavilla, M. Atteritano, A. Gaudio, S. Mazzaferro, A. Frisina, N. Frisina, C. Lubrano, M. Bonaiuto, R. D'Anna, M. L. Cannata, F. Corrado, E. B. Adamo, S. Wilson and F. Squadrito, Effects of the phytoestrogen genistein on bone metabolism in osteopenic postmenopausal women: a randomized trial, Ann. Intern. Med., 2007, 146, 839–847 CrossRef PubMed.
- H. Marini, A. Bitto, D. Altavilla, B. P. Burnett, F. Polito, V. Di Stefano, L. Minutoli, M. Atteritano, R. M. Levy, N. Frisina, S. Mazzaferro, A. Frisina, R. D'Anna, F. Cancellieri, M. L. Cannata, F. Corrado, C. Lubrano, R. Marini, E. B. Adamo and F. Squadrito, Efficacy of genistein aglycone on some cardiovascular risk factors and homocysteine levels: A follow-up study, Nutr. Metab. Cardiovasc. Dis., 2010, 20, 332–340 CrossRef CAS PubMed.
- E. R. Gilbert and D. Liu, Anti-diabetic functions of soy isoflavone genistein: mechanisms underlying its effects on pancreatic beta-cell function, Food Funct., 2013, 4, 200–212 RSC.
- M. Ding, A. Pan, J. E. Manson, W. C. Willett, V. Malik, B. Rosner, E. Giovannucci, F. B. Hu and Q. Sun, Consumption of soy foods and isoflavones and risk of type 2 diabetes: a pooled analysis of three US cohorts, Eur. J. Clin. Nutr., 2016, 70, 1381–1387 CrossRef CAS PubMed.
- A. Nanri, T. Mizoue, Y. Takahashi, K. Kirii, M. Inoue, M. Noda and S. Tsugane, Soy product and isoflavone intakes are associated with a lower risk of type 2 diabetes in overweight Japanese women, J. Nutr., 2010, 140, 580–586 CrossRef CAS PubMed.
- S. Amanat, M. H. Eftekhari, M. Fararouei, K. Bagheri Lankarani and S. J. Massoumi, Genistein supplementation improves insulin resistance and inflammatory state in non-alcoholic fatty liver patients: A randomized, controlled trial, Clin. Nutr., 2018, 37, 1210–1215 CrossRef CAS PubMed.
- H. Liu, H. Zhong, Y. Yin and Z. Jiang, Genistein has beneficial effects on hepatic steatosis in high fat-high sucrose diet-treated rats, Biomed. Pharmacother., 2017, 91, 964–969 CrossRef CAS PubMed.
- P. Lopez, M. Sanchez, C. Perez-Cruz, L. A. Velazquez-Villegas, T. Syeda, M. Aguilar-Lopez, A. K. Rocha-Viggiano, M. Del Carmen Silva-Lucero, I. Torre-Villalvazo, L. G. Noriega, N. Torres and A. R. Tovar, Long-term genistein consumption modifies gut microbiota, improving glucose metabolism, metabolic endotoxemia, and cognitive function in mice fed a high-fat diet, Mol. Nutr. Food Res., 2018, 62, e1800313 CrossRef PubMed.
- G. Huang, J. Xu, D. E. Lefever, T. C. Glenn, T. Nagy and T. L. Guo, Genistein prevention of hyperglycemia and improvement of glucose tolerance in adult non-obese diabetic mice are associated with alterations of gut microbiome and immune homeostasis, Toxicol. Appl. Pharmacol., 2017, 332, 138–148 CrossRef CAS PubMed.
- P. T. Koleva, J. S. Kim, J. A. Scott and A. L. Kozyrskyj, Microbial programming of health and disease starts during fetal life, Birth Defects Res., Part C, 2015, 105, 265–277 CrossRef CAS PubMed.
- L. Zhou and X. Xiao, The role of gut microbiota in the effects of maternal obesity during pregnancy on offspring metabolism, Biosci. Rep., 2018, 38 DOI:10.1042/BSR20171234.
- J. Zheng, X. Xiao, Q. Zhang, M. Yu, J. Xu and Z. Wang, Maternal high-fat diet modulates hepatic glucose, lipid homeostasis and gene expression in the PPAR pathway in the early life of offspring, Int. J. Mol. Sci., 2014, 15, 14967–14983 CrossRef CAS PubMed.
- L. Zhou, X. Xiao, Q. Zhang, J. Zheng, M. Li, M. Yu, X. Wang, M. Deng, X. Zhai, R. Li and J. Liu, Dietary genistein could modulate hypothalamic circadian entrainment, reduce body weight, and improve glucose and lipid metabolism in female mice, Int. J. Endocrinol., 2019, 2019, 1–10 Search PubMed.
- R. C. Edgar, UPARSE: highly accurate OTU sequences from microbial amplicon reads, Nat. Methods, 2013, 10, 996–998 CrossRef CAS PubMed.
- J. G. Caporaso, J. Kuczynski, J. Stombaugh, K. Bittinger, F. D. Bushman, E. K. Costello, N. Fierer, A. G. Pena, J. K. Goodrich, J. I. Gordon, G. A. Huttley, S. T. Kelley, D. Knights, J. E. Koenig, R. E. Ley, C. A. Lozupone, D. McDonald, B. D. Muegge, M. Pirrung, J. Reeder, J. R. Sevinsky, P. J. Turnbaugh, W. A. Walters, J. Widmann, T. Yatsunenko, J. Zaneveld and R. Knight, QIIME allows analysis of high-throughput community sequencing data, Nat. Methods, 2010, 7, 335–336 CrossRef CAS PubMed.
- T.
Z. DeSantis, P. Hugenholtz, N. Larsen, M. Rojas, E. L. Brodie, K. Keller, T. Huber, D. Dalevi, P. Hu and G. L. Andersen, Greengenes, a chimera-checked 16S rRNA gene database and workbench compatible with ARB, Appl. Environ. Microbiol., 2006, 72, 5069–5072 CrossRef CAS PubMed.
- Q. Wang, G. M. Garrity, J. M. Tiedje and J. R. Cole, Naive Bayesian classifier for rapid assignment of rRNA sequences into the new bacterial taxonomy, Appl. Environ. Microbiol., 2007, 73, 5261–5267 CrossRef CAS PubMed.
- S. C. Langley-Evans, Nutrition in early life and the programming of adult disease: a review, J. Hum. Nutr. Diet., 2015, 28(Suppl 1), 1–14 CrossRef PubMed.
- R. F. Boente, L. Q. Ferreira, L. S. Falcao, K. R. Miranda, P. L. Guimaraes, J. Santos-Filho, J. M. Vieira, D. E. Barroso, J. P. Emond, E. O. Ferreira, G. R. Paula and R. M. Domingues, Detection of resistance genes and susceptibility patterns in Bacteroides and Parabacteroides strains, Anaerobe, 2010, 16, 190–194 CrossRef CAS PubMed.
- A. Walker, B. Pfitzner, M. Harir, M. Schaubeck, J. Calasan, S. S. Heinzmann, D. Turaev, T. Rattei, D. Endesfelder, W. Z. Castell, D. Haller, M. Schmid, A. Hartmann and P. Schmitt-Kopplin, Sulfonolipids as novel metabolite markers of Alistipes and Odoribacter affected by high-fat diets, Sci. Rep., 2017, 7, 11047 CrossRef PubMed.
- C. Bressa, M. Bailen-Andrino, J. Perez-Santiago, R. Gonzalez-Soltero, M. Perez, M. G. Montalvo-Lominchar, J. L. Mate-Munoz, R. Dominguez, D. Moreno and M. Larrosa, Differences in gut microbiota profile between women with active lifestyle and sedentary women, PLoS One, 2017, 12, e0171352 CrossRef PubMed.
- A. B. Granado-Serrano, M. Martin-Gari, V. Sanchez, M. Riart Solans, R. Berdun, I. A. Ludwig, L. Rubio, E. Vilaprinyo, M. Portero-Otin and J. C. E. Serrano, Faecal bacterial and short-chain fatty acids signature in hypercholesterolemia, Sci. Rep., 2019, 9, 1772 CrossRef CAS PubMed.
- J. F. Garcia-Mazcorro, R. Pedreschi, B. Chew, S. E. Dowd, J. R. Kawas and G. Noratto, Dietary supplementation with raspberry extracts modifies the fecal microbiota in obese diabetic db/db mice, J. Microbiol. Biotechnol., 2018, 28, 1247–1259 CrossRef CAS PubMed.
- L. Wang, L. Hu, S. Yan, T. Jiang, S. Fang, G. Wang, J. Zhao, H. Zhang and W. Chen, Effects of different oligosaccharides at various dosages on the composition of gut microbiota and short-chain fatty acids in mice with constipation, Food Funct., 2017, 8, 1966–1978 RSC.
- B. R. Robertson, J. L. O'Rourke, B. A. Neilan, P. Vandamme, S. L. On, J. G. Fox and A. Lee,
Mucispirillum schaedleri gen. nov., sp. nov., a spiral-shaped bacterium colonizing the mucus layer of the gastrointestinal tract of laboratory rodents, Int. J. Syst. Evol. Microbiol., 2005, 55, 1199–1204 CrossRef CAS PubMed.
- D. Berry, C. Schwab, G. Milinovich, J. Reichert, K. Ben Mahfoudh, T. Decker, M. Engel, B. Hai, E. Hainzl, S. Heider, L. Kenner, M. Muller, I. Rauch, B. Strobl, M. Wagner, C. Schleper, T. Urich and A. Loy, Phylotype-level 16S rRNA analysis reveals new bacterial indicators of health state in acute murine colitis, ISME J., 2012, 6, 2091–2106 CrossRef CAS PubMed.
- D. Berry, O. Kuzyk, I. Rauch, S. Heider, C. Schwab, E. Hainzl, T. Decker, M. Muller, B. Strobl, C. Schleper, T. Urich, M. Wagner, L. Kenner and A. Loy, Intestinal microbiota signatures associated with inflammation history in mice experiencing recurring colitis, Front. Microbiol., 2015, 6, 1408 Search PubMed.
- S. El Aidy, M. Derrien, R. Aardema, G. Hooiveld, S. E. Richards, A. Dane, J. Dekker, R. Vreeken, F. Levenez, J. Dore, E. G. Zoetendal, P. van Baarlen and M. Kleerebezem, Transient inflammatory-like state and microbial dysbiosis are pivotal in establishment of mucosal homeostasis during colonisation of germ-free mice, Benefic. Microbes, 2014, 5, 67–77 CrossRef CAS PubMed.
- M. Xiao, X. Fu, Y. Ni, J. Chen, S. Jian, L. Wang, L. Li and G. Du, Protective effects of Paederia scandens extract on rheumatoid arthritis mouse model by modulating gut microbiota, J. Ehnopharmacol., 2018, 226, 97–104 CrossRef CAS PubMed.
- J. Lee, J. Y. Jang, M. S. Kwon, S. K. Lim, N. Kim, J. Lee, H. K. Park, M. Yun, M. Y. Shin, H. E. Jo, Y. J. Oh, B. H. Ryu, M. Y. Ko, W. Joo and H. J. Choi, Mixture of two Lactobacillus Plantarum strains modulates the gut microbiota structure and regulatory T cell response in diet-induced obese mice, Mol. Nutr. Food Res., 2018, 62, e1800329 CrossRef PubMed.
- X. Zhai, D. Lin, Y. Zhao, W. Li and X. Yang, Effects of dietary fiber supplementation on fatty acid metabolism and intestinal microbiota diversity in C57BL/6J mice fed with a high-fat diet, J. Agric. Food Chem., 2018, 66, 12706–12718 CrossRef CAS PubMed.
- K. Li, L. Zhang, J. Xue, X. Yang, X. Dong, L. Sha, H. Lei, X. Zhang, L. Zhu, Z. Wang, X. Li, H. Wang, P. Liu, Y. Dong and L. He, Dietary inulin alleviates diverse stages of type 2 diabetes mellitus via anti-inflammation and modulating gut microbiota in db/db mice, Food Funct., 2019, 10, 1915–1927 RSC.
- A. Everard, V. Lazarevic, N. Gaia, M. Johansson, M. Stahlman, F. Backhed, N. M. Delzenne, J. Schrenzel, P. Francois and P. D. Cani, Microbiome of prebiotic-treated mice reveals novel targets involved in host response during obesity, ISME J., 2014, 8, 2116–2130 CrossRef CAS PubMed.
- X. Zhang, Y. Zhao, M. Zhang, X. Pang, J. Xu, C. Kang, M. Li, C. Zhang, Z. Zhang, Y. Zhang, X. Li, G. Ning and L. Zhao, Structural changes of gut microbiota during berberine-mediated prevention of obesity and insulin resistance in high-fat diet-fed rats, PLoS One, 2012, 7, e42529 CrossRef CAS PubMed.
- X. Zhang, Y. Zhao, J. Xu, Z. Xue, M. Zhang, X. Pang, X. Zhang and L. Zhao, Modulation of gut microbiota by berberine and metformin during the treatment of high-fat diet-induced obesity in rats, Sci. Rep., 2015, 5, 14405 CrossRef CAS PubMed.
- P. Louis and H. J. Flint, Diversity, metabolism and microbial ecology of butyrate-producing bacteria from the human large intestine, FEMS Microbiol. Lett., 2009, 294, 1–8 CrossRef CAS PubMed.
- . P. Van den Abbeele, C. Belzer, M. Goossens, M. Kleerebezem, W. M. De Vos, O. Thas, R. De Weirdt, F. M. Kerckhof and T. Van de Wiele, Butyrate-producing Clostridium cluster XIVa species specifically colonize mucins in an in vitro gut model, ISME J., 2013, 7, 949–961 CrossRef PubMed.
- M. Remely, E. Aumueller, C. Merold, S. Dworzak, B. Hippe, J. Zanner, A. Pointner, H. Brath and A. G. Haslberger, Effects of short chain fatty acid producing bacteria on epigenetic regulation of FFAR3 in type 2 diabetes and obesity, Gene, 2014, 537, 85–92 CrossRef CAS PubMed.
- M. Sasaki, N. Ogasawara, Y. Funaki, M. Mizuno, A. Iida, C. Goto, S. Koikeda, K. Kasugai and T. Joh, Transglucosidase improves the gut microbiota profile of type 2 diabetes mellitus patients: a randomized double-blind, placebo-controlled study, BMC Gastroenterol., 2013, 13, 81 CrossRef CAS PubMed.
- U. Etxeberria, N. Arias, N. Boque, M. T. Macarulla, M. P. Portillo, F. I. Milagro and J. A. Martinez, Shifts in microbiota species and fermentation products in a dietary model enriched in fat and sucrose, Benefic. Microbes, 2015, 6, 97–111 CrossRef CAS PubMed.
- M. Remely, I. Tesar, B. Hippe, S. Gnauer, P. Rust and A. G. Haslberger, Gut microbiota composition correlates with changes in body fat content due to weight loss, Benefic. Microbes, 2015, 6, 431–439 CrossRef CAS PubMed.
- Y. S. Kuang, J. H. Lu, S. H. Li, J. H. Li, M. Y. Yuan, J. R. He, N. N. Chen, W. Q. Xiao, S. Y. Shen, L. Qiu, Y. F. Wu, C. Y. Hu, Y. Y. Wu, W. D. Li, Q. Z. Chen, H. W. Deng, C. J. Papasian, H. M. Xia and X. Qiu, Connections between the human gut microbiome and gestational diabetes mellitus, GigaScience, 2017, 6, 1–12 CrossRef PubMed.
- X. Si, W. Shang, Z. Zhou, P. Strappe, B. Wang, A. Bird and C. Blanchard, Gut microbiome-induced shift of acetate to butyrate positively manages dysbiosis in high fat diet, Mol. Nutr. Food Res., 2018, 62 DOI:10.1002/mnfr.201700670.
- X. Wei, J. Tao, S. Xiao, S. Jiang, E. Shang, Z. Zhu, D. Qian and J. Duan, Xiexin Tang improves the symptom of type 2 diabetic rats by modulation of the gut microbiota, Sci. Rep., 2018, 8, 3685 CrossRef PubMed.
- S. Perez-Burillo, S. Pastoriza, A. Fernandez-Arteaga, G. Luzon, N. Jimenez-Hernandez, G. D'Auria, M. P. Francino and J. A. Rufian-Henares, Spent coffee grounds extract, rich in mannooligosaccharides, promotes a healthier gut microbial community in a dose-dependent manner, J. Agric. Food Chem., 2019, 67, 2500–2509 CrossRef CAS PubMed.
- F. F. Anhe, R. T. Nachbar, T. V. Varin, V. Vilela, S. Dudonne, G. Pilon, M. Fournier, M. A. Lecours, Y. Desjardins, D. Roy, E. Levy and A. Marette, A polyphenol-rich cranberry extract reverses insulin resistance and hepatic steatosis independently of body weight loss, Mol. Metab., 2017, 6, 1563–1573 CrossRef CAS PubMed.
- L. Li, W. L. Guo, W. Zhang, J. X. Xu, M. Qian, W. D. Bai, Y. Y. Zhang, P. F. Rao, L. Ni and X. C. Lv,
Grifola frondosa polysaccharides ameliorate lipid metabolic disorders and gut microbiota dysbiosis in high-fat diet fed rats, Food Funct., 2019, 10, 2560–2572 RSC.
- T. T. Li, Y. Y. Liu, X. Z. Wan, Z. R. Huang, B. Liu and C. Zhao, Regulatory efficacy of the polyunsaturated fatty acids from microalgae spirulina platensis on lipid metabolism and gut microbiota in high-fat diet rats, Int. J. Mol. Sci., 2018, 19, 3075 CrossRef PubMed.
- S. M. Henning, J. Yang, M. Hsu, R. P. Lee, E. M. Grojean, A. Ly, C. H. Tseng, D. Heber and Z. Li, Decaffeinated green and black tea polyphenols decrease weight gain and alter microbiome populations and function in diet-induced obese mice, Eur. J. Nutr., 2018, 57, 2759–2769 CrossRef CAS PubMed.
- C. Ye, L. Liu, X. Ma, H. Tong, J. Gao, Y. Tai, L. Huang, C. Tang and R. Wang, Obesity Aggravates Acute Pancreatitis via Damaging Intestinal Mucosal Barrier and Changing Microbiota Composition in Rats, Sci. Rep., 2019, 9, 69 CrossRef PubMed.
- M. Li, Y. Wu, Y. Hu, L. Zhao and C. Zhang, Initial gut microbiota structure affects sensitivity to DSS-induced colitis in a mouse model, Sci. China: Life Sci., 2018, 61, 762–769 CrossRef PubMed.
- S. M. McNabney and T. M. Henagan, Short chain fatty acids in the colon and peripheral tissues: a focus on butyrate, colon cancer, obesity and insulin resistance, Nutrients, 2017, 9, 1348 CrossRef PubMed.
- G. den Besten, K. van Eunen, A. K. Groen, K. Venema, D. J. Reijngoud and B. M. Bakker, The role of short-chain fatty acids in the interplay between diet, gut microbiota, and host energy metabolism, J. Lipid Res., 2013, 54, 2325–2340 CrossRef CAS PubMed.
- S. Louis, R. M. Tappu, A. Damms-Machado, D. H. Huson and S. C. Bischoff, Characterization of the gut microbial community of obese patients following a weight-loss intervention using whole metagenome shotgun sequencing, PLoS One, 2016, 11, e0149564 CrossRef PubMed.
- Y. Kang, Y. Li, Y. Du, L. Guo, M. Chen, X. Huang, F. Yang, J. Hong and X. Kong, Konjaku flour reduces obesity in mice by modulating the composition of the gut microbiota, Int. J. Obes., 2018 DOI:10.1038/s41366-018-0187-x.
- A. Riviere, M. Selak, D. Lantin, F. Leroy and L. De Vuyst,
Bifidobacteria and butyrate-producing colon bacteria: importance and strategies for their stimulation in the human gut, Front. Microbiol., 2016, 7, 979 Search PubMed.
Footnote |
† Electronic supplementary information (ESI) available. See DOI: 10.1039/c9fo01046g |
|
This journal is © The Royal Society of Chemistry 2019 |