Overcoming ammonia inhibition in anaerobic blackwater treatment with granular activated carbon: the role of electroactive microorganisms
Received
29th August 2018
, Accepted 30th October 2018
First published on 9th January 2019
Abstract
Methanogenesis and enrichment of microorganisms capable of interspecies electron and/or hydrogen exchange was investigated with addition of granular activated carbon (GAC) to batch anaerobic digesters treating vacuum collected blackwater with high ammonia concentration. The biochemical methane potential increased from 34% to 53%, while chemical oxygen demand removal efficiencies increased from 37% up to 56.7%. GAC did not influence the concentration of total ammonia but reduced its inhibition. The methanogenesis lag phase did not differ between groups. In 10 days, increases of up to 48% of acetate and up to 52% of propionate were observed in GAC cultures. After 45 days, propionate was depleted in all cultures, except the GAC 4 g culture. Acetate accumulated in control groups, reaching approximately 126 mg L−1 after 21 days. GAC promoted cell attachment and biofilm formation on its surface, while polyethylene did not. 16S rRNA gene sequencing revealed enhancement of conductive bacterial OTUs in the GAC group, including the Geobacteraceae family (4.0%) and the Clostridiales order (14%), while the versatile archaeal group Methanosarcina (∼63%) was preserved, and unclassified hydrogenotroph members of the Methanomicrobiales order increased by 14%. Results suggest that activated carbon improves methane production from blackwater degradation by enriching electroactive microorganisms tolerant to high ammonia concentrations.
Water impact
This study shows that GAC improves methanogenesis from anaerobic degradation of blackwater by promoting direct interspecies electron transfer, in the presence of high concentration of free ammonia. GAC is an effective strategy to overcome ammonia inhibition in this process. It improves methanogenesis in a sustainable way, and thus, it is a cost-effective strategy for converting waste to biogas in large scale.
|
Introduction
Uniquely comprising waste from toilets (urine and faecal matter), blackwater contains around half of the load of domestic chemical oxygen demand (COD) and more than 90% nitrogen and phosphorous.1,2 Due to its specific composition, source-diverted blackwater collection improves organic compound energy recovery and nutrient recovery. For wastewater highly rich in organic matter, anaerobic digestion has been applied as the key treatment technology.3–5 The process is robust, coping with high organic loading rates and generating low levels of sludge. Besides its efficiency, it has less operational complexity and lower energetic costs compared to other traditional wastewater treatment methods.3 While anaerobically breaking down organic matter, this process yields an effluent with low organic content, biogas in a methane
:
carbon dioxide ratio of 55–70
:
30–45 (v:v), and trace concentrations of hydrogen sulfide (H2S), hydrogen, and water vapor.3
Conventionally, in anaerobic degradation, complex organic matter is hydrolyzed to monomeric organic materials6,7 that are afterward fermented by acidogenic bacteria generating acetate and small organic molecules such as lactate, succinate, and fatty acids; syntrophic bacteria convert such molecules to acetate.6–9 The electrons generated in both processes are used to form hydrogen and formate.6,7,9 Methanogenic archaea, in turn, convert acetate into methane and carbon dioxide, or utilize the electrons from hydrogen and formate to reduce carbon dioxide to methane.6,9
Anaerobic digestion of blackwater is influenced by the organic matter and nutrient concentration in blackwater, which vary significantly based on the amount of water used for toilet flushing. Low dilution blackwater generated from low-flush or vacuum toilets has been shown to contain very high organic matter and nutrient loads, which can affect the speed of hydrolysis, accumulating suspended solids and decreasing methanogenic activity in the system.4,5,10 Free NH3 molecules and the ion NH4+ are the two most significant methanogenesis inhibitors when present in high concentrations in anaerobic digestion processes.11,12 Inhibition likely occurs due to a proton imbalance and/or potassium deficiency induced by diffusion of NH3 into the cells.11 When ammonium, under pH interference, interacts with acids in the medium, the system might deteriorate or run stably but with a low methane yield.5
Microorganisms able to syntrophically oxidize acetate have been found in anaerobic digestion systems, especially under high ammonia concentrations,11,13 which suggests their importance in overcoming ammonia inhibition issues by providing hydrogen for methanogenic archaea. Although a clear relationship between NH3 concentration and methanogenic pathways has not been reported, acetoclastic methanogens are considered to be less tolerant than hydrogenotrophic methanogens.5 Therefore, the shift of predominant pathway under high NH3 concentration seems to be triggered by the activity of acetate-oxidizing syntrophs.14
The syntrophic relationship between bacteria and hydrogenotrophic methanogenic archaea is a widely publicized process11,13,15,16 in which hydrogen is transferred interspecies. The concentration of hydrogen in the aqueous phase of the cultures directly impacts the activity of syntrophic bacteria. Under high organic load rates, when the activity of methanogens is impacted, and the consumption of hydrogen is relatively low, the thermodynamics of interspecies hydrogen transfer gets inhibited, which can contribute to the accumulation of volatile fatty acids (VFAs), drop in pH and failure of the anaerobic digestion process.17,18 Some studies have shown that a direct transfer of electrons between syntrophic bacteria and methanogenic archaea is an alternative to hydrogen transfer during syntrophic metabolism. This direct interspecies electron transfer (DIET) is reported to happen via biological electrical connections, such as electrically conductive pili and outer surface c-type cytochromes. Although studies performed on extracellular pili-like structures of Shewanella oneidensis and Pseudomonas aeruginosa indicated those structures to be non-conductive, in the most studied members of Geobacter species,19 the combination of conductive pili and c-cytochromes seem to be responsible for the direct interspecies electron transfer. Conductive materials have been added to digesters to improve the efficiency of anaerobic digestion, accelerating and stabilizing the conversion of organic substrates to methane by promoting DIET.19–21 Most studies, however, focus on the degradation of simple substrates such as ethanol, propionate, and butyrate9,20,22 and are performed with defined co-cultures or within bioreactors fed pre-treated wastewater or semi-solid slurry.9,23 The ability of activated carbon to enhance anaerobic digestion of blackwater in the presence of high ammonia concentration has not yet been investigated.
This study evaluated methanogenic activity in anaerobic batch cultures treating vacuum toilet collected blackwater (1 L water per flush configuration), with and without the supplementation of granular activated carbon (GAC). Moreover, the effect of different concentrations of the conductive material on anaerobic digestion was correlated to the VFA profile, COD, conductivity measurements of microbial cells, and shifts within archaeal and bacterial communities detected by 16S rRNA gene sequencing.
Material and methods
Blackwater preparation and inoculum sludge
Blackwater (urine and feces) with no flush water was collected over a one week period from 10 individuals, as previously described by Gao et al. (2018),10 then mixed and stored anaerobically at 4 °C. Vacuum collected blackwater used in this study was prepared by diluting collected blackwater using demineralized water to reach a 1 L water-flush configuration. The inoculum was anaerobic sludge obtained from a conventional anaerobic digester treating waste activated sludge from a wastewater treatment plant in Alberta.
Experimental set-up
An aliquot (200 μL) of anaerobic sludge was added as inoculum to 157 mL serum bottles with 60 mL of blackwater. The small inoculum addition aimed to reduce methane production contributed by the organic matter present in the inoculum solution. Serum bottles were sealed with butyl rubber stoppers (Bellco Glass, Vineland, NJ) and flushed with N2 in the headspace. Enrichments were incubated in the dark at 35 °C on a shaking incubator at 120 rpm for 65 days. GAC with 4–12 mesh particle-size (Sigma-Aldrich, St. Louis, Missouri) was used as conductive material. Six sets of triplicate anaerobic bottles were configured: a control group consisting of blackwater + inoculum; 4 groups consisting of blackwater + inoculum + GAC (0.5, 1.0, 2.0, and 4.0 g), and one group consisting of blackwater + inoculum + 2.0 g of inert polyethylene (PE) (Rauschert, Steinwiessen, Germany) with the same particle size as the activated carbon. GAC particles were immersed in demineralized water overnight, then washed thoroughly prior to addition to the bottles to avoid carbon powder being washed out and suspended in the cultures.
Analytical methods
The methane composition of the biogas in the headspace of the batch bottles was tracked using a gas chromatograph GC-7890B (Agilent Technologies, Santa Clara, CA) equipped with a G3591-81023, Hayesep Q column and a thermal conductivity detector. Temperatures of the oven and detector were set to 100 and 200 °C, respectively. Helium (99.999%) was used as carrier gas. Acetate, propionate, and butyrate concentrations were quantified using a Dionex ICS-2100 ion chromatograph equipped with an IonPac AS18 column and 4.5 mM carbonate/1.4 mM bicarbonate eluent at a flow rate of 0.25 ml min− 1 (Dionex, Sunnyvale, CA). Culture pH was measured immediately after sampling using a symphony pH probe (VWR, Radnor, PA). COD was determined according to the standard methods using the closed reflux titrimetric method 5220C. The biochemical methane potential (BMP) was calculated as a percentage of the influent COD converted to methane. Total ammonia nitrogen (NH3–N + NH4+–N, TAN) was determined via the Nessler method (HACH, Loveland, CO), and the concentration of free ammonia (NH3) was calculated as previously described13 using the following equation, where T stands for temperature (K): | 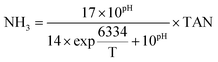 | (1) |
Conductivity measurements
Biofilms of the control and GAC-amended cultures showing the best methanogenic performance were analyzed for conductivity. Biomass in suspension was harvested by centrifugation at 800 × g for 5 minutes, while biomass attached to GAC was detached by sonication in a FS30H water bath (Fisher Scientific, Hampton, NH) for 15 minutes. Each type of biomass was placed on a gold spiral electrode (A-AD-GG-103-N Bare Gold Electrode, Zimmer and Peacock, Horten, Norway) and a Keithley 2400 SourceMeter (Keithley, Cleveland, OH) was used to apply a voltage ramp from 0.0 V to 0.3 V at intervals of 0.1 V. The measurements were recorded by LabTracer 2.0 (Keithley, Cleveland, OH) software.
The conductivity (σ, S m−1) of the biomass was calculated as described in Zhao et al. (2016)24 using the following equation:
|  | (2) |
where
ρ is the resistivity of the biomass (
Ω m), which was calculated by the following equation:
|  | (3) |
where
R is the reciprocal of the average slope of the current–voltage curve (
Ω),
S is the side area of the spiral conductive print (2.09 × 10
−2 m in length and 13.50 × 10
−9 m in thickness, m
2) and
L is the width of the gap between working and counter electrodes (3.00 × 10
−4 m).
All voltage values reported in our biofilm conductivity experiments are potential difference, not absolute potential. Hence, the reference electrode was combined with the working electrode into a single electrode which was then connected to ground (i.e. a zero potential point).
Scanning electron microscopy (SEM) analysis
A 3% (v/v) solution of glutaraldehyde in phosphate buffered saline was used to fix the cells at 4 °C; afterward, samples were dehydrated in increasing concentrations of ethanol and ethanol
:
hexamethyldisilazane and air-dried. Cells were analysed using a Zeiss Sigma 300 VP-FESEM microscope (Zeiss, Oberkochen, Germany).
DNA extraction
Control group and GAC-amended cultures showing the best methanogenic performance (i.e. highest BMP) were selected to have their microbial community investigated. Biomass in suspension was harvested by centrifuging samples at 10
000 × g for 5 minutes. Biomass attached to the GAC was detached by vigorous vortexing for 5 minutes then centrifuging at 10
000 × g for 5 min. The harvested suspended biomass from the control group and the combination of suspended and attached biomass from the GAC groups were used for DNA extraction with the DNeasy PowerSoil Kit (QIAGEN, Hilden, Germany), according to the manufacturer's protocol. Purity and concentration of the extracted DNA were analyzed using a NanoDrop One (ThermoFisher, Walthan, MA). Concentrations of DNA were approximately 50 ng μL−1, with 260/280 ratios of approximately 1.8. Extracted DNA samples were stored at −20 °C until sequencing. Kit blanks were performed and resulted in no amplification when universal primers for bacteria and archaea were used.
Sequencing and microbial community analysis
Frozen DNA samples were dispatched to RTL Genomics (Texas, USA), where PCR was performed followed by sequencing on the Illumina MiSeq platform. Bacterial 16S rRNA genes were amplified using bacterial universal primers, 357wF (CCTACGGGNGGCWGCAG) and 785R (GACTACHVGGGTATCTAATCC). Similarly, archaeal 16S rRNA genes were amplified by the universal primer set for archaea: 517F (GCYTAAAGSRNCCGTAGC) and 909R (TTTCAGYCTTGCGRCCGTAC). The 16S Illumina paired-end data of both forward and reverse reads were merged into a single sequence. The output file, the barcode file, and its respective mapping file were then processed and sorted using the default parameters on the quantitative insights into microbial ecology pipeline – QIIME version 1.9.1. Quality filtered reads were assigned to operational taxonomic units (OTUs)25 at 97% similarity and blasted against the reference SILVA database project (SILVA_128_release).26 Reads that did not match the reference database were subsequently clustered using a de novo approach. Furthermore, diversity analyses were performed by running a workflow on QIIME, which analyzed alpha-diversity, beta-diversity, and composition analysis scripts of the samples.
Results and discussion
Effect of GAC on blackwater anaerobic digestion
Levels of total and free ammonia remained constant throughout all incubations, around 2800 ± 200 mg L−1 and 281 ± 20 mg L−1, respectively. After 57 days of anaerobic digestion, the accumulative methane production reached on average 133, 145, 166, 209, and 204 mmol L−1 under a GAC dosage of 0, 0.5, 1, 2, and 4 g, respectively (Fig. 1). When inert material was added to the culture, methane production yielded concentrations comparable to the control groups (138 ± 9 mmol L−1, P = 0.18). The lag period for methane production did not differ among the groups (∼8 days), and after only 21 days of digestion the GAC-amended cultures started showing considerably higher methane production. Although distinct additions of GAC did not affect the kinetics of methanogenesis, they clearly improved methane production, mitigating the inhibition of this step by the presence of constantly high free-ammonia levels. Activated carbon has been widely recognized as a suitable adsorbent material for removing and preventing odor or fumes, but due to its nonpolar surface, in general it does not have enough adsorption capacity for the polar ammonia.26,27 Few studies have been performed to alleviate ammonia inhibition by the addition of activated carbon. Hansen et al. (1999)27 reported that the addition of 1.5% (w/w) of activated carbon to cultures in the presence of total ammonia in initial concentrations of 6 g L−1 increased methane production from 67 up to 126 mL CH4 g VS−1, which was reported to occur due to adsorption of ammonia by the conductive material. The study of Poirier et al. (2017),28 in turn, revealed that the addition of 10 g L−1 of activated carbon to batch digesters decreased the lag phase of the cultures by 10 days, while methane yields were comparable to those achieved by control groups, indicating that activated carbon improved the kinetics of methanogenesis due to its conductive property, rather than to its adsorption of ammonia. In this study, GAC most likely improved methane production by playing a conductive role instead of adsorbing ammonia, as the levels of ammonia did not undergo changes during the experiments. Moreover, the similar levels of methane obtained in control and inert material groups, and those previously obtained by Gao et al. (2018)10 in ammonia-inhibited cultures, reinforce the conclusion that conductivity is involved in the methanogenic activity observed in GAC-amended cultures.
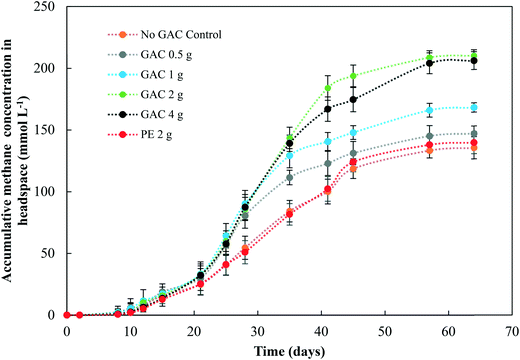 |
| Fig. 1 Accumulative methane concentration in the headspace during anaerobic digestion of blackwater. Measurements of biological triplicates were averaged, and the standard deviation is represented by the error bar. GAC – granular activated carbon; PE – polyethylene. | |
Initially, a COD of ∼20 g L−1 was present in the cultures. After 65 days of anaerobic digestion, the total COD concentration decreased by 37.0, 38.5, 42.5, 47.5, and 50.5% in cultures receiving 0, 0.5, 1, 2, and 4 g of granular activated carbon, respectively (Fig. 2). Total COD removal efficiency in the group receiving 2 g of inert material reached 38.2%, similar to that of the no-GAC control group. The organics biodegradation observed was proportional to the yields of methane in the cultures, suggesting that the organic matter was mostly converted into methane and incorporated as microbial biomass. Compared to the initial COD concentration, the BMP of the cultures increased from 34.2% in the no-GAC control group to 36.1, 41.3, 52.8, and 51.7% when 0.5, 1, 2, and 4 g of GAC, respectively, were added. The BMP of the inert material group cultures reached 34.3%. Comparable values obtained from the inert material and the control groups indicate that the enhanced methane production with GAC materials cannot be attributed to the presence of biofilm support media (GAC or PE) for the microorganisms, but material conductivity played an important role in methanogenic activity. Besides, the addition of 2 g GAC per 60 mL culture (∼33 g L−1) seemed to be the optimal concentration, above which, the methanogenesis efficiency was shown to slightly decrease (P = 0.008), with a significant difference in the COD removal efficiency (P = 0.002). Liu et al. (2012)20 and Yan et al. (2017)29 also reported high GAC loadings (25 and 10 g GAC L−1, respectively) to achieve optimal concentrations of activated carbon, yielding higher methane production from anaerobic digesters. Yang et al. (2017),30 however, reported 5 g GAC L−1 as the ideal concentration of GAC to achieve the best methanogenic performance in cultures performing anaerobic sludge digestion. Therefore, the optimum GAC concentration and its specific surface area have yet to be established.
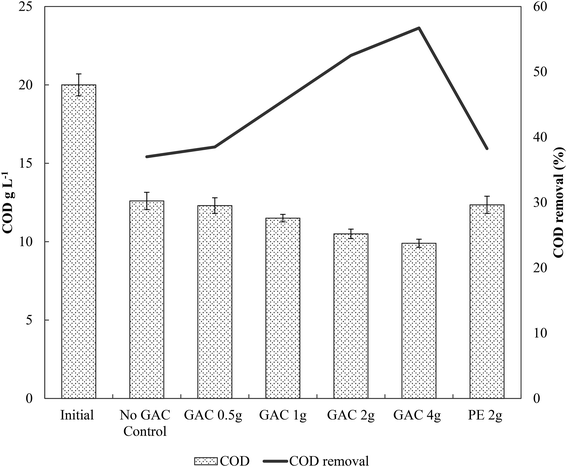 |
| Fig. 2 Chemical oxygen demand (COD) and COD removal efficiency in the cultures. Measurements of biological triplicates were averaged and the standard deviation is represented by the error bar. GAC – granular activated carbon; PE – polyethylene. | |
Gao et al. (2018),10 using a similar blackwater source for anaerobic degradation of diluted blackwater, reported methanogenesis efficiency comparable to the results obtained by the no-GAC control group in the present study. The authors investigated the relatively low BMP in such condition and reported free ammonia to be the main cause of inhibition in the process. The addition of activated carbon in this study increased the methanogenic efficiency of the cultures by approximately 18.6%, resulting in BMP values similar to those obtained by Gao et al. (2018)10 for degradation of blackwater in 9 L-dilution condition, when ammonia inhibition did not play a significant role.
Volatile fatty acids dynamics
The pH of the analyzed cultures ranged from 7.7 to 8.1 throughout the experiment. The VFAs, acetate, propionate, and butyrate peaked in the first 8 days of incubation in all the cultures (Fig. 3), and dropped after that, coinciding with the beginning of methanogenic activity (Fig. 1). Acetate reached concentrations of 1277, 1234, 1312, 1578, and 1788 mg L−1 when GAC was added in 0, 0.5, 1, 2, and 4 g quantities, respectively. In cultures receiving inert material, acetate reached 1174 mg L−1. This VFA was depleted in cultures receiving 0.5 g and 1 g GAC after 46 days of incubation, while for cultures receiving 2 g and 4 g GAC it was completely depleted only at day 64. In the control group and the group receiving inert material, however, 139 and 148 mg L−1 acetate accumulated in the cultures. Propionate reached concentrations of 500, 558, 582, 692, and 643 mg L−1 in cultures receiving 0, 0.5, 1, 2, and 4 g GAC, respectively. Such proprionate concentrations were depleted in all the cultures within 46 days of incubation, except for the group receiving 4 g GAC, in which 466 mg L−1 proprionate remained in the cultures. Butyrate was not produced in any of the cultures, varying from 131 to 254 mg L−1 and completely consumed within 25 to 36 days of incubation.
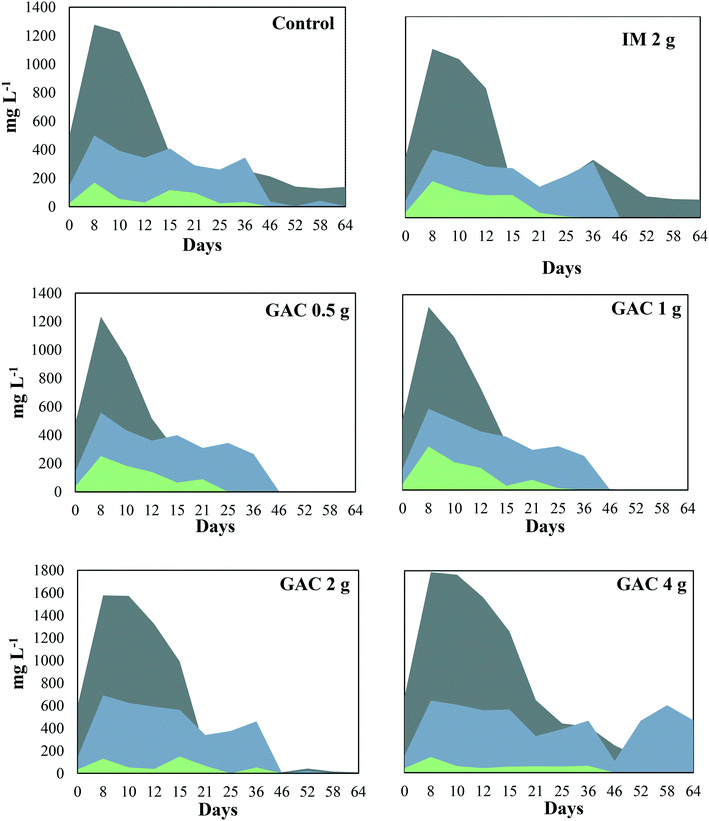 |
| Fig. 3 Volatile fatty acid profile during anaerobic digestion of blackwater with and without the addition of granular activated carbon. = acetate; = propionate; = butyrate. | |
Although acetate reached up to 1788 mg L−1, the anaerobic digestion did not show instability in methanogenic activity. Overall, the addition of GAC led to a higher production of acetate in the cultures, in comparison to control groups. The results obtained in this study are in line with those obtained by Lozecznik et al. (2010),31 in which at low concentrations of propionate (<178 mg L−1), methanogenesis of leachate was greater at high (2137 mg L−1) rather than low (510 mg L−1) acetate concentrations. However, higher VFA concentrations have been reported to hinder methanogenesis, and the most common explanation for process failure is acidification due to the concentration of undissociated fatty acids, particularly propionic acid.32 Lozecznik et al. (2010)31 suggested that the ratio between acetate and propionate has an important influence on methanogenic efficiency during anaerobic digestion. The authors reported that the greatest methanogenic activity from landfill leachate was achieved when concentrations of acetate and propionate initially ranged from 1500–1900 and from 1000–1800 mg L−1, respectively. The lower production of acetate and its accumulation in the control groups indicated for the GAC-amended cultures that GAC affected the hydrolysis and/or the acidification steps of anaerobic digestion. It likely improved the substrate availability, allowing formation of higher concentrations of VFAs and hydrogen, and therefore provided more substrates for methanogenic microorganisms.
Oxidation of the VFAs propionate and butyrate is inhibited by H2 partial pressures, therefore it can only occur when H2 partial pressures decrease to an extremely low level (<10−4 atm).33 In this study, the dynamics of propionate did not seem to be significantly influenced by the addition of GAC, except for the GAC 4 g condition in which VFA accumulated to approximately 465 mg L−1. The buildup of propionate suggests that its conversion to acetate might have been hampered, likely due to an increased partial pressure of hydrogen, as the VFA levels were much higher than those observed in the control groups. Moreover, methane production from the GAC 4 g group did not significantly differ from that of the GAC 2 g group, which could also indicate that the accumulation of propionate might be responsible for the decrease in methanogenesis efficiency, as the buildup of propionate overcame the concentration of acetate after 46 days.
Conductivity measurements
In the stationary phase, samples were taken from the control groups and from the GAC 2 g group, as it showed the best methanogenic performance, for conductivity measurements of the suspended biomass and for the biomass attached to GAC (Fig. 4). The electrical conductivity of the suspended biomass in the control group was 1.33 μS cm−1, while in the GAC 2 g group it reached 4.87 μS cm−1 in suspension and 8.08 μS cm−1 in the biomass attached to GAC. As reported in previous studies, conductive materials affect anaerobic digestion by establishing direct electron transfer between syntrophic acetate-oxidizing bacteria and hydrogenotrophic methanogenic archaea.19–21,30,34 In this study, GAC stimulated methanogenesis, as it was improved by a 18.6% increase in BMP compared to the control groups. The increase in conductivity and the improvement in methane production reinforce the idea that activated carbon stimulated syntrophic metabolism in the system.
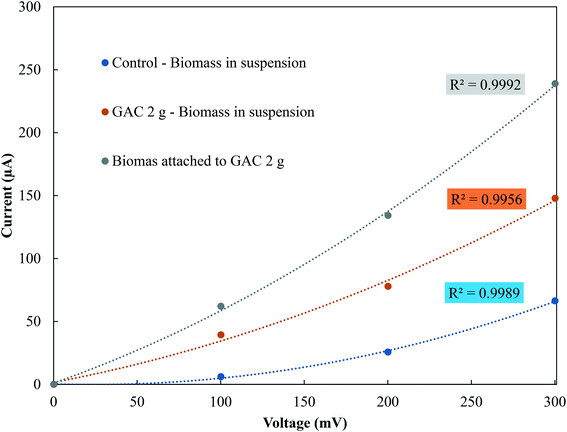 |
| Fig. 4 Current–voltage response of suspended and attached biomass in control and GAC 2 g groups in the stationary phase. | |
SEM analysis
Fig. 5 shows scanning electron micrographs retrieved from the no-GAC control and from the GAC surface in GAC-embedded cultures. The presence of cells tightly bound to the surface of the conductive material underlines the role of GAC in transferring electrons between groups during anaerobic digestion of blackwater.
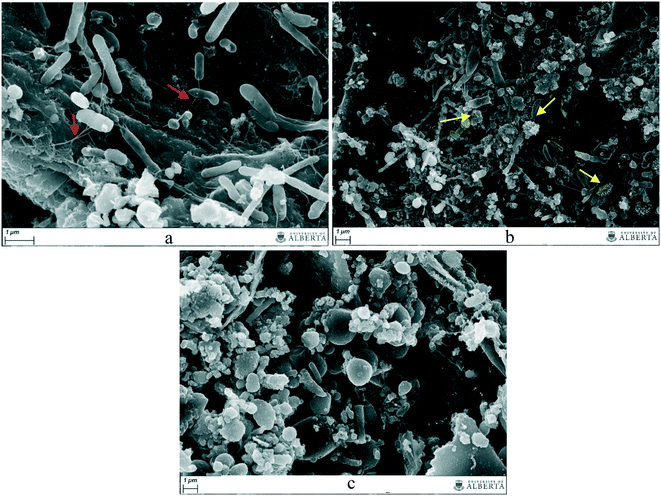 |
| Fig. 5 Scanning electron micrographs. (a) GAC surface. Red arrows point to pili-like structures likely from Geobacter species, highly enriched in the GAC embedded cultures. (b) Biomass in suspension in GAC-embedded cultures. Yellow arrows point to a group of conductive bacteria exclusively enriched in GAC-embedded cultures. (c) No-GAC control culture. Scale is represented below each photograph. | |
The addition of GAC to the cultures led to an enrichment of more conductive organisms, as confirmed by 16S rRNA sequence analysis. Pili-like structures can also be observed in such cultures, although further investigation is required to confirm the nature of the connections between cells and between cells and GAC (Fig. 5a). Moreover, the suspended biomass in the GAC-amended cultures disclosed the presence of Chlorobiales-like cells (Fig. 5b), similar to those reported by Lovley (2017),19 growing on the GAC surface and exchanging electrons with Geobacter sulfurreducens, as evidence of the direct interspecies electron transfer (DIET) hypothesized by the author. Analysis of the suspended biomass in no-GAC control cultures did not reveal a noticeable presence of pili-like structures connecting the cells (Fig. 5c), or any other structure indicative of conductivity. Therefore, the increased production of methane in GAC-embedded culture is likely due to an enhanced conductivity generated by the addition of activated carbon. Previous SEM studies have reported that in the presence of conductive materials, electroactive microorganisms prefer to electrically connect to the carbon surface rather than to other cells, likely to improve electron conduction.20,35Geobacter, Methanosaeta, and Methanothermobacter species are the groups mostly studied in co-cultures for methane production improvement by GAC addition. For those groups, pili-like structures were described as one of the main components in DIET,19 and SEM images, similarly to this study, corroborated the importance of such structures for the connection of cells to the conductive material.
Although biological connections between microorganisms might be established without the need of GAC, it is likely that activated carbon improves the syntrophic metabolism by providing higher conductivity between cells than would be generated by biological electrical connections. Due to its large size and insolubility, GAC is prevented from penetrating the cell surface, therefore, GAC-mediated electron exchange between species takes place outside the cell, similarly to DIET.19,21 Furthermore, the surface hydrophobicity and the porous character of conductive materials promote a suitable support for microorganism attachment, as reported by Sasaki et al. (2010).36 The close proximity between syntrophic bacteria and methanogenic archaea associated with biofilms on the GAC surface reduces diffusion constraints and assists direct or indirect interspecies electron exchange. Moreover, methanogenic archaea are retained in the pores of the conductive material, and thus are protected from potential inhibitory compounds.37 Studies have shown that hydrogen can also adsorb to carbon materials,38 which might imply their ability to adsorb hydrogen gas generated by fermentative bacteria and syntrophic acetate oxidizers in the anaerobic digester, allowing hydrogenotrophic methanogens present on the conductive surface to directly utilize it.
Microbial community profiles
To explore the microbiology behind the enhanced methanogenesis in GAC-amended cultures, bacterial and archaeal communities from the inoculum, collected blackwater, the control group, and the methanogenic GAC group with best performance were assessed. Analysis of the 16S rRNA gene amplicons of the inoculum yielded 54
476 high quality sequences, 67.5% of which belonged to bacteria, 32.27% to archaea, and 0.23% that could not be assigned to a category. Blackwater analysis resulted in 33
737 sequences, with 99.9% assigned to bacteria and 0.1% that could not be identified. Control and GAC groups yielded, respectively, 47
635 and 51
188 sequences, from which 66.3 and 62.2% belonged to bacteria and 33.6 and 37.8% to archaea. All the sequences from GAC groups could be assigned to a taxonomic unit, and 0.1% of the sequences obtained from the control group could not be assigned to any OTU.
Bacterial community.
The three most representative bacterial phyla in all cultures were Firmicutes, Proteobacteria, and Bacteroidetes, comprising up to 96% of the diversity. Fig. 6 reveals the bacterial diversity at the family or at the next highest resolution level. Although there is great diversity in all the analysed samples, an enrichment of OTUs with low representativeness in the inoculum and blackwater could be seen in the no-GAC control and in GAC-amended groups. Some OTUs were slightly enriched in GAC-amended cultures, such as uncultured members of the orders Bacteroidales (4.9%) and Chlorobiales (3.5%), and of the families Spirochaetaceae (1.7%), Marinilabiaceae (2.1%), Bacteroidaceae (2.7%) and Lachnospiraceae (3.0%). In the control group, the Bacteroidales order represented 2.0% of the sequences, while Spirochaetaceae, Marinilabiaceae, Bacteroidaceae, and Lachnospiraceae families represented 0.3, 0, 0.9, and 1.1% of the sequences, respectively. Members of the Spirochaetaceae family have been reported to play a role in hydrolysis during anaerobic digestion,39 while OTUs affiliated with the family Marinilabiaceae have been reported to be more represented in the inoculum than in the digested cultures.28 Li et al. (2015a)40 and Miura et al. (2016),41 however, detected Marinilabiaceae-related sequences in a non-saline mesophilic digester and in methanogenic cultures treating sugar and salt-containing brown algae under undiluted conditions. Members of Bacteroidaceae and Lachnospiraceae families are classified as cellulolytic microorganisms,42 and carbohydrate-fermenting bacteria are known to produce large amounts of butyrate, acetate, and carbon dioxide.43
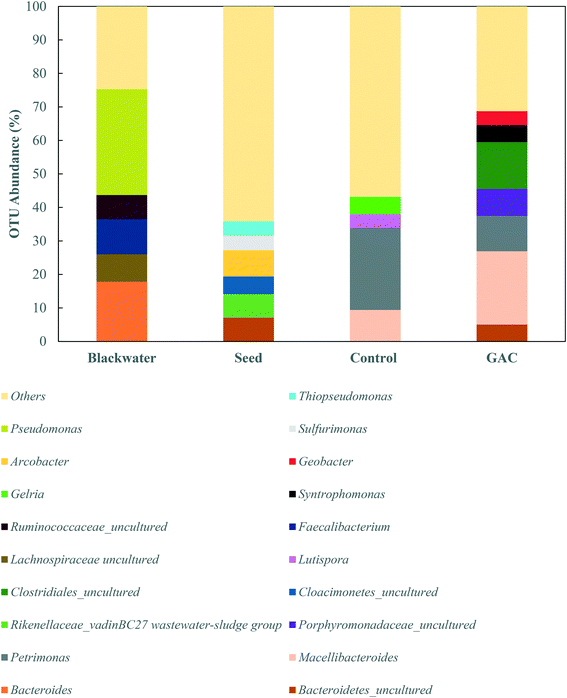 |
| Fig. 6 Relative abundances of phylogenetic bacterial groups in the inoculum, blackwater, the control group, and the GAC-amended group. Bacterial communities are presented in taxonomic family or at the next highest resolution level. Phylogenetic groups accounting for ≤4.0% of classified sequences are summarized as “other.” | |
The greatest abundance in both cultures, however, were members of the Porphyromonadaceae family (35.7–37.3%) and Clostridiales order (16.6–22.0%), which comprise syntrophic bacteria known to provide acetate for methanogenic archaea during anaerobic digestion.44,45 The major genera in the Porphyromonadaceae family in both cultures were Petrimonas (10.5–24.4%) and Macellibacteroides (9.4–21.9%), both classified as mesophilic fermentative bacteria, with growth in temperature ranges of 15–35 °C and 20–45 °C, respectively.46,47 Members of the Petrimonas genus have the ability to ferment sugars generating acetate and propionate as major products,46 while Macellibacteroides are reported to ferment sugars producing mainly lactate, acetate, butyrate, and isobutyrate.47
The addition of conductive material to the cultures clearly led to an enrichment of the Geobacteraceae family (4.0%), while in the no-GAC control group Geobacteraceae represented only 0.3% of the diversity. Such microorganisms are known to perform direct interspecies electron transfer in co-cultures with methanogenic archaea by oxidizing a broad range of organic substrates.48,49Geobacter genus-related microorganisms were reported to be key players in studies utilizing granular activated carbon to improve methane production in anaerobic reactors,22,30 in cultures supplemented with conductive iron oxide particles,50 and in conductive materials-free systems.6,34 The conductivity of Geobacter-related aggregates is reported to range from 2–20 μS cm−1,34,51,52 while the conductivity of GAC measured under the same conditions was around 3000 ± 327 μS cm−1,51 which is in line with values reported previously for GAC conductivity.53 Therefore, although Geobacteraceae-affiliated microorganisms have the ability to biologically establish electrical connections with some methanogenic archaea, GAC might have enhanced their syntrophic metabolism due to its much higher conductivity, playing an electron acceptor role during acetate oxidation.
Archaeal community.
The most representative OTUs in the sludge used as inoculum belonged to the genera Methanospirillum (59.6%), Methanoculleus (27.2%), and Methanolinea (4.5%). The anaerobic degradation of blackwater in the control group shifted the archaeal community toward members of the genera Methanosarcina (64.1%) and Methanoculleus (15.2%), and unclassified members of the Methanomicrobiales order (13.8%). The addition of conductive material to the cultures did not affect the representativeness of Methanosarcina (62.9%), but enriched unclassified Methanobacteriaceae-affiliated microorganisms (27.8%) and decreased the abundance of Methanoculleus representatives to 4.5%. Fig. 7 gives an overview of the microbial community structure of each condition studied. As also shown by Gao et al. (2018),10 anaerobic degradation of blackwater did not enable the retention of microorganisms related to the Methanospirillum genus in the cultures. The drastic elimination of this hydrogen-utilizing methanogenic OTU might be explained by the general shifts in community structure that always occur in the direction of more robust microorganisms that are better at dealing with and adapting to harsh conditions.54
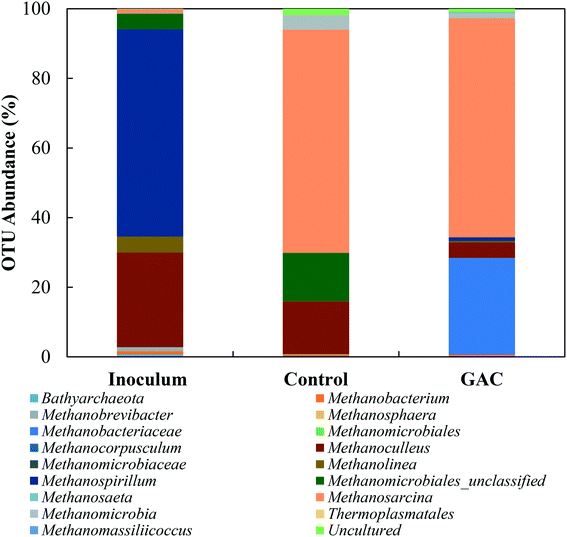 |
| Fig. 7 Relative abundances of phylogenetic archaeal groups in the seed sludge, blackwater, control group, and GAC-amended group. Archaeal communities are presented in taxonomic genus or at the next highest resolution level. | |
Li et al. (2016),55 Goux et al. (2015),56 Schluter et al. (2008),57 and Franke-Whittle et al. (2014)57 reported a predominance of the hydrogenotroph Methanoculleus genus under stress conditions, and the authors related such dominance to the ability of Methanoculleus to tolerate high concentrations of ammonia.58 In this study, despite the high concentration of ammonia, Methanoculleus genus-affiliated members were more abundant in the control group, while in the GAC-amended culture its abundance decreased by approximately 3.4-fold, with a replacement by unclassified members of the hydrogenotrophic Methanobacteriaceae family. A reasonable explanation for Methanobacteriaceae to stand in might be the ability of its members to directly accept electrons transferred from syntrophic bacteria with mediation of the conductive material added to the cultures, as suggested by Dubé and Guiot (2015).59 Together with the above-mentioned enrichment of Geobacteraceae-affiliated bacteria, it is likely that GAC stimulated the growth of electroactive species by facilitating electron transfer between them.
Versatile Methanosarcina genus members did not seem to be affected by the high conductivity applied to the cultures. Methanosarcina species are largely reported as an abundant methanogenic group enriched during anaerobic digestion. The high representativeness of this OTU can be related to its diverse metabolism. Methanosarcina metabolize acetate, hydrogen, formate, ethanol, isopropanol, and methylated compounds,60 and accept electron transfer from syntrophic bacteria upon acetate oxidation.48 In large abundance, Methanosarcina effectively buffer against fluctuations in substrate availability, preventing accumulation or shock loading of acetic acid in the environment.61,62 The ability of Methanosarcina species to function as hydrogen scavengers has been reported in investigations of syntrophic ammonia-oxidizing-dominated processes, and occasionally at relatively high ammonia levels.15,16,63 Moreover, members of the Methanosarcina genus have a tendency to form dense aggregates, which might have assisted their propagation on the surface of GAC and their protection from high levels of ammonia in the medium.64
Concluding remarks
Methane production from the anaerobic degradation of blackwater was improved up to 18.6% by GAC in the presence of high concentrations of free ammonia. The methane production was in accordance with the VFA profile, with accumulation of acetate in the no-GAC and PE control groups and complete depletion in GAC-amended cultures. The addition to cultures of conductive material promoted a shift in the microbial community toward syntrophic bacteria and methanogenic archaea which likely participated in direct electron transfer. The genus Methanosarcina was dominant in all analyzed conditions; and metabolic versatility, a large cell size, and a unique mode of aggregated growth allow Methanosarcina to thrive in harsh conditions. The results suggest that GAC application is an effective strategy to overcome ammonia inhibition of methanogenesis during anaerobic degradation of blackwater. As a reusable material, GAC, improves methane production in anaerobic digesters in a sustainable way, thus, GAC application is a cost-effective strategy for converting waste to biogas on a large scale. Further studies are required to assess direct interspecies electron transfer (DIET) in blackwater digestion, the factors that affect its efficiency such as particle size and C/N ratio, and the competence of DIET in continuous processes.
Conflicts of interest
There are no conflicts to declare.
Acknowledgements
The authors acknowledge financial support from the Natural Sciences and Engineering Research Council of Canada (NSERC) Collaborative Research and Development (CRD) Grant, an NSERC Industrial Research Chair (IRC) Program in Sustainable Urban Water Development (Liu, Y.) through the support of EPCOR Water Services, EPCOR Drainage, Alberta Innovates, and WaterWerx, the Canada Research Chair (CRC) in Future Community Water Services (Liu, Y.), and a Mitacs Accelerate Postdoctoral Fellowship (Zhang, L.). We thank Dr. Bipro Ranjan Dhar (University of Alberta, Alberta, Canada) for support with conductivity measurements.
References
- B. Vinnerås, H. Palmquist, P. Balmér and H. Jönsson, The characteristics of household wastewater and biodegradable solid waste—A proposal for new Swedish design values, Urban Water J., 2006, 3(1), 3–11 CrossRef.
-
T. Z. D. d. Mes, A. J. M. Stams, J. H. Reith and G. Zeeman, Bio-methane & Bio-hydrogen: status and perspectives of biological methane and hydrogen production, Dutch Biological Hydrogen Foundation, Petten, 2003, pp. 58–102 Search PubMed.
-
B. E. Rittmann and P. L. McCarty, Environmental biotechnology : principles and applications, Boston McGraw-Hill, 2001 Search PubMed.
- M. S. Fountoulakis and T. Manios, Enhanced methane and hydrogen production from municipal solid waste and agro-industrial by-products co-digested with crude glycerol, Bioresour. Technol., 2009, 100(12), 3043–3047 CrossRef CAS PubMed.
- Y. Chen, J. J. Cheng and K. S. Creamer, Inhibition of anaerobic digestion process: a review, Bioresour. Technol., 2008, 99(10), 4044–4064 CrossRef CAS PubMed.
- P. M. Shrestha and A. E. Rotaru, Plugging in or going wireless: strategies for interspecies electron transfer, Front. Microbiol., 2014, 5, 237 Search PubMed.
- M. A. Mir, A. Hussain, C. Verma and S. Dubey, Design considerations and operational performance of anaerobic digester: A review, Cogent Eng., 2016, 3(1), 1181696 Search PubMed.
- Y. Shen, J. L. Linville, P. A. A. Ignacio-de Leon, R. P. Schoene and M. Urgun-Demirtas, Towards a sustainable paradigm of waste-to-energy process: Enhanced anaerobic digestion of sludge with woody biochar, J. Cleaner Prod., 2016, 135, 1054–1064 CrossRef CAS.
- Z. Zhao, Y. Zhang, D. E. Holmes, Y. Dang, T. L. Woodard, K. P. Nevin and D. R. Lovley, Potential enhancement of direct interspecies electron transfer for syntrophic metabolism of propionate and butyrate with biochar in up-flow anaerobic sludge blanket reactors, Bioresour. Technol., 2016, 209, 148–156 CrossRef CAS PubMed.
- M. Gao, L. Zhang, A. P. Florentino and Y. Liu, Performance of Anaerobic Treatment of Blackwater Collected from Different Toilet Flushing Systems: Can We Achieve Both Energy Recovery and Water Conservation?, J. Hazard. Mater., 2018 DOI:10.1016/j.jhazmat.2018.10.055.
- M. Westerholm, L. Leven and A. Schnurer, Bioaugmentation of syntrophic acetate-oxidizing culture in biogas reactors exposed to increasing levels of ammonia, Appl. Environ. Microbiol., 2012, 78(21), 7619–7625 CrossRef CAS PubMed.
- I. A. Fotidis, D. Karakashev, T. A. Kotsopoulos, G. G. Martzopoulos and I. Angelidaki, Effect of ammonium and acetate on methanogenic pathway and methanogenic community composition, FEMS Microbiol. Ecol., 2013, 83(1), 38–48 CrossRef CAS PubMed.
- L. P. Hao, L. Mazeas, F. Lu, J. Grossin-Debattista, P. J. He and T. Bouchez, Effect of ammonia on methane production pathways and reaction rates in acetate-fed biogas processes, Water Sci. Technol., 2017, 75(7–8), 1839–1848 CrossRef CAS PubMed.
- A. Schnurer and A. Nordberg, Ammonia, a selective agent for methane production by syntrophic acetate oxidation at mesophilic temperature, Water Sci. Technol., 2008, 57(5), 735–740 CrossRef CAS PubMed.
- M. Westerholm, J. Dolfing, A. Sherry, N. D. Gray, I. M. Head and A. Schnurer, Quantification of syntrophic acetate-oxidizing microbial communities in biogas processes, Environ. Microbiol. Rep., 2011, 3(4), 500–505 CrossRef CAS PubMed.
- T. Shimada, E. Morgenroth, M. Tandukar, S. G. Pavlostathis, A. Smith, L. Raskin and R. E. Kilian, Syntrophic acetate oxidation in two-phase (acid-methane) anaerobic digesters, Water Sci. Technol., 2011, 64(9), 1812–1820 CrossRef CAS PubMed.
- J. Wang, J. Zhang, H. Xie, P. Qi, Y. Ren and Z. Hu, Methane emissions from a full-scale A/A/O wastewater treatment plant, Bioresour. Technol., 2011, 102, 5479–5485 CrossRef CAS PubMed.
- A. M. Zealand, A. P. Roskilly and D. W. Graham, Effect of feeding frequency and organic loading rate on biomethane production in the anaerobic digestion of rice straw, Appl. Energy, 2017, 207, 156–165 CrossRef CAS.
- D. R. Lovley, Syntrophy goes electric: direct interspecies electron transfer, Annu. Rev. Microbiol., 2017, 71, 26 CrossRef PubMed.
- F. Liu, A.-E. Rotaru, P. M. Shrestha, N. S. Malvankar, K. P. Nevin and D. R. Lovley, Promoting direct interspecies electron transfer with activated carbon, Energy Environ. Sci., 2012, 5(10), 8982 RSC.
- Y. Dang, D. Sun, T. L. Woodard, L. Y. Wang, K. P. Nevin and D. E. Holmes, Stimulation of the anaerobic digestion of the dry organic fraction of municipal solid waste (OFMSW) with carbon-based conductive materials, Bioresour. Technol., 2017, 238, 30–38 CrossRef CAS PubMed.
- J. Y. Lee, S. H. Lee and H. D. Park, Enrichment of specific electro-active microorganisms and enhancement of methane production by adding granular activated carbon in anaerobic reactors, Bioresour. Technol., 2016, 205, 205–212 CrossRef CAS PubMed.
- Y. Dang, D. E. Holmes, Z. Zhao, T. L. Woodard, Y. Zhang, D. Sun, L. Y. Wang, K. P. Nevin and D. R. Lovley, Enhancing anaerobic digestion of complex organic waste with carbon-based conductive materials, Bioresour. Technol., 2016, 220, 516–522 CrossRef CAS PubMed.
- Z. Zhao, Y. Zhang, Q. Yu, Y. Dang, Y. Li and X. Quan, Communities stimulated with ethanol to perform direct interspecies electron transfer for syntrophic metabolism of propionate and butyrate, Water Res., 2016, 102, 475–484 CrossRef CAS PubMed.
- J. G. Caporaso, J. Kuczynski, J. Stombaugh, K. Bittinger, F. D. Bushman, E. K. Costello, N. Fierer, A. G. Pena, J. K. Goodrich, J. I. Gordon, G. A. Huttley, S. T. Kelley, D. Knights, J. E. Koenig, R. E. Ley, C. A. Lozupone, D. McDonald, B. D. Muegge, M. Pirrung, J. Reeder, J. R. Sevinsky, P. J. Turnbaugh, W. A. Walters, J. Widmann, T. Yatsunenko, J. Zaneveld and R. Knight, QIIME allows analysis of high-throughput community sequencing data, Nat. Methods, 2010, 7(5), 335–336 CrossRef CAS PubMed.
- C. Quast, E. Pruesse, P. Yilmaz, J. Gerken, T. Schweer, P. Yarza, J. Peplies and F. O. Glockner, The SILVA ribosomal RNA gene database project: improved data processing and web-based tools, Nucleic Acids Res., 2013, 41(Database issue), D590–596 CAS.
- K. H. Hansen, I. Angelidaki and B. K. Ahring, Improving thermophilic anaerobic digestion of swine manure, Water Res., 1999, 33(8), 1805–1810 CrossRef CAS.
- S. Poirier, C. Madigou, T. Bouchez and O. Chapleur, Improving anaerobic digestion with support media: Mitigation of ammonia inhibition and effect on microbial communities, Bioresour. Technol., 2017, 235, 229–239 CrossRef CAS PubMed.
- W. Yan, N. Shen, Y. Xiao, Y. Chen, F. Sun, V. Kumar Tyagi and Y. Zhou, The role of conductive materials in the start-up period of thermophilic anaerobic system, Bioresour. Technol., 2017, 239, 336–344 CrossRef CAS PubMed.
- Y. Yang, Y. Zhang, Z. Li, Z. Zhao, X. Quan and Z. Zhao, Adding granular activated carbon into anaerobic sludge digestion to promote methane production and sludge decomposition, J. Cleaner Prod., 2017, 149, 1101–1108 CrossRef CAS.
- S. Lozecznik, R. Sparling, J. A. Oleszkiewicz, S. Clark and J. F. VanGulck, Leachate treatment before injection into a bioreactor landfill: clogging potential reduction and benefits of using methanogenesis, Waste Manage., 2012, 30(11), 2030–2036 CrossRef PubMed.
- K. A. Taconi, M. E. Zappi, W. Todd French and L. R. Brown, Methanogenesis under acidic pH conditions in a semi-continuous reactor system, Bioresour. Technol., 2008, 99(17), 8075–8081 CrossRef CAS PubMed.
- V. Siriwongrungson, R. J. Zeng and I. Angelidaki, Homoacetogenesis as the alternative pathway for H2 sink during thermophilic anaerobic degradation of butyrate under suppressed methanogenesis, Water Res., 2007, 41(18), 4204–4210 CrossRef CAS PubMed.
- M. Morita, N. S. Malvankar, A. E. Franks, Z. M. Summers, L. Giloteaux, A. E. Rotaru, C. Rotaru and D. R. Lovley, Potential for direct interspecies electron transfer in methanogenic wastewater digester aggregates, mBio, 2011, 2(4), 8 CrossRef PubMed.
- Q. Cheng and D. F. Call, Hardwiring microbes via direct interspecies electron transfer: mechanisms and applications, Environ. Sci.: Processes Impacts, 2016, 18(8), 968–980 RSC.
- K. Sasaki, F. Takasaki, Z. Noda, S. Hayashi, Y. Shiratori and K. Ito, Alternative electrocatalyst support materials for polymer electrolyte fuel cells, ECS Trans., 2010, 33(1), 10 Search PubMed.
- K. Sasaki, M. Morita, S. Hirano, N. Ohmura and Y. Igarashi, Decreasing ammonia inhibition in thermophilic methanogenic bioreactors using carbon fiber textiles, Appl. Microbiol. Biotechnol., 2011, 90(4), 1555–1561 CrossRef CAS PubMed.
- H. Jin, Y. S. Lee and I. Hong, Hydrogen adsorption characteristics of activated carbon, Catal. Today, 2007, 120(3–4), 399–406 CrossRef CAS.
- G. T. Shaw, A. C. Liu, C. Y. Weng, C. Y. Chou and D. Wang, Inferring microbial interactions in thermophilic and mesophilic anaerobic digestion of hog waste, PLoS One, 2017, 12(7), e0181395 CrossRef PubMed.
- Y. F. Li, M. C. Nelson, P. H. Chen, J. Graf, Y. Li and Z. Yu, Comparison of the microbial communities in solid-state anaerobic digestion (SS-AD) reactors operated at mesophilic and thermophilic temperatures, Appl. Microbiol. Biotechnol., 2015, 99(2), 969–980 CrossRef CAS PubMed.
- T. Miura, A. Kita, Y. Okamura, T. Aki, Y. Matsumura, T. Tajima, J. Kato and Y. Nakashimada, Semi-continuous methane production from undiluted brown algae using a halophilic marine microbial community, Bioresour. Technol., 2016, 200, 616–623 CrossRef CAS PubMed.
-
M. T. Madigan, K. S. Bender, D. H. Buckley, W. M. Sattley and D. A. Stahl, Brock Biology of Microorganisms, Pearson, Boston, 2017 Search PubMed.
- J. H. Cummings and G. T. Macfarlane, Gastrointestinal effects of prebiotics, J. Geophys. Res. Oceans, 2007, 87(S2), S145–S151 Search PubMed.
- L. Y. Wang, K. P. Nevin, T. L. Woodard, B. Z. Mu and D. R. Lovley, Expanding the Diet for DIET: Electron Donors Supporting Direct Interspecies Electron Transfer (DIET) in Defined Co-Cultures, Front. Microbiol., 2016, 7, 236 Search PubMed.
- J. R. Sieber, D. R. Sims, C. Han, E. Kim, A. Lykidis, A. L. Lapidus, E. McDonnald, L. Rohlin, D. E. Culley, R. Gunsalus and M. J. McInerney, The genome of Syntrophomonas wolfei: new insights into syntrophic metabolism and biohydrogen production, Environ. Microbiol., 2010, 12(8), 2289–2301 CAS.
- A. Grabowski, B. J. Tindall, V. Bardin, D. Blanchet and C. Jeanthon, Petrimonas sulfuriphila gen. nov., sp. nov., a mesophilic fermentative bacterium isolated from a biodegraded oil reservoir, Int. J. Syst. Evol. Microbiol., 2005, 55(Pt 3), 1113–1121 CrossRef CAS PubMed.
- L. Jabari, H. Gannoun, J. L. Cayol, A. Hedi, M. Sakamoto, E. Falsen, M. Ohkuma, M. Hamdi, G. Fauque, B. Ollivier and M. L. Fardeau, Macellibacteroides fermentans gen. nov., sp. nov., a member of the family Porphyromonadaceae isolated from an upflow anaerobic filter treating abattoir wastewaters, Int. J. Syst. Evol. Microbiol., 2012, 62(Pt 10), 2522–2527 CrossRef CAS PubMed.
- A. E. Rotaru, T. L. Woodard, K. P. Nevin and D. R. Lovley, Link between capacity for current production and syntrophic growth in Geobacter species, Front. Microbiol., 2015, 6, 744 Search PubMed.
- H. Li, J. Chang, P. Liu, L. Fu, D. Ding and Y. Lu, Direct interspecies electron transfer accelerates syntrophic oxidation of butyrate in paddy soil enrichments, Environ. Microbiol., 2015, 17(5), 1533–1547 CrossRef PubMed.
- S. Kato, K. Hashimoto and K. Watanabe, Microbial interspecies electron transfer via electric currents through conductive minerals, Proc. Natl. Acad. Sci. U. S. A., 2012, 109(25), 5 CrossRef PubMed.
- Z. M. Summers, H. E. Fogarty, C. Leang, A. E. Franks, N. S. Malvankar and D. R. Lovley, Direct exchange of electrons within aggregates of an evolved syntrophic coculture of anaerobic bacteria, Science, 2010, 330(6009), 1413–1415 CrossRef CAS PubMed.
- D. R. Bond and D. R. Lovley, Electricity Production by Geobacter sulfurreducens Attached to Electrodes, Appl. Environ. Microbiol., 2003, 69(3), 1548–1555 CrossRef CAS PubMed.
- B. Kastening, M. Hahn, M. Rabanus, M. Heins and U. zum Felde, Electronic properties and double layer of activated carbon, Electrochim. Acta, 1997, 42(18), 11 CrossRef.
- J. De Vrieze, T. Hennebel, N. Boon and W. Verstraete, Methanosarcina: the rediscovered methanogen for heavy duty biomethanation, Bioresour. Technol., 2012, 112, 1–9 CrossRef CAS PubMed.
- L. Li, Q. He, Y. Ma, X. Wang and X. Peng, A mesophilic anaerobic digester for treating food waste: process stability and microbial community analysis using pyrosequencing, Microb. Cell Fact., 2016, 15, 65 CrossRef PubMed.
- X. Goux, M. Calusinska, S. Lemaigre, M. Marynowska, M. Klocke, T. Udelhoven, E. Benizri and P. Delfosse, Microbial community dynamics in replicate anaerobic digesters exposed sequentially to increasing organic loading rate, acidosis, and process recovery, Biotechnol. Biofuels, 2015, 8, 122 CrossRef PubMed.
- A. Schluter, T. Bekel, N. N. Diaz, M. Dondrup, R. Eichenlaub, K. H. Gartemann, I. Krahn, L. Krause, H. Kromeke, O. Kruse, J. H. Mussgnug, H. Neuweger, K. Niehaus, A. Puhler, K. J. Runte, R. Szczepanowski, A. Tauch, A. Tilker, P. Viehover and A. Goesmann, The metagenome of a biogas-producing microbial community of a production-scale biogas plant fermenter analysed by the 454-pyrosequencing technology, J. Biotechnol., 2008, 136(1–2), 77–90 CrossRef PubMed.
- I. H. Franke-Whittle, A. Walter, C. Ebner and H. Insam, Investigation into the effect of high concentrations of volatile fatty acids in anaerobic digestion on methanogenic communities, Waste Manage., 2014, 34(11), 2080–2089 CrossRef CAS PubMed.
-
C.-D. Dubé and S. R. Guiot, Biogas Science and Technology, ed. G. M. Guebitz, A. Bauer, G. Bochmann, A. Gronauer and S. Weiss, Springer International Publishing, Cham, 2015, pp. 101–115 Search PubMed.
-
M. M. Kendall and D. R. Boone, The Order Methanosarcinales, 2006, pp. 244–256 Search PubMed.
- A. Conklin, H. D. Stensel and J. Ferguson, Growth kinetics and competition between Methanosarcina and Methanosaeta in mesophilic anaerobic digestion, Water Environ. Res., 2006, 78(5), 486–496 CrossRef CAS PubMed.
- T. Hori, S. Haruta, Y. Ueno, M. Ishii and Y. Igarashi, Dynamic transition of a methanogenic population in response to the
concentration of volatile fatty acids in a thermophilic anaerobic digester, Appl. Environ. Microbiol., 2006, 72(2), 1623–1630 CrossRef CAS PubMed.
- J. Moestedt, B. Muller, M. Westerholm and A. Schnurer, Ammonia threshold for inhibition of anaerobic digestion of thin stillage and the importance of organic loading rate, Microb. Biotechnol., 2016, 9(2), 180–194 CrossRef CAS PubMed.
- L. Hao, F. Lu, L. Mazeas, E. Desmond-Le Quemener, C. Madigou, A. Guenne, L. Shao, T. Bouchez and P. He, Stable isotope probing of acetate fed anaerobic batch incubations shows a partial resistance of acetoclastic methanogenesis catalyzed by Methanosarcina to sudden increase of ammonia level, Water Res., 2015, 69, 90–99 CrossRef CAS PubMed.
|
This journal is © The Royal Society of Chemistry 2019 |
Click here to see how this site uses Cookies. View our privacy policy here.