Trophic transfer of CuO NPs and dissolved Cu from sediment to worms to fish – a proof-of-concept study†
Received
22nd January 2019
, Accepted 16th February 2019
First published on 18th February 2019
Abstract
Man-made nanoparticles (NPs) released into the aquatic environment are likely to accumulate in sediment. Uptake of NPs by benthic invertebrates that serve as food for higher trophic level organisms such as fish may result in their transfer along the food chain. We tested this hypothesis in a proof-of-concept approach consisting of two studies examining the transfer of copper(II) oxide NPs (CuO NPs) (<50 nm) and dissolved copper (CuCl2). Study 1 focussed on transfer from sediment to worms (Tubifex tubifex) and study 2 on transfer from worms (T. tubifex) to fish (Gasterosteus aculeatus, three-spined stickleback). A key methodological achievement of study 2 was the development of worm homogenate-based food packages, which allowed controlling CuO NPs and CuCl2 dosing of fish. In T. tubifex, no significant differences in Cu accumulation were detected following 7 days of exposure to sediment-associated CuO NPs or CuCl2 (100 μg Cu per g dw sediment). Yet, metallothionein-like protein levels were significantly elevated in CuO NP-exposed worms compared to the control, while they were not in CuCl2-exposed worms. G. aculeatus fed CuO NP- and CuCl2-spiked food packages (2 μg Cu per g fish ww per day; 7 days) showed increased Cu concentrations (∼13 and ∼31 μg Cu per g dw compared to control, respectively) and metallothionein (mta) mRNA expression in intestinal tissue. In CuO NP-exposed fish, Cu and mta mRNA levels were noticeably lower than in CuCl2-exposed fish and inversely correlated with the amount of Cu in their faeces, suggesting that a large fraction of NPs was egested. Altogether, the results suggest that sediment-associated CuO NPs can enter the aquatic food web, but that transfer of CuO NPs from T. tubifex to stickleback may be limited compared to dissolved Cu. Furthermore, there were no indications of induction of oxidative stress in fish intestine and liver, suggesting that effects from environmentally relevant Cu/CuO NP exposure via the diet may be minimal.
Environmental significance
Man-made nanoparticles (NPs) may accumulate in sediment and be taken up by benthic invertebrates that serve as food for higher trophic level organisms, such as fish. The current knowledge and understanding of the dietary uptake and bioaccumulation of NPs in aquatic organisms is limited. Even less is known on their fate within food webs. Important questions in this context are if and how uptake, bioaccumulation and trophic transfer of NPs differ compared to non-nanoparticulate counterparts. In this proof-of-concept study we present a strategy to examine trophic transfer along simplified freshwater food chains including sediment, endobenthic worms and predatory fish in a highly controlled manner. Our results show differential uptake, fate and biological effects of copper(II) oxide (CuO) NPs and dissolved Cu in the model organisms used. Our results suggest that chemical and biological processes in sediment, food/prey and predator can result in NP transformation and should receive more attention in future research studies to generate sufficient knowledge to be able to adequately account for and incorporate environmental realism in the safety evaluation of NPs.
|
Introduction
Nanoparticles (NPs) are increasingly produced and will inevitably end up in the environment. In order to evaluate the environmental risk of these NPs, information on bioaccumulation, trophic transfer in food webs, and adverse effects towards organisms is vital. While the uptake and effects of these particles in short-term water-only laboratory tests have been widely examined in controlled freshwater systems,1 only a few studies have focused on dietary/sediment exposure even though most NPs (incl. CuO NPs) will agglomerate/aggregate upon introduction to the aquatic environment and settle on the sediment surface often to levels several fold higher than in overlying water.1–5 Due to this behaviour of NPs, sediment-dwelling organisms, such as molluscs and worms, are at particular risk of NP exposure.6–11 In aquatic environments, sediment-associated Cu is known to accumulate in tubificid species, often to a higher extent than in other non-deposit feeding species, such as fish (including sticklebacks). However, trophic transfer of metals, such as Cu, is occurring in the environment and accumulation beyond threshold levels for essential metals should not be neglected. Bioavailability of sediment-associated CuO NPs has been reported for endobenthic worms, both in estuarine and freshwater systems (for instance, Nereis (Hediste) diversicolor and Lumbriculus variegatus2,8,9), which constitute a major food source for fish.
Tangaa et al. (2016) reviewed the available literature on trophic transfer of metal NPs and found that little information was available, thus emphasizing the need for more information on this subject.13 Most of the studies carried out to this day have investigated NP transfer along simplified food chains using diatoms or algae as trophic level one organisms (producers) and crustaceans, bivalves or snails as trophic level two organisms (primary consumers).12–14 Comparatively few studies examined NP transfer along trophic chains including fish.15–22 Among these studies all except one20 worked with pelagic prey organisms (mainly artemia15,17,22 and daphnia16,19,21), and the majority thereof used zebrafish as the model predator species.16–19,21,22 Prey–predatory transfer of the used NPs (or the constituting metal element) was observed in all published papers, which confirms the results from dietary uptake studies, where fish were fed artificial food.23–26 However, no biomagnification was reported in any of the studies. To the best of our knowledge only a single study specifically examined the trophic transfer of NPs from benthic invertebrates to fish, namely from the clamworm (Perinereis aibuhitensis) to juvenile turbot (Scophthalmus maximus),20 and the influence of fish digestive physiology on NP properties, dietary availability and bioaccumulation has not been studied.
Since uptake and accumulation of NPs in sediment-ingesting organisms may comprise a major input to the food web, the overall aim of the current study was to study the trophic transfer of CuO NPs from sediment-dwelling worms to fish using spiked sediments. The specific objectives were to examine 1) the introduction of sediment-associated CuO NPs to the benthic food web (from sediment to worm) and 2) trophic transfer from prey (worms) to predator (fish) and 3) to assess potential effects resulting from NP exposure. The oligochaete worm Tubifex tubifex and the fish three-spined stickleback (Gasterosteus aculeatus) were chosen as model organisms to simulate a two-step food chain. Both species are widely distributed geographically and coexist in freshwater ecosystems. T. tubifex is a widely used standard test organism for bioaccumulation studies as described in the OECD Test Guideline 315 on bioaccumulation in sediment-dwelling benthic oligochaetes.27 As a deposit-feeder, this species is at particular risk of exposure to sediment-associated metals and metal NPs. The three-spined stickleback is also widely used in ecotoxicology testing. This species is particularly relevant in the current study since it forages at the bottom and feeds on a variety of items from the river bed, including tubificid worms. Dissolved Cu, administered as CuCl2, was used as a reference treatment as previously recommended.28
Recognizing the complexity of designing, conducting and interpreting trophic transfer studies, here we used a proof-of-concept approach. In brief, fish were fed for up to one week with food packages (made from T. tubifex tissue homogenates, which were spiked with CuCl2 or CuO NPs or clean Milli-Q (MQ) water as a negative control). The originality of this work is the use of spiked worm homogenates, which allowed having the highest possible control of Cu concentrations in the feed and to control the dietary exposure dose of individual fish. Furthermore, considering the potential influence of the organism's digestive physiology on NP dissolution and hence availability and bioaccumulation,29 we complemented our dietary exposure study with a number of in vitro experiments to obtain information on CuO NP dissolution in the gastrointestinal tract.
1. Experimental
1.1. General experimental design
Overall, two separate studies were conducted exposing worms and fish to three dietary treatments (clean/control, CuCl2 or CuO NP) simulating transfer from sediment to worms (study 1) and from worms to fish (study 2). In study 1, worms were exposed via sediment for 7 days and in study 2, fish were fed food packages made from worm homogenates for up to 7 days.
1.2. Test compounds
CuO NPs were purchased from Sigma-Aldrich Sweden AB (Stockholm, SE) as nanopowder (CuO NPs <50 nm (TEM), CAS: 1317-38-0). The manufacturer reported a primary size of below 50 nm and a surface area of 29 m2 g−1. CuCl2·2H2O was used as a reference for dissolved Cu (including all Cu species). CuO NP stock dispersions prepared in MQ water (1 mg mL−1 Cu) were subjected to probe sonication to enhance dispersion. The protocol was adapted and modified from Taurozzi et al.30 and was successfully employed to disperse TiO2 NPs in one of our previous studies.31 In brief, CuO NP dispersions (∼10 ml) were sonicated for 20 min in pulse mode (1 s on/1 s off) at 10% maximum amplitude (∼20 W) using a Branson 250 sonifier equipped with a 3 mm tapered microtip while being kept in an ice-water bath. ICP-MS (inductively coupled plasma mass spectrometry) analysis after centrifugal ultrafiltration showed that sonication did not enhance CuO NP dissolution.
1.3. CuO NP characterization
Depending on the model organism and specific research question addressed, CuO NPs were characterized in different matrices and under different experimental conditions using a series of analytical techniques.
Transmission electron microscopy (TEM) was used to determine the primary particle size, aspect ratio (AR) and morphology of CuO NPs in the stock dispersions (see section 1.2). Carbon-coated copper grids were drop-coated with 10 μl of a 10 μg mL−1 CuO NP dispersion (stock diluted in MQ water) and then imaged on a Zeiss Leo 912 Omega TEM (120 kV) equipped with a 2k × 2k VELETA Olympus CCD camera. The primary size (minimum and maximum caliper diameter) and shape descriptors such as the AR of the imaged CuO NPs (n = 400) were measured using ImageJ software (ImageJ 1.49, National Institutes of Health, USA; http://imagej.nih.gov/ij).
Dynamic light scattering (DLS) analysis was performed to obtain information about the hydrodynamic size distribution of CuO NPs in the stock dispersions (see section 1.2). DLS was performed on 1
:
10 and 1
:
100 dilutions in MQ water of the sonicated stock dispersion (1 mg mL−1 Cu) using disposable polystyrene macro cuvettes (VWR International AB, Göteborg, SE) and a Zetasizer Nano-ZS apparatus (Malvern Instruments Ltd., Malvern, UK). Four consecutive measurements at ten runs in 173° back scatter detection mode were performed on each sample. The optimum measurement position and attenuation were set automatically. Results were calculated using the general purpose (normal resolution) analysis model. Zetasizer software version 7.11 (Malvern Instruments Ltd.) was used for data analysis and visualization.
The zeta potential was determined by measuring the electrophoretic mobility of CuO NPs in 1
:
10 and 1
:
100 dilutions of the sonicated stock dispersion (1 mg mL−1 Cu) using disposable capillary cuvettes and a Nano-ZS Zetasizer. Three consecutive measurements were performed on each sample. The number of runs was set automatically. The Smoluchowski approximation was used for zeta potential calculation.
CuO NP dissolution was studied using centrifugal filter units followed by Cu quantification with ICP-MS. The experimental and methodological details are described below.
1.4. CuO NP dissolution
A number of dissolution experiments (expdiss) were conducted to obtain information on CuO NP dissolution under different conditions. Specifically, it was tested whether a) the sonication protocol used to disperse the particles would increase the amount of dissolved Cu in the stock (expdiss. 1) and b) the NPs would dissolve in an acidic environment as it is prevalent in the fish stomach (expdiss. 2).
For expdiss. 1, 3 ml of the non-sonicated CuO NP stock dispersion (1 mg Cu per mL MQ) or 3 ml of the sonicated CuO NP stock dispersion were loaded onto Amicon Ultra-4 centrifugal filter units (Millipore, Merck AB, Solna, SE) with a molecular weight cut-off of 3 kDa. In addition, 3 ml of a CuCl2 stock dispersion of equivalent Cu concentration (1 mg Cu per mL MQ) was loaded onto a centrifugal filter unit to check for potential losses due to adsorption of dissolved Cu to the filter material/plastic (recovery control). Thereafter, the centrifugal filter units were spun at 4000g for 40 min in a Beckman Coulter Allegra X-15R centrifuge equipped with a swinging bucket rotor. Subsequently, the amount of Cu in the concentrate and the ultrafiltrate was quantified by ICP-MS (see section 1.7 for detailed information on sample processing for ICP-MS analysis). The measured concentrations of the loaded CuCl2 stock and of the concentrate and filtrate after ultrafiltration were 1013.9, 1008.2 and 999.1 μg Cu per mL MQ, respectively, demonstrating that recovery was close to ∼98%.
For expdiss. 2, the CuO NP stock dispersion was diluted in MQ water (pH 7) or in simulated gastric fluid (SGF; 2.0 g NaCl per L MQ, 0.0001 N HCl, pH 4) to a final concentration of 50 μg Cu per mL. A concentration of 50 μg Cu per mL was chosen to simulate the Cu concentration in the food packages (50 μg Cu per g ww food), that is the maximum Cu concentration expected to be present in the stomach (assuming that no further dilution or enrichment occurs). 3 ml of the 50 μg Cu per mL CuO NP dispersions in MQ water and in SGF were loaded onto Amicon Ultra-4 centrifugal filter units (see above for details) directly after their preparation and after incubation for 7.5 h on a rotary suspension mixer at room temperature (RT). An incubation time of 7.5 h was chosen as it corresponds to the gastric half-life of food/prey in three-spined stickleback.32 Ultrafiltration and Cu quantification in filtrates by ICP-MS was carried out as described above and in section 1.7. In parallel, a 50 μg Cu per mL CuCl2 solution was subjected to ultrafiltration to determine losses due to adsorption of dissolved Cu to the filter matrix/plastic. The measured concentration of the loaded CuCl2 stock and of the concentrate and filtrate after ultrafiltration were 51.8, 49.2 and 53.1 μg Cu per mL MQ, respectively, demonstrating that recovery was close to 100%.
Aliquots of the CuO NP dispersions subjected to the different treatments were also analysed by TEM to obtain information on how sonication and incubation at low pH would affect NP morphology.
1.5. Worm exposure and analysis
1.5.1. Test organism Tubifex tubifex.
T. tubifex were originally purchased from Bonnies Dyrecenter (Rødovre Centrum, Rødovre, DK). The worms were acclimatized for at least 24 h in the laboratory in glass aquaria filled with T. tubifex medium (see below). The worms were kept at 20 °C under full light. Aeration was provided using a pump, a silicon tube and an aeration stone.
T. tubifex medium was made from deionized water, calcium chloride (4 mM CaCl2·2H20, 10035-04-8, Merck), magnesium sulphate (1 mM MgS04·7H20, 10034-99-8, Merck), sodium bicarbonate (1.5 mM NaHC03, 144-55-8, Merck) and potassium chloride (0.15 mM KCl, 7447-40-7, Merck). The total volume was made up to 20 L with deionized water and aerated until oxygen saturation was achieved and stored at 20 °C. Sediment was collected from Roskilde Fjord, Munkholmbroen (55°40′25′′N, 11°48′44′′E) in October 2016 by scraping of the top few cm of sediment. The collected sediment was sieved (<0.5 mm) at the site using water from the site. Subsequently, the sediment was left to settle before the overlying water was removed. The sediment was then frozen at −20 °C for at least 24 h to kill macro- and meiofauna. Thawed sediment was subsequently sieved to <125 μm with tap water and rinsed twice with fresh, aerated T. tubifex medium and stored until experimental use (approximately one week). The dry weight to wet weight ratio (0.38 ± 0.05, n = 6) was determined after drying aliquots of approximately 1 g wet sediment for 24 h at 105 °C. The organic matter content (3.89 ± 0.93%, n = 6) on dried samples was determined as loss on ignition (LOI) for 6 h at 550 °C. The Cu concentration of natural sediment (<125 μm) was 8.0 ± 1.1 μg Cu per g dw sediment.
Sieved sediment was spiked by adding a known amount of the respective stock suspensions, CuO NP (12 g L−1) or CuCl2 (2.3 g L−1), to a known amount of wet sediment aiming at 100 μg Cu per g dw sediment. These exposure concentrations were chosen to be high enough to allow detection of bioaccumulation and biochemical responses in T. tubifex and, at the same time, low enough to ensure limited mortality during exposures. In addition, the Cu concentration is environmentally relevant: Cu concentrations in sediment in Europe are typically between 5 and 50 μg Cu per g dw sediment and may reach 4 mg Cu per g dw sediment.33,34 Clean MQ water was added to uncontaminated control sediment and CuO NP-spiked sediment in a volume similar to the maximum volume of stock added to the CuCl2 treatments. This was done to ensure similarity among all treatments. Equal volumes of aerated T. tubifex medium was added to obtain a sediment slurry and sediment was then mixed by hand and subsequently placed on a shaking table for 48 h to obtain a homogenous Cu distribution in the sediment.
1.5.2. Exposures of T. tubifex for Cu body burden and MT analysis.
Wet sediment (450 g ww; i.e., approximately 4 cm sediment layer) was transferred to one individual exposure beaker (1 L beakers; 14 cm height and 10.5 cm in diameter) per treatment, and aerated tubifex medium (500 ml; approximately 6 cm) was carefully added to avoid disturbing the sediment. Each beaker was aerated using an air pump with a silicone tube with a needle protruding from the overlying water and the system was left to settle for 24 h. Before exposure initiation, approximately 3/4 of the overlying water was exchanged with clean aerated Tubifex medium and the system was left to settle for 3 h before introducing T. tubifex. Subsequently, 12.7 g ww (corresponding to approximately 4000 worms) were carefully transferred into each of three exposure beakers (control, CuCl2 and CuO NP), wherein they immediately burrowed into the sediment. This significant number of worms was initially exposed to be used as feed for three-spined sticklebacks. After seven days of exposure, worms were sieved from the sediment, gently rinsed in Tubifex medium and transferred to a beaker containing clean medium for 20 h to empty their gut of sediment and any unassimilated Cu. This duration was chosen based on initial observations of depuration times needed to obtain a clean sediment-free gut. Selected samples were immediately stored at −80 °C for MT analysis (6 samples of 8 worms each) and Cu measurements (6 samples of 4 worms each). The remaining worms were stored at −20 °C for later trophic transfer studies. However, since Cu accumulation in these worms was low, any trophic transfer of Cu would likely be undetectable, and this approach was discarded. Instead, it was decided to proceed with producing food packages from spiked worm homogenates in the current study.
1.5.3. Analysis of metallothionein-like protein levels.
Worm tissue samples were homogenized on ice with 20 mM Trisma buffer (Trisma base, Sigma Aldrich, CAS: 77-86-1), 10−5 mM beta-mercaptoethanol (Sigma-Aldrich, CAS: 60-24-2), 150 mM NaCl (Sigma-Aldrich, 7647-14-5) and MQ water, and adjusted to pH 8.6 with HCl in a volume-to-weight ratio of 4 mL buffer per g worm tissue using a plastic pestle. Samples were transferred to a new sterile micro tube and the soluble and insoluble fractions were separated by centrifugation at 14
000 rpm (∼20
000g) at 4 °C for 55 min. The supernatant was transferred into a new sterile pre-weighed micro tube, and a 200 μl aliquot was then transferred into a new micro tube. The aliquot was heated at 75 °C for 15 min in a heating bath and subsequently kept for 10 min in ice water. Metallothionein-like proteins (MTLPs) were isolated by centrifugation at 13
000 rpm (15
000g) for 10 min at 4 °C. The supernatant was transferred into a new micro tube and frozen until further analysis. In the heat-denatured cytosol (S2), the amount of MTLPs was determined by differential pulse polarography (DPP; 797 VA Computracemetrohm), a technique based on –SH compound determination according to the Brdicka reaction (Brdicka, 1933). A sample aliquot (5 μl) was added to 10 mL freshly prepared ice-cold Bridcka buffer (ammonium chloride (Sigma-Aldrich, CAS: 12125-02-9), hexaamminecobalttrichloride (Sigma-Aldrich, CAS: 10534-89-1) and ammonium hydroxide solution (Sigma Aldrich, CAS: 1336-21-6) and MQ water). Standard addition was used for calibration of the DPP with rabbit liver metallothionein-1 (ENZO Life Sciences, ALX-202-072-M001) in the absence of a worm MT standard.
1.6. Dietary exposure and analysis of three-spined sticklebacks
1.6.1. Test organism: three-spined stickleback.
Three-spined sticklebacks (G. aculeatus) were caught from a small channel (58°13′57.4′′N, 11°28′22.9′′E) close to the marine research station Kristineberg, Sven Lovén Centre for Marine Infrastructure, on the island of Skaftö on the Swedish west coast in early April 2017. The fish were transported in cool boxes with well-oxygenated water to the Zoological Institute at the Department of Biological and Environmental Sciences, University of Gothenburg, where they were placed in a holding tank with a continuous flow-through of aerated artificial seawater with a temperature of ∼10 °C. After one week a subset of the fish was transferred to airstone-equipped 50 L glass aquaria, acclimatised to freshwater conditions by gradually replacing the initial artificial seawater with filtered, UV-treated tap water (∼0.15 mS cm−1, ∼10–14 °C) originating from the in-house semi-recirculating system over a period of ten days. Thereafter, the fish were kept under normal flow-through conditions until used for the dietary exposure study (∼2 months). During this time the fish were fed ad libitum with frozen chironomid larvae (Röda mygglarver, Akvarie Teknik).
1.6.2. Preparation of food packages.
Live T. tubifex (EAN 4038358100154) were purchased from Zoo.se (Bromma, SE) and cultured in a glass aquarium with aerated Tubifex medium for one week before being used for food package preparation. T. tubifex were collected in a 13 mL round based polypropylene (PP) tube (Sarstedt) and all excess water was aspirated using a micropipette. After determination of their wet weight (5 g) the worms were homogenized using a rotor–stator type tissue homogenizer (∼30 s at RT). Subsequently, 50 μl of red food colour containing carmine, ascorbic acid and potassium sorbate (Röd Hushållsfärg, Dr. Oetker) was added per 950 μl homogenate in order to reconstitute a “natural” colour. The tube with the T. tubifex homogenate was placed in a beaker with tepid water to adjust its temperature to that of a previously prepared 40–50 °C warm 0.3 g mL−1 gelatin solution (CAS: 9000-70-8, VWR international), with which it was subsequently mixed in a 1
:
1 (v/v) ratio. This “master-mix” was distributed into three 5 mL polystyrene (PS) tubes until each tube contained 2.375 g ww. Subsequently, 125 μl MQ water (for controls), 125 μl CuCl2 stock solution (1 mg Cu per mL MQ), 125 μl CuO NP stock dispersion (1 mg Cu per mL MQ; sonicated as described in section 1.2) were added to a tube each with 2.375 g ww master mix, resulting in nominal Cu concentrations of 50 μg Cu per g ww food. Spiking was performed immediately after sonication of the NP dispersion. The differently spiked homogenates were transferred into correspondingly labelled 1 mL disposable PP syringes (CodanTriplus AB, SE), which were immediately put on ice to initiate gelatinisation and subsequently stored at 4 °C until further use.
The syringes were equipped with a microlance (hypodermic needle, 1.20 × 50 mm BC/SB, B. Braun Melsungen AG, Melsungen, DE) through which the gelatinized T. tubifex homogenate was extruded and re-shaped into worm-like food packages of predefined diameter (1.2 mm) and adjustable length (accuracy ±0.5 mm) (Fig. S4A†). Knowing the food's density, it was thus possible to prepare daily rations corresponding exactly to 4% of each individual fish's body wet weight. The worm-like food packages were produced fresh each day and added to the tanks in palatable pieces of ∼10 mm length.
Leaching of Cu from CuCl2- and CuO NP-spiked food packages was assessed by incubating five 10 mm-long rations of each type (control, CuCl2- and CuO NP-spiked) in 3 mL MQ water for 30 min at 19 °C. After the incubation time 3 mL were carefully transferred to a fresh tube and the amount of Cu quantified by ICP-MS. Cu quantification was also done on the remaining food packages.
CuO NPs inside the food packages were characterized by means of TEM. The food packages were fixed in modified Karnovsky's fixative and postfixed in 1% osmium tetroxide (OsO4) including 1% potassium ferrocyanide. Following 5 min of incubation in 0.5% uranyl acetate, they were dehydrated in an ethanol gradient and then embedded in Agar 100 resin (Agar Scientific Ltd., UK). Ultrathin sections (∼70 nm) were collected on hexagonal 150 mesh copper grids and imaged on a Talos L120C (FEI, Thermo Scientific) equipped with a 4 × 4k CMOS Ceta camera.
1.6.3. Three-spined stickleback dietary exposure, sampling and tissue dissection.
Fish were exposed via diet (spiked food packages) for seven days. Two weeks prior to the start of the experiment, 36 sticklebacks of similar weight class (mean body ww: 1.42 ± 0.2 g) were selected and transferred to 36 individual experimental tanks (water volume: 1.5 L), i.e. each tank contained one fish. Eleven tanks were assigned to each treatment group (Cu-free control, CuCl2, CuO NP) (three tanks per sampling time point; day 1, 3 and 7, and two additional tanks as back-up in case some fish would not consume the spiked-food packages on any of the exposure days). The fish in the three remaining tanks not assigned to any treatment group were sampled before the start of the exposure (= t0 control). A flow-through system was used to maintain water quality and to minimize aqueous exposure to Cu potentially leaking from the food packages and/or egested faeces. Filtered tap water (background Cu concentration of 20 μg L−1) was supplied via an in-house semi-recirculating system at a flow rate of approximately 0.1 L min−1. During the first week, sticklebacks were fed frozen chironomid larvae; during the second week they were fed food packages without test substance (background Cu concentration: 1.4 μg Cu per g food ww; see section 2.3.1). After adaptation to the test diet, feeding was suspended for two days to ensure that the guts were completely empty at the start of the experiment. Furthermore, prior to exposure, all tanks were cleaned to remove faeces and the wet weight of all fish was re-determined. From the first experimental day (= day 0) fish were fed control food or food spiked with CuO NPs or CuCl2 for up to one week. The dietborne Cu dose was 2 μg Cu per g fish ww per day, based on the following considerations: three-spined sticklebacks reach satiation after ingesting ∼5% of their body weight.35 50 μg Cu per g ww food can be considered as the maximum Cu concentration that can be expected in foraging organisms of stickleback in metal-polluted streams.36–38 Furthermore, a dietary dose of 2 μg Cu per g fish ww per day approximately corresponds to the dose(s) for which chronic toxicity can be observed in the most sensitive fish species,39 but no acute toxicity is expected. During exposure, it was verified that each fish consumed its entire daily ration, and faeces were removed and collected in separate polypropylene tubes for later Cu analysis.
After 1, 3 and 7 days of exposure, at least three fish of each treatment group were sampled. Following measurement of their body ww, fish were killed with a sharp blow to the head followed by spinal transection and the total fish length was determined. The fish's body cavity was opened, and the gastrointestinal tract and liver were excised. The stomach was abscised from the intestine, emptied of chyme and placed back into the carcass. The wet weight of intestines and liver was determined, and intestines were flushed with phosphate buffer to remove chyme/faeces. ∼5 mm long fragments were abscised from the liver and the proximal end of the intestine for gene expression analysis. All samples were snap-frozen and stored in liquid nitrogen. The remaining larger parts of the organs were analysed for Cu by ICP-MS.
During exposures, DO, pH, temperature and conductivity were measured approximately once a day. All parameters were within the quality criteria and no significant differences were observed among aquaria/treatments (DO: control 85.1 ± 7.4%, CuCl2 84.9 ± 7.3%, CuO NP 85.1 ± 7.6%; pH: control 8.77 ± 0.28, CuCl2 8.77 ± 0.30, CuO NP 8.80 ± 0.29; temperature: control 14.0 ± 0.2 °C, CuCl2 14.0 ± 0.2 °C, CuO NP 13.9 ± 0.3 °C; conduct.: control 152.8 ± 0.6 μS cm−1, CuCl2 152.7 ± 0.6 μS cm−1, CuO NP 152.4 ± 1.2 μS cm−1). All fish were treated in accordance with the ethical practices defined by the Swedish Board of Agriculture (ethical permit number 220-2013).
1.6.4. Gene expression analysis in three-spined stickleback.
Organ samples were homogenized using stainless steel beads (5 mm, Qiagen) and a TissueLyser II apparatus (Qiagen) according to the manufacturer's instructions. Total RNA was isolated using an RNeasy Plus Mini Kit (Qiagen). RNA quantity and purity were verified on a NanoDrop 2000c spectrophotometer (ThermoFisher). RNA was transcribed into cDNA using an iScript™ cDNA synthesis kit from BioRad. Quantification of relative mRNA expression levels (cDNA copy numbers) of the reference genes β-actin (β-act), ubiquitin (ubq) and 18S ribosomal RNA (18s) and the target genes zona occludens protein-1 (zo-1), high affinity copper transporter (ctr1), metallothionein-A (mta), glutamate-cysteine-ligase, catalytic subunit (gcl), glutathione reductase (gr), glutathione peroxidase (gpx), cytosolic superoxide dismutase (sod-1) and catalase (cat) was carried out using SsoAdvanced™ Universal SYBR Green Supermix from BioRad and appropriate primer pairs obtained from the literature or designed using NCBI PrimerBLAST and genome sequence/gene annotation information available at the Ensemble genome browser (https://www.ensembl.org/Gasterosteus_aculeatus/Info/Annotation; accessed September 2017). Designed primer pairs either span the exon–intron boundary or flank large introns. The sequence of the oligonucleotide primers, their concentration, and the efficiencies of the respective qPCR assays are shown in Table S3.† All qPCR reactions were run on a Bio-Rad CFX Connect™ Real-Time System programmed to conduct one 3 min cycle at 95 °C followed by 40 cycles of 10 s at 95 °C and 30 s at 60 °C or 62 °C. All reactions were run in duplicate. ΔCq values were calculated by subtracting the mean Cq value of each target gene from the average of the mean Cq values of all three reference genes.
1.7. ICP-MS analysis
The total Cu content of fish and worm tissues, sediment, water, food packages and fish faeces were measured by ICP-MS (Agilent 7900 ICP-MS). Samples were dried in an oven at 40 °C for >24 h. Fish carcass samples were weighed in Weflon vials and dissolved with 65% ultrapure nitric acid (HNO3) and MQ water (1
:
1) in a volume of 6.25 mL. All other samples were weighed in Teflon inserts, which was possible due to the smaller size of these samples. Samples were dissolved in HNO3 and MQ water (1
:
1) similar to fish carcass samples but in smaller volumes (0.625 mL). Three Teflon inserts were placed in each Weflon vial containing 10 mL MQ water and 2 mL 30% H2O2. All samples were subsequently digested according to ISO15587-2. Briefly, samples were heated in a microwave oven (Milestone Start D microwave digestion system, Leutkirch, DE) at 250, 400, 650, and 250 W for 6 min, at each power setting. All samples were then transferred into volumetric flasks, resulting in a known volume (50 mL for fish carcasses and 5 or 10 mL for all other samples) resulting in 8% HNO3. Finally, the total Cu content in each sample was determined directly after digestion or after a short storage period (<48 h). A series of standard Cu solutions (8% HNO3) were used to calibrate Cu concentrations (0.1, 1, 10, 100 and 1000 μg Cu per L). Cu recovery was examined using certified lobster hepatopancreas (LUTS-1, National Research Council of Canada), and certified freshwater sediment (RIZA, Trace metals WD), which were digested and analysed together with different sample batches. Results were in good agreement with the certificate of analysis. All equipment was thoroughly acid-washed (including soaking in an acid bath for >24 h) before use. Samples from dissolution and leaching experiments were analysed using ICP-MS (Perkin Elmer, NexION 350D). Samples were acidified by adding trace metal-grade 65% HNO3 and subsequently diluting with MQ water, bringing the final acid concentration of the sample to 2% HNO3. The recovery was tested using CuO nanoparticles (40–50 nm, PlasmaChem GmbH, DE) and resulted in 98.5 ± 6.7% recovery, validating the sample preparation method. All glassware used during sample processing was acid washed with HNO3.
1.8. Statistical analysis
Data are presented as mean ± SD (standard deviation of the mean) throughout unless otherwise stated. Statistical comparisons of target gene expression levels between treatments were carried out on ΔCq values using one-way analysis of variance (one-way ANOVA) when data met the test's requirements for normality and homoscedasticity, or Kruskal–Wallis one-way analysis of variance on ranks when the latter was not the case. By means of these tests no statistically significant differences were detected between treatment groups. Therefore, no further post hoc tests were conducted. In addition, t-tests were performed to separately compare means of two of the three treatment groups (control vs. CuCl2, control vs. CuO NP, CuCl2vs. CuO NP). The number of independent samples (n) was at least 3 (except for the control from day 7, where it was only 2). The statistical software used was SigmaPlot for Windows Version 13.0 (Systat Software, Inc.). For the graphical presentation of the qPCR results the fold change (FC) in target gene expression levels with respect to the control was calculated as FC = 2−ΔΔCq, where ΔΔCq = ΔCq (control) − ΔCq (treatment). The corresponding upper and lower bound were calculated as FCmax = 2−ΔΔCq−SD and FCmin = 2−ΔΔCq+SD, respectively. The strength of the association between pairs of variables (for instance, Cu concentration and mRNA expression level) was measured by Pearson product–moment correlation analysis using SigmaPlot for Windows Version 13.0 (Systat Software, Inc., San Jose, CA, USA).
2. Results and discussion
2.1. Characterisation of CuO NPs before and after incorporation into the food packages
Characterization was carried out in both the stock dispersion used for spiking T. tubifex homogenates and the food packages. TEM images of the stock dispersion revealed that the CuO NPs were of spheroidal-ellipse, polygonal and rod-like shape (Fig. 1A and B), had a mean AR of ∼1.6 ± 1.2, and a mean minimum and maximum caliper diameter (Feret diameter) of 51.9 ± 25.1 nm and 79.1 ± 57.9 nm, respectively (n = 400). The AR and size-frequency distributions are shown in Fig. 1 as well as in Fig. S1 and Table S1 in the ESI.† Large and compact CuO NP agglomerates/aggregates were frequently observed in the TEM images but were probably in part drying artefacts produced during sample preparation.40 In addition, smaller agglomerates/aggregates and single NPs were identified.
 |
| Fig. 1 Characterisation of CuO NPs in the stock dispersion. (A and B) TEM micrographs of the aqueous CuO NP stock dispersion (1 mg Cu mL−1) diluted 1 : 100 in MQ water and drop-casted onto carbon-coated copper grids. Scale bars in (A) and (B) correspond to 200 nm. (C and D) Primary particle size-frequency distribution based on the NPs' minimum caliper diameter (n = 400) and corresponding AR-frequency distribution. (E) Hydrodynamic size distribution of a 10 μg mL−1 dilution (1 : 100) of the sonicated CuO NP stock dispersion in MQ used for spiking of worm homogenate for the preparation of NP-containing food packages. Furthermore, the corresponding descriptive variables including z-average, polydispersity index (PdI), mean size (diameter) and intensity of the individual peaks are displayed (table right of the graph). | |
DLS analysis performed on the stock dispersion revealed the presence of at least three distinct particle size populations. The size intensity distribution including the mean diameter of the intensity peaks is shown together with the z-average and polydispersity index (PdI) in Fig. 1E. The zeta-potential of the CuO NPs in the stock dispersion was −16.5 ± 6.3 mV indicating that the tested CuO NPs were relatively unstable in suspension.
While data on the NP characteristics in the stock dispersion is of outmost importance for reasons of study reproducibility and inter-study comparisons, its informative value regarding the actual exposure conditions is limited. Therefore, TEM imaging was conducted to obtain additional information on NP size and shape once embedded in the gelatinous food matrix. The images showed – besides cellular debris including lipid droplets and cellular organelles originating from the homogenized T. tubifex tissue – that CuO NPs were present both in form of single particles (∼50–100 nm) (cp. single NP size distribution in the stock dispersion used for food spiking, Fig. 1C) as well as agglomerates with sizes up to a few hundred nanometres (Fig. S4, images B and C†).
Possibilities for NP characterisation in complex environmental matrices including sediment systems are limited due to lack of appropriate analytical methods.41,42 Therefore, we characterised the NPs before spiking them into the sediment (Fig. 1, S1 and Table S1†).
2.2. Transfer of dissolved Cu and CuO NPs from sediment to T. tubifex
2.2.1. Cu accumulation in T. tubifex.
In the first experiment, where accumulation of sediment-associated CuCl2 and CuO NP in T. tubifex was examined, the spiked sediment concentrations were significantly higher than in the control sediment and did not differ significantly between the CuCl2 and CuO NP treatments (Fig. 2). After one week of exposure, the weight specific body burden (WSBB) of Cu in T. tubifex did not differ significantly among the three treatments (CuO NPs, CuCl2 and control) (p = 0.229; Fig. 2). Thus, we argue that it was not possible to distinguish newly accumulated Cu from background levels due to a high background concentration and large variation in Cu levels in T. tubifex. However, our initial studies showed a significant increase in body burden of isotopically labelled 65Cu in T. tubifex compared to control worms after 7 days' exposure to sediment amended with 65CuO NPs or 65CuCl2 at environmentally realistic Cu sediment concentrations (30 and 40 μg 65Cu per g dw sediment). Specifically, the increase in total Cu body burden in the CuCl2 and CuO NP treatment was ∼3.5 and 3 μg Cu per g dw, respectively (unpublished results).
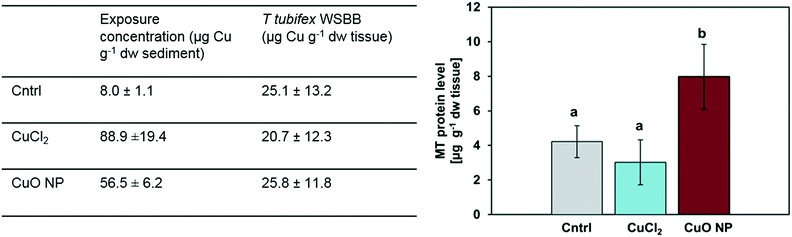 |
| Fig. 2 Sediment exposure concentrations, T. tubifex WSBB (weight specific body burden), and MT (metallothionein) protein level after seven days of exposure to clean sediment (control, above abbreviated as “cntrl”) or sediment spiked with CuCl2 or CuO NPs, presented as mean ± SD; n = 6. WSBBs of T. tubifex exposed to clean, CuCl2- and CuO NP-spiked sediment were not significantly different (table on the left). Statistically significant differences in MT protein levels are indicated by different letters (figure on the right). | |
Thus, the use of isotopically labelled Cu will make it possible to discriminate between background Cu and newly accumulated Cu and hence detect Cu accumulation at low levels. Accordingly, previous studies with isotopically labelled 65CuCl2 and 65CuO NPs have showed a significant increase in 65Cu compared to control worms after sediment exposure of L. variegatus8,43 and T. tubifex (our unpublished results). These results suggest that both dissolved and nanoparticulate Cu is available for uptake and accumulate in T. tubifex and suggest that the extent of Cu accumulation is largely independent of the Cu form (dissolved vs. nanoparticulate) present in the sediment. However, more information about the accumulation kinetics of CuO NPs and dissolved counterparts in sediment is needed. This is consistent with previous results obtained in the endobenthic worms N. diversicolor, in which slightly higher, albeit not significantly different, Cu levels were measured following sediment exposure to dissolved Cu compared to nanoparticulate Cu.2,8,9
2.2.2. Induction of metallothionein-like proteins in T. tubifex.
Interestingly, DPP analysis demonstrated that MTLP levels in T. tubifex exposed to CuCl2-spiked sediment were comparable to those measured in the control, but MTLP levels in worms exposed to CuO NP-spiked sediment were significantly increased compared to both the CuCl2 and the control treatment (Fig. 2). This suggests that there might be differences in the biological response, even though the WSBBs of Cu are comparable.
The absence of a positive correlation between MTLP levels and WSBB in the CuCl2-exposure may seem counterintuitive but is consistent with previous observations in the scientific literature, for instance in N. diversicolor.44,45 Considering that the determined MTLP levels reflect the net balance of MTLP synthesis and breakdown at a snapshot in time, different possible explanations exist for such observation.46 First, it has been proposed that increased activity of MTLP-mediated metal detoxification may not always be reflected by an elevated intracellular MTLP concentration, but by an increased MTLP turnover rate.44,45 The half-life of MTLPs in T. tubifex is not known, but for some aquatic invertebrates including worms the half-life of MTLPs was reported to be only 1–2 days,44,46 which would render the above explanation also plausible for a short-term exposure scenario, such as was the case in our study. An alternative explanation for a lack of increase in MTLP levels in the CuCl2-exposed T. tubifex compared to the control could be an overall decreased biosynthetic capacity due to sublethal effects induced by dissolved Cu species.47 Although CuO NPs may partly dissolve in presence of strong ligands,48 worms in the NP-spiked sediment were probably not exposed to as high concentrations of dissolved Cu species as in the CuCl2 treatment, or if so, then exposure to high concentrations occurred delayed in time, i.e. for a shorter period of time, which is likely not to cause the same effect. Further research on Cu/CuO NP fate and transformation in sediment, gut lumen and tissue, especially Cu form-dependent differences in chemical speciation, relative significance of dermal and dietary exposure route, and cellular/organismal uptake, transport, storage and detoxification processes is needed to acquire a deeper mechanistic understanding of the observed differences in MTLP levels and is planned for future studies.
2.3. Preparation and performance of worm-shaped food packages
Exposure of worms to dissolved and nanoparticulate metals in sediment often results in high variability in worm metal WSBB, both within and between treatment groups. Thus, assessing the further transfer of dissolved and nanoparticulate metals to higher trophic level organisms, such as fish, in a comparative manner, i.e. using equivalent exposure concentrations, is challenging when feeding pre-exposed worms. To circumvent confounding factors of variation in WSBB in fish food and consequently exposure dose, CuO NP-spiked food packages prepared from T. tubifex homogenate were used as an alternative experimental approach (proof of concept). The Cu concentration in CuCl2- and CuO NP-containing food packages was similar (36.9 and 36.2 μg g−1 food ww, respectively), demonstrating that the spiking of the worm homogenate could be equally well performed regardless of Cu form. The Cu concentration in the control food was 1.4 μg g−1 food ww. Furthermore, the length of the worm-shaped food packages could be adjusted with high accuracy (±0.5 mm; see Fig. S4†) resulting in a maximum deviation from the nominal food ration of ∼0.5 mg, corresponding to a deviation of ∼25 ng Cu or 1.25% from the nominal daily dietary exposure dose (2 μg Cu per g fish ww per day) (calculating with a food density of ∼0.7 mg mm−3, a nominal Cu concentration of 50 μg g−1 ww food and a fish body ww of 1 g). In addition, although preliminary tests revealed inter-individual differences in the efficiency with which clean food packages were consumed, approximately 90% of the fish selected for the experiment ingested the food packages within a few seconds after addition to the aquarium (food packages were generally swallowed in one piece and no visible leftovers or disgorged food debris remained in the water). In the rest of the cases (4 out of 36 fish) food packages remained lying on the bottom of the tank for approximately 15 min before being consumed.
Cu leaching tests showed that the Cu concentration in the “water column” after 30 min of incubation was 0.009, 0.109 and 0.010 mg L−1 for control, CuCl2- and CuO NP-spiked food packages, respectively. Thus, some Cu leaching from CuCl2-containing food packages into the water was detected. However, this leaching rate would result in very low waterborne Cu concentrations in the exposure tanks: the water volume in these tanks was 500 times larger (1.5 L) than that in the leaching experiment, which together with the high water turnover will decrease the likelihood of any unintentional waterborne Cu exposure.
All together these data demonstrate that the developed food packages are a suitable tool enabling highly controlled dietary NP exposure of small fish, which has an enormous potential for other contaminants as well.
2.4. Trophic transfer of Cu from dissolved Cu and CuO NPs to three-spined stickleback
2.4.1. Dissolution of CuO NPs at gastric pH.
The Cu concentration in ultrafiltrates of 50 μg mL−1 CuO NP dispersions prepared in simulated gastric fluid (SGF, pH 4) was considerably higher than in MQ water (pH 7) after incubation at RT for 7 h (which is the estimated gastric half-life in stickleback32) (Table 1). This finding is consistent with the literature reporting a dissolution minimum at neutral pH and a significant increase when reaching pH values of 4–5.48 However, overall dissolution was low (4% in SGF and <0.1% in MQ water), which was also confirmed by the unaltered particle morphology (Fig. S3;† compare with Fig. 1A and B). These results suggest that some CuO NP dissolution is likely to occur in the stomach, but it may be rather low, and most of the nanomaterial reached the intestine in particulate form. Yet, additional experiments in the presence of organic ligands in the food, such as metal-chelating amino acids, need to be conducted to obtain a more realistic quantitative estimate of CuO NP dissolution during gastrointestinal passage.
Table 1 CuO NP dissolution after prolonged incubation time and at gastric pH
Stock dispersions (50 μg Cu per mL) |
Dissolved Cu [μg mL−1] |
t = 0 |
t = 7 h |
CuCl2 (recovery control), pH = 7 |
53.130 |
N.A. |
CuO NP in MQ, pH = 7 |
0.070 |
0.047 |
CuO NP in SGF, pH = 4 |
N.A. |
1.902 |
2.4.2. Cu accumulation and tissue distribution in stickleback.
Dietary exposure to CuCl2 and CuO NPs for 7 days at a daily dose of 2 μg Cu per g ww fish resulted in an increase in the total Cu content of intestinal tissue of the three-spined sticklebacks (Fig. 3). The average Cu concentration in intestinal tissue of fish fed control, CuCl2- and CuO NP-spiked food packages during seven days was ∼12, 42 and 24 μg Cu per g dw tissue, corresponding to an increase of ∼1, 32 and 13 μg Cu per g dw tissue compared to background levels (= day 0), respectively (Fig. 3A). Wang et al. (2014) reported higher intestinal Cu concentrations after exposure to nanoparticulate Cu compared to CuSO4 in juveniles of the marine fish Epinephelus coioides.49 However, in their study a waterborne exposure scenario was used, which is likely to result in a different accumulation pattern.
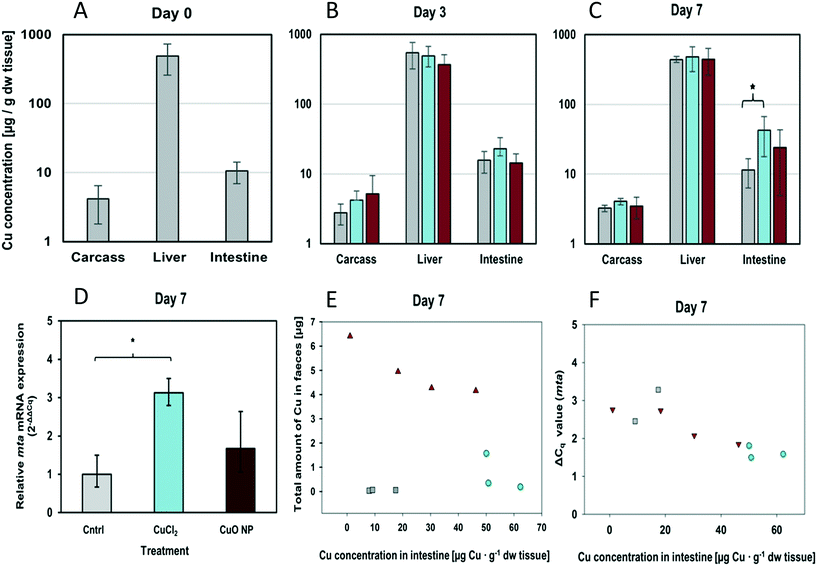 |
| Fig. 3 Cu uptake, tissue distribution, egestion and metallothionein induction in three-spined sticklebacks upon dietary exposure to control (cntrl), CuCl2 and CuO NPs. Cu concentration in carcass, liver and intestine of unexposed fish (day 0) (A), after three days (B), and after seven days (C) of dietary exposure to spiked food packages presented as mean and SD (n = 3). (D) Relative mta mRNA expression levels in intestinal tissue after seven days of exposure. Bars show the mean fold change with respect to the negative control (cntrl). Error bars show the lower and upper bound calculated from the SD of ΔΔCq values (n ≥ 3). Asterisks (*) indicate statistically significant differences between treatment groups. (E) Multiple scatter plots showing an inverse correlation of Cu concentrations in intestinal tissue and faeces of three-spined sticklebacks fed spiked food packages for seven days. Note the treatment-dependent clustering of data pairs (■ = control, ● = CuCl2, ▲ = CuO NP). (F) Multiple scatter plots showing a positive correlation between Cu concentration and mta mRNA expression in stickleback intestine (■ = control, ● = CuCl2, ▲ = CuO NP). Note: the higher the ΔCq value, the lower the amount of mRNA. Asterisks (*) indicate statistically significant differences between treatment groups. | |
The hepatic Cu concentrations measured in fish exposed to control, CuCl2- and CuO NP-spiked food packages for 7 days were not significantly different (442 ± 44, 481 ± 186 and 448 ± 187 μg Cu per g dw tissue; mean ± SD, n = 3, respectively). Furthermore, there was no evidence for accumulation in liver over time. The average hepatic Cu concentration at day 0 was 494 ± 236 μg Cu per g dw tissue, which is within the range of hepatic Cu concentrations reported in the published literature, though relatively high considering that the fish originated from a pristine site. For instance, Bervoets et al. (2001) measured concentrations of around 50 μg Cu per g liver dw in sticklebacks caught from Belgian river basins,50 and Roussel et al. (2007) reported Cu concentrations as high as ∼75–340 μg Cu per g liver ww in three-spined sticklebacks from unpolluted streams.51 High Cu levels in the liver are in agreement with the central role the organ plays in Cu metabolism including supply, storage and clearance in fish.52 Also, for the carcass samples no significant differences between treatments or sampling time points were observed.
Overall, these results suggest that Cu from both CuCl2- and CuO NP-containing food packages was available and taken up into intestinal tissue. However, further transport to internal organs and systemic accumulation was limited, that is, the capacity of the stickleback's intestine to buffer the temporary increase in dietary Cu absorption was not exceeded. This is in agreement with previous studies in salmonids showing that dietary Cu exposure needs to surpass a threshold level (typically several ten- to hundred-fold higher compared to normal dietary Cu levels) before hepatic Cu accumulation occurs.53–56
2.4.3. CuO NP egestion and uptake efficiency.
The faeces egested by the three-spined sticklebacks did not disintegrate in aquarium water and could be collected from each individual aquarium daily (Fig. S2†). The total amount of Cu measured in the faeces from fish fed CuO NP- and CuCl2-contaminated food packages was 4.94 ± 0.99 μg and 1.19 ± 0.90 μg corresponding to 25.3 ± 7.4% and 8.1 ± 10.6% of the totally administered/ingested Cu, respectively (Error! Reference source not found.). This would suggest that ∼75% and >90% of Cu from CuO NP-food and CuCl2-food, respectively, were taken up via the gastrointestinal tract. Although previous studies showed that the net Cu absorption in the intestine can be up to ∼50% of the amount of ingested Cu,57 dietary uptake efficiencies of such a magnitude seem unrealistic. It is known that a significant amount of dietborne Cu is excreted with the mucus and through sloughing of intestinal cells, in which the metal has accumulated.39 Possibly the chyme/faeces comprised a physically less stable fraction or fluid phase, which contained part of the ingested, not absorbed Cu, and which was egested without being noted and/or without that it could be collected.
The relative uptake efficiencies of CuO NP and CuCl2 were not determined in this study, but comparison of Cu concentrations in faeces showed that three- to nine- times more Cu was egested by fish fed with CuO NP-spiked diet than by fish fed with CuCl2-spiked diet (Table S4†). Furthermore, there was a strong negative and statistically significant correlation between the amount of Cu egested by individual fish and their intestinal Cu concentration (Pearson's r: −0.91; p = 0.005, n = 7) (Fig. 3). These results demonstrate that Cu uptake from the food containing a nanoparticulate Cu source was lower than that from the food where Cu was present in an ionic or complexed form. However, which influence the use of an artificial diet has compared to a more realistic exposure scenario needs to be examined, for instance when using pre-exposed live worms as prey/diet, which will be the focus of our future studies.
The literature on the dietary uptake of nanoparticulate Cu in fish is scarce. To the best of our knowledge, only two studies have been published and both within the field of aquaculture. However, these studies lack NP characterization data, making a comparison difficult.58,59 The results obtained by Wang et al. (2018) suggest that the bioavailability of nanoparticulate CuO (40 nm) was up to two times higher compared to that of CuSO4.59 El Basuini et al. (2016), on the contrary, did not observe any differences in Cu accumulation between fish (red sea bream) receiving a diet containing Cu powder or CuSO4.58 However, although the authors use the term “nanopowder”, the size of the original Cu particles added to the food was <75 μm (manufacturer information) and the relative significance of the nanoparticulate fraction cannot be evaluated in the diet used in their study.
2.4.4. Genetic response to dietary CuCl2 and CuO NP exposure.
2.4.4.1. Changes in hepatic gene expression.
Hepatic mta mRNA expression levels tended to increase with the Cu tissue concentration (Pearson's r of [Cu] vs. ΔCqmta = −0.342), but there was no statistically significant relationship between the two variables (p = 0.333) (Table S5†). Similarly, no significant correlation with the hepatic Cu content was found for the other variables (ΔCqctr1, ΔCqgcl, ΔCqgr, ΔCqsod-1, ΔCqcat and ΔCqgpx). The data are further described in the ESI.† The absence of Cu-dependent effects in liver may be explained by the low, environmentally realistic dietary exposure dose in our study (2 μg Cu per g fish ww per day). Excess dietary Cu is expected to be sequestered in the intestinal tissue and only be transported to other internal organs when the intestinal storage capacity is surpassed.39,52
2.4.4.2. Changes in intestinal gene expression.
Alterations in gene expression levels were observed in the intestine. In fish receiving CuCl2-spiked food packages for seven days, mRNA expression levels of zo-1, ctr1, mta, gpx and sod-1 were on average ∼1.4-, 1.3-, 2.9-, 1.5- and 1.2-fold elevated compared to control fish (Fig. 3D and Table 2). The increase in mta mRNA expression was statistically significant (p = 0.033, t-test), showing the induction of adaptive responses to countervail excess intracellular Cu. The effect of CuO NPs on intestinal gene expression was generally weaker than that observed for CuCl2. CuO NP exposure resulted in a noticeable, but not statistically significant, increase in mta mRNA expression levels (1.6 times higher with respect to the control) (Fig. 3D). These results are congruent with the Cu concentration in intestine at day 7 (Fig. 3C). Furthermore, Pearson product moment correlation analysis demonstrated a strong negative correlation between the intestinal Cu concentration and the ΔCq values for mta measured in each individual fish (Pearson's r = −0.851; p = 0.0018) (note: the higher the ΔCq value, the lower the amount of mRNA) (Table S5†). In addition, fish-specific xy-pairs ([Cu] vs. ΔCqmta) were observed to cluster in a treatment-specific manner (Fig. 3F). The strong correlation between exposure and effect level reflects the success of the individual-specific dosing procedure in reducing data variability. This makes it possible to work with a low replicate number. Furthermore, it demonstrates that mta induction was a direct consequence of dietary Cu exposure and that the observed differences between treatments were not due to random sampling variability or other external factors (e.g. other metals in the food or stress). The strong correlation between intestinal [Cu] and mta expression strongly suggests that the Cu, which has accumulated in the intestinal tissue of CuO NP-exposed stickleback, was intracellularly present in a form that can be sensed by and activate metalloregulatory transcription factors, that is, as dissolved Cu. Since CuO NP dissolution in the sonicated stock dispersion was minimal (0.020 μg mL−1 dissolved Cu, or ≤0.002%) the most plausible explanation is that CuO NPs partly dissolved in the gastrointestinal tract. This interpretation is substantiated by our data showing minimal yet enhanced NP dissolution at gastric pH 4.
Table 2 Relative mRNA expression levels of selected target genes in the intestine of three-spined stickleback upon seven days of dietary exposure to CuCl2 and CuO NPs. Values in bold represent the mean fold change (FC) with respect to the negative control (cntrl). Values in square brackets indicate the deviations from the mean FC in both directions, which were calculated from the SD of ΔΔCq values (n ≥ 3). No statistically significant differences were observed between treatment groups
Target gene |
Fold change |
Cntrl |
CuCl2 |
CuO NP |
zo-1
|
1.00 [−0.10/+0.11] |
1.41 [−0.44/+0.63] |
1.03 [−0.27/+0.38] |
ctr1
|
1.00 [−0.04/+0.04] |
1.34 [−0.26/+0.32] |
0.79 [−0.38/+0.74] |
gcl
|
1.00 [−0.21/+0.26] |
1.00 [−0.24/+0.31] |
1.04 [−0.14/+0.16] |
gr
|
1.00 [−0.49/+0.95] |
1.17 [−0.69/+1.68] |
1.11 [−0.88/+4.22] |
gpx
|
1.00 [−0.21/+0.26] |
1.48 [−0.29/+0.37] |
0.87 [−0.38/+0.68] |
sod-1
|
1.00 [−0.12/+0.13] |
1.16 [−0.21/+0.26] |
1.36 [−0.27/+0.35] |
cat
|
1.00 [−0.04/+0.04] |
0.51 [−0.36/+1.25] |
0.89 [−0.28/+0.40] |
There is increasing evidence that Cu exposure can compromise epithelial tight junctions and increase the permeability of the intestinal mucosal barrier. Tight junction function was shown to be impaired upon short exposure (60–180 min) to 50 μmol L−1 (∼0.32 μg mL−1),60 which corresponds to the concentration the sticklebacks' intestines were exposed to in our study. Indeed, we observed elevated transcript levels of the tight junction protein encoding gene zo-1, but only in fish fed CuCl2-spiked food packages (Table 2). The increase was not statistically significant with respect to the control (p > 0.05; one-way ANOVA and t-test on ΔCq-value means), but transcript levels strongly correlated with the intestinal Cu concentrations (Pearson's r = −0.718, p = 0.0195) (Table S5†). Previous studies reported increased transcription of tight junction protein encoding genes concomitant with or following tight junction disruption,61,62 and a relation between the degree of tight junction disruption and intracellular Cu concentration.61 Therefore, the observed upregulation of zo-1 transcription in stickleback intestine may be indicative of an adaptive response to Cu-induced tight junction disruption and represent the initiation of compensatory tight junction protein synthesis aiming at maintaining/restoring intestinal barrier function. There is a discussion on whether tight junction disruption may be a consequence of Cu-induced oxidative stress. We observed that gpx mRNA levels were 1.5-fold elevated in the CuCl2 treatment compared to control fish, which may be indicative of an increased need to cope with peroxidised biomolecules, such as lipids. However, as for the other oxidative stress-related genes (sod-1, gcl, gr and cat), the observed up-/downregulation was not statistically significant (p > 0.05; one-way ANOVA) (Table 2). Further studies are needed to examine the effect of Cu/CuO on tight junction function and to identify the mechanisms involved. Yet, our data give rise to some intriguing speculations, for example, whether dissolved Cu could act as a “gate opener” for CuO NPs enabling their paracellular passage across the epithelial mucosal barrier. Overall, however, the obtained data suggest that the effects of dietary exposure to environmentally relevant Cu concentrations are minimal – especially when Cu is present in particulate form.
3. Conclusions and perspectives
The current knowledge and understanding of dietary uptake and bioaccumulation of metal/metal oxide NPs in aquatic organisms is limited. Even less is known on the fate of metal/metal oxide NPs within food webs. Important questions in this context are if and how uptake, bioaccumulation and trophic transfer of metals/metal oxides differ depending on whether they are present in dissolved or nanoparticulate form. Satisfactory answers to these questions can only be obtained by collaborative interdisciplinary, systematic research efforts that integrate different biological model systems and analytical methodologies.
Here we present the results of the first of a series of studies carried out within the NanoTransfer project, which aims at examining the trophic transfer of Cu-based NPs from the sediment compartment to the endobenthic worm T. tubifex to the predatory fish three-spined stickleback (G. aculeatus). The experimental strategy adopted in this study comprised two independent exposure experiments: 1) an exposure of T. tubifex to CuCl2 or CuO NP-spiked sediment and 2) a dietary exposure of G. aculeatus to CuCl2- or CuO NP-spiked food packages prepared from T. tubifex homogenate, together simulating Cu/CuO NP transfer from sediment to worm to fish. The originality of the second experiment lies in the production and use of food packages prepared from NP-spiked worm homogenates, which allowed one to obtain information on the NP characteristics after their incorporation into the test diet, enabled equivalent Cu dosing in the CuO NP and dissolved Cu treatment, and provided maximum control of the dietary dose each fish received. The food packages not only proved to be a valuable tool to examine NP transfer from worm to fish, but will also be useful for future kinetic uptake/bioaccumulation studies with CuO NPs and/or other contaminants, in which maximum control of the exposure route and dose is desirable.
The evidence available from this proof-of-concept study suggests that both dissolved and nanoparticulate Cu may be transferred from sediment-dwelling worms to higher trophic level organisms, such as fish. Our data show that CuO NPs partly dissolve in simulated gastric fluid, suggesting that food type-dependent parameters including Cu/CuO NP speciation, gastric residence time, co-existing ligands, stomach pH and fish species-dependent differences in digestive physiology are factors that deserve careful consideration. All these factors may determine the degree of NP dissolution and which Cu form (dissolved or nanoparticulate, large or small particle size etc.) absorptive epithelia will eventually be exposed to. Our results suggest that only the dissolved Cu fraction may be taken up and sequestered in the intestinal tissue of sticklebacks and that a large part of the particulate fraction is egested with the faeces. Trophic transfer of CuO NPs from Tubifex to sticklebacks may hence be limited. Furthermore, the results obtained in this study suggest that dietary exposure of fish to environmentally relevant concentrations results in no or only minimal effects – especially when Cu is present in particulate form. Yet, NPs may undergo transformation during their passage through the gastrointestinal system and NPs with a different physico-chemical identity will thus re-enter the abiotic compartment and be available again for uptake by third organisms. Our future work will concentrate on determining how NP transformation by chemical and biological processes in sediment, food/prey and predator influences Cu/CuO NP bioavailability, uptake, internal fate, accumulation, trophic transfer and biological effects. An enhanced and mechanistic understanding of these processes will increase environmental realism in the environmental safety evaluation of metal NPs.
Conflicts of interest
There are no conflicts to declare.
Acknowledgements
This research was financed by the Villum Foundation (grant reference number: 00010592). The authors acknowledge the Centre for Cellular Imaging at the University of Gothenburg and the National Microscopy Infrastructure, NMI (VR-RFI 2016-00968), for providing assistance in microscopy, as well as Dr. Aiga Mackevica (DTU) and Dr. Messika Revel (UCO) for their help with ICP-MS and DPP measurements.
Notes and references
- L. M. Skjolding, S. N. Sorensen, N. B. Hartmann, R. Hjorth, S. F. Hansen and A. Baun, Aquatic Ecotoxicity Testing of NanoparticlesThe Quest To Disclose Nanoparticle Effects, Angew. Chem., Int. Ed., 2016, 55, 15224–15239 CrossRef CAS PubMed.
- A. Thit, A. Dybowska, C. Kobler, G. Kennaway and H. Selck, Influence of copper oxide nanoparticle shape on bioaccumulation, cellular internalization and effects in the estuarine sediment-dwelling polychaete, Nereis diversicolor, Mar. Environ. Res., 2015, 111, 89–98 CrossRef CAS PubMed.
- T. Gomes, J. P. Pinheiro, I. Cancio, C. G. Pereira, C. Cardoso and M. J. Bebianno, Effects of Copper Nanoparticles Exposure in the Mussel Mytilus galloprovincialis, Environ. Sci. Technol., 2011, 45, 9356–9362 CrossRef CAS PubMed.
- A. Baun, N. B. Hartmann, K. Grieger and K. O. Kusk, Ecotoxicity of engineered nanoparticles to aquatic invertebrates: a brief review and recommendations for future toxicity testing, Ecotoxicology, 2008, 17, 387–395 CrossRef CAS PubMed.
- S. J. Klaine, P. J. J. Alvarez, G. E. Batley, T. F. Fernandes, R. D. Handy, D. Y. Lyon, S. Mahendra, M. J. McLaughlin and J. R. Lead, Nanomaterials in the environment: Behavior, fate, bioavailability, and effects, Environ. Toxicol. Chem., 2008, 27, 1825–1851 CrossRef CAS PubMed.
-
S. Luoma and P. Rainbow, Metal Contamination in Aquatic Environments: Science and Lateral Management, Cambridge University Press, New York, 2008 Search PubMed.
- M.-N. Croteau, A. D. Dybowska, S. N. Luoma and E. Valsami-Jones, A novel approach reveals that zinc oxide nanoparticles are bioavailable and toxic after dietary exposures, Nanotoxicology, 2011, 5, 79–90 CrossRef CAS PubMed.
- A. Thit, T. Ramskov, M. N. Croteau and H. Selck, Biodynamics of copper oxide nanoparticles and copper ions in an oligochaete - Part II: Subcellular distribution following sediment exposure, Aquat. Toxicol., 2016, 180, 25–35 CrossRef CAS PubMed.
- A. Thit, G. T. Banta and H. Selck, Bioaccumulation, subcellular distribution and toxicity of sediment-associated copper in the ragworm Nereis diversicolor: The relative importance of aqueous copper, copper oxide nanoparticles and microparticles, Environ. Pollut., 2015, 202, 50–57 CrossRef CAS PubMed.
- H. Selck, R. D. Handy, T. F. Fernandes, S. J. Klaine and E. J. Petersen, Nanomaterials in the Aquatic Environment: A European Union-United States Perspective on the Status of Ecotoxicity Testing, Research Priorities, and Challenges Ahead, Environ. Toxicol. Chem., 2016, 35, 1055–1067 CrossRef CAS PubMed.
- C. Mouneyrac, P. E. Buffet, L. Poirier, A. Zalouk-Vergnoux, M. Guibbolini, C. Risso-de Faverney, D. Gilliland, D. Berhanu, A. Dybowska, A. Chatel, H. Perrein-Ettajni, J. F. Pan, H. Thomas-Guyon, P. Reip and E. Valsami-Jones, Fate and effects of metal-based nanoparticles in two marine invertebrates, the bivalve mollusc Scrobicularia plana and the annelid polychaete Hediste diversicolor, Environ. Sci. Pollut. Res., 2014, 21, 7899–7912 CrossRef CAS PubMed.
- P. E. Buffet, J. F. Pan, L. Poirier, C. Amiard-Triquet, J. C. Amiard, P. Gaudin, C. Risso-de Faverney, M. Guibbolini, D. Gilliland, E. Valsami-Jones and C. Mouneyrac, Biochemical and behavioural responses of the endobenthic bivalve Scrobicularia plana to silver nanoparticles in seawater and microalgal food, Ecotoxicol. Environ. Saf., 2013, 89, 117–124 CrossRef CAS PubMed.
- S. R. Tangaa, H. Selck, M. Winther-Nielsen and F. R. Khan, Trophic transfer of metal-based nanoparticles in aquatic environments: a review and recommendations for future research focus, Environ. Sci.: Nano, 2016, 3, 966–981 RSC.
- A. Bour, F. Mouchet, J. Silvestre, L. Gauthier and E. Pinelli, Environmentally relevant approaches to assess nanoparticles ecotoxicity: a review, J. Hazard. Mater., 2015, 283, 764–777 CrossRef CAS PubMed.
- M. Ates, Z. Arslan, V. Demir, J. Daniels and I. O. Farah, Accumulation and toxicity of CuO and ZnO nanoparticles through waterborne and dietary exposure of goldfish (Carassius auratus), Environ. Toxicol., 2015, 30, 119–128 CrossRef CAS PubMed.
- Y. Chae and Y. J. An, Toxicity and transfer of polyvinylpyrrolidone-coated silver nanowires in an aquatic food chain consisting of algae, water fleas, and zebrafish, Aquat. Toxicol., 2016, 173, 94–104 CrossRef CAS PubMed.
- J. M. Lacave, A. Fanjul, E. Bilbao, N. Gutierrez, I. Barrio, I. Arostegui, M. P. Cajaraville and A. Orbea, Acute toxicity, bioaccumulation and effects of dietary transfer of silver from brine shrimp exposed to PVP/PEI-coated silver nanoparticles to zebrafish, Comp. Biochem. Physiol., Part C: Toxicol. Pharmacol., 2017, 199, 69–80 CAS.
- W. M. Lee and Y. J. An, Evidence of three-level trophic transfer of quantum dots in an aquatic food chain by using bioimaging, Nanotoxicology, 2015, 9, 407–412 CrossRef CAS PubMed.
- L. M. Skjolding, M. Winther-Nielsen and A. Baun, Trophic transfer of differently functionalized zinc oxide nanoparticles from crustaceans (Daphnia magna) to zebrafish (Danio rerio), Aquat. Toxicol., 2014, 157, 101–108 CrossRef CAS PubMed.
- Z. Wang, L. Yin, J. Zhao and B. Xing, Trophic transfer and accumulation of TiO2 nanoparticles from clamworm (Perinereis aibuhitensis) to juvenile turbot (Scophthalmus maximus) along a marine benthic food chain, Water Res., 2016, 95, 250–259 CrossRef CAS PubMed.
- X. Zhu, J. Wang, X. Zhang, Y. Chang and Y. Chen, Trophic transfer of TiO(2) nanoparticles from Daphnia to zebrafish in a simplified freshwater food chain, Chemosphere, 2010, 79, 928–933 CrossRef CAS PubMed.
- C. C. Piccinetti, C. Montis, M. Bonini, R. Laura, M. C. Guerrera, G. Radaelli, F. Vianello, V. Santinelli, F. Maradonna, V. Nozzi, A. Miccoli and I. Olivotto, Transfer of Silica-Coated Magnetic (Fe3O4) Nanoparticles Through Food: A Molecular and Morphological Study in Zebrafish, Zebrafish, 2014, 11, 567–579 CrossRef CAS PubMed.
- C. S. Ramsden, T. J. Smith, B. J. Shaw and R. D. Handy, Dietary exposure to titanium dioxide nanoparticles in rainbow trout, (Oncorhynchus mykiss): no effect on growth, but subtle biochemical disturbances in the brain, Ecotoxicology, 2009, 18, 939–951 CrossRef CAS PubMed.
- L. Chupani, H. Niksirat, J. Velisek, A. Stara, S. Hradilova, J. Kolarik, A. Panacek and E. Zuskova, Chronic dietary toxicity of zinc oxide nanoparticles in common carp (Cyprinus carpio L.): Tissue accumulation and physiological responses, Ecotoxicol. Environ. Saf., 2018, 147, 110–116 CrossRef CAS PubMed.
- M. Connolly, M. Fernandez, E. Conde, F. Torrent, J. M. Navas and M. L. Fernandez-Cruz, Tissue distribution of zinc and subtle oxidative stress effects after dietary administration of ZnO nanoparticles to rainbow trout, Sci. Total Environ., 2016, 551, 334–343 CrossRef PubMed.
- B. Geffroy, C. Ladhar, S. Cambier, M. Treguer-Delapierre, D. Brethes and J. P. Bourdineaud, Impact of dietary gold nanoparticles in zebrafish at very low contamination pressure: The role of size, concentration and exposure time, Nanotoxicology, 2012, 6, 144–160 CrossRef CAS PubMed.
-
OECD, Test No. 315: Bioaccumulation in Sediment-dwelling Benthic Oligochaetes, 2008 Search PubMed.
- S. R. Tangaa, H. Selck, M. Winther-Nielsen and F. R. Khan, Trophic transfer of metal-based nanoparticles in aquatic environments: a review and recommendations for future research focus, Environ. Sci.: Nano, 2016, 3, 966–981 RSC.
- I. L. Bergin and F. A. Witzmann, Nanoparticle toxicity by the gastrointestinal route: evidence and knowledge gaps, Int. J. Biomed. Nanosci. Nanotechnol., 2013, 3(1–2) DOI:10.1504/IJBNN.2013.054515.
- J. S. Taurozzi, V. A. Hackley and M. R. Wiesner, A standardised approach for the dispersion of titanium dioxide nanoparticles in biological media, Nanotoxicology, 2013, 7, 389–401 CrossRef CAS PubMed.
- T. Lammel and J. Sturve, Assessment of titanium dioxide nanoparticle toxicity in the rainbow trout (Onchorynchus mykiss) liver and
gill cell lines RTL-W1 and RTgill-W1 under particular consideration of nanoparticle stability and interference with fluorometric assays, NanoImpact, 2018, 11, 1–19 CrossRef.
- P. Kotterba, C. Kuhn, C. Hammer and P. Polte, Predation of threespine stickleback (Gasterosteus aculeatus) on the eggs of Atlantic herring (Clupea harengus) in a Baltic Sea lagoon, Limnol. Oceanogr., 2014, 59, 578–587 CrossRef.
- Y. E. Roman, K. A. C. De Schamphelaere, L. T. H. Nguyen and C. R. Janssen, Chronic toxicity of copper to five benthic invertebrates in laboratory-formulated sediment: Sensitivity comparison and preliminary risk assessment, Sci. Total Environ., 2007, 387, 128–140 CrossRef CAS PubMed.
- B. Berthet, C. Mouneyrac, J. C. Amiard, C. Amiard-Triquet, Y. Berthelot, A. Le Hen, O. Mastain, P. S. Rainbow and B. D. Smith, Accumulation and soluble binding of cadmium, copper, and zinc in the polychaete Hediste diversicolor from coastal sites with different trace metal Bioavailabilities, Arch. Environ. Contam. Toxicol., 2003, 45, 468–478 CrossRef CAS PubMed.
- J. J. Beukema, Predation by 3-spined stickleback (gasterosteus aculeatus l) - influence of hunger and experience, Behaviour, 1968, 31(1), 1–126 CAS.
- M. K. Saiki, D. T. Castleberry, T. W. May, B. A. Martin and F. N. Bullard, Copper, cadmium, and zinc concentrations in aquatic food-chains from the upper sacramento river (california) and selected tributaries, Arch. Environ. Contam. Toxicol., 1995, 29, 484–491 CrossRef CAS.
- M. K. Saiki, B. A. Martin, L. D. Thompson and D. Welsh, Copper, cadmium, and zinc concentrations in juvenile chinook salmon and selected fish-forage organisms (aquatic insects) in the upper Sacramento River, California, Water, Air, Soil Pollut., 2001, 132, 127–139 CrossRef CAS.
- S. Smith, M. H. Chen, R. G. Bailey and W. P. Williams, Concentration and distribution of copper and cadmium in water, sediments, detritus, plants and animals in a hardwater lowland river, Hydrobiologia, 1996, 341, 71–80 CrossRef CAS.
- S. J. Clearwater, A. M. Farag and J. S. Meyer, Bioavailability and toxicity of dietborne copper and zinc to fish, Comp. Biochem. Physiol., Part C: Toxicol. Pharmacol., 2002, 132, 269–313 CrossRef.
- B. Michen, C. Geers, D. Vanhecke, C. Endes, B. Rothen-Rutishauser, S. Balog and A. Petri-Fink, Avoiding drying-artifacts in transmission electron microscopy: Characterizing the size and colloidal state of nanoparticles, Sci. Rep., 2015, 5, 9793 CrossRef CAS.
- F. Gottschalk, T. Y. Sun and B. Nowack, Environmental concentrations of engineered nanomaterials: Review of modeling and analytical studies, Environ. Pollut., 2013, 181, 287–300 CrossRef CAS PubMed.
- J. Fabrega, S. N. Luoma, C. R. Tyler, T. S. Galloway and J. R. Lead, Silver nanoparticles: Behaviour and effects in the aquatic environment, Environ. Int., 2011, 37, 517–531 CrossRef CAS PubMed.
- T. Ramskov, A. Thit, M.-N. Croteau and H. Selck, Biodynamics of copper oxide nanoparticles and copper ions in an oligochaete – Part I: Relative importance of water and sediment as exposure routes, Aquat. Toxicol., 2015, 164, 81–91 CrossRef CAS.
- J. C. Amiard, C. Amiard-Triquet, S. Barka, J. Pellerin and P. S. Rainbow, Metallothioneins in aquatic invertebrates: Their role in metal detoxification and their use as biomarkers, Aquat. Toxicol., 2006, 76, 160–202 CrossRef CAS PubMed.
- C. Mouneyrac, O. Mastain, J. C. Amiard, C. Amiard-Triquet, P. Beaunier, A. Y. Jeantet, B. D. Smith and P. S. Rainbow, Trace-metal detoxification and tolerance of the estuarine worm Hediste diversicolor chronically exposed in their environment, Mar. Biol., 2003, 143, 731–744 CrossRef CAS.
- T. Y. T. Ng, P. S. Rainbow, C. Amiard-Triquet, J.-C. Amiard and W.-X. Wang, Decoupling of cadmium biokinetics and metallothionein turnover in a marine polychaete after metal exposure, Aquat. Toxicol., 2008, 89, 47–54 CrossRef CAS PubMed.
- A. Dagnino, J. I. Allen, M. N. Moore, K. Broeg, L. Canesi and A. Viarengo, Development of an expert system for the integration of biomarker responses in mussels into an animal health index, Biomarkers, 2007, 12, 155–172 Search PubMed.
- R. Ma, J. Stegemeier, C. Levard, J. G. Dale, C. W. Noack, T. Yang, G. E. Brown and G. V. Lowry, Sulfidation of copper oxide nanoparticles and properties of resulting copper sulfide, Environ. Sci.: Nano, 2014, 1, 347–357 RSC.
- T. Wang, X. H. Long, Y. Z. Cheng, Z. P. Liu and S. H. Yan, The potential toxicity of copper nanoparticles and copper sulphate on juvenile Epinephelus coioides, Aquat. Toxicol., 2014, 152, 96–104 CrossRef CAS PubMed.
- L. Bervoets, R. Blust and R. Verheyen, Accumulation of Metals in the Tissues of Three Spined Stickelback (Gasterosteus aculeatus) from Natural Fresh Waters, Ecotoxicol. Environ. Saf., 2001, 48, 117–127 CrossRef CAS PubMed.
- H. Roussel, S. Joachim, S. Lamothe, O. Palluel, L. Gauthier and J. M. Bonzom, A long-term copper exposure on freshwater ecosystem using lotic mesocosms: individual and population responses of three-spined sticklebacks (Gasterosteus aculeatus), Aquat. Toxicol., 2007, 82, 272–280 CrossRef CAS PubMed.
-
C. M. Wood, A. P. Farrell and C. J. Brauner, Homeostasis and Toxicology of Essential Metals, Elsevier, 2012 Search PubMed.
- M. H. G. Berntssen, K. Hylland, S. E. Wendelaar Bonga and A. Maage, Toxic levels of dietary copper in Atlantic salmon (Salmo salar L.) parr, Aquat. Toxicol., 1999, 46, 87–99 CrossRef CAS.
- R. D. Handy, The effect of acute exposure to dietary Cd and Cu on organ toxicant concentrations in rainbow trout, Oncorhynchus mykiss, Aquat. Toxicol., 1993, 27, 1–14 CrossRef CAS.
- S. J. Clearwater, S. J. Baskin, C. M. Wood and D. G. McDonald, Gastrointestinal uptake and distribution of copper in rainbow trout, J. Exp. Biol., 2000, 203, 2455–2466 CAS.
- R. D. Handy, The assessment of episodic metal pollution. II. The effects of cadmium and copper enriched diets on tissue contaminant analysis in rainbow trout (Oncorhynchus mykiss), Arch. Environ. Contam. Toxicol., 1992, 22, 82–87 CrossRef CAS PubMed.
- S. R. Nadella, C. Bucking, M. Grosell and C. M. Wood, Gastrointestinal assimilation of Cu during digestion of a single meal in the freshwater rainbow trout (Oncorhynchus mykiss), Comp. Biochem. Physiol., Part C: Toxicol. Pharmacol., 2006, 143, 394–401 Search PubMed.
- M. F. El Basuini, A. M. El-Hais, M. A. O. Dawood, A. E.-S. Abou-Zeid, S. Z. El-Damrawy, M. M. E. L. S. Khalafalla, S. Koshio, M. Ishikawa and S. Dossou, Effect of different levels of dietary copper nanoparticles and copper sulfate on growth performance, blood biochemical profiles, antioxidant status and immune response of red sea bream (Pagrus major), Aquaculture, 2016, 455, 32–40 CrossRef CAS.
- H. Wang, H. Zhu, X. Wang, E. Li, Z. Du, J. Qin and L. Chen, Comparison of copper bioavailability in copper-methionine, nano-copper oxide and copper sulfate additives in the diet of Russian sturgeon Acipenser gueldenstaedtii, Aquaculture, 2018, 482, 146–154 CrossRef CAS.
- S. Ferruzza, M. Scacchi, M. L. Scarino and Y. Sambuy, Iron and copper alter tight junction permeability in human intestinal Caco-2 cells by distinct mechanisms, Toxicol. In Vitro, 2002, 16, 399–404 CrossRef CAS.
- S. Ferruzza, M. L. Scarino, G. Rotilio, M. R. Ciriolo, P. Santaroni, A. O. Muda and Y. Sambuy, Copper treatment alters the permeability of tight junctions in cultured human intestinal Caco-2 cells, Am. J. Physiol., 1999, 277, G1138–G1148 CAS.
- R. Rincon-Heredia, D. Flores-Benitez, C. Flores-Maldonado, J. Bonilla-Delgado, V. García-Hernández, O. Verdejo-Torres, A. M. Castillo, I. Larré, A. C. Poot-Hernández, M. Franco, P. Gariglio, J. L. Reyes and R. G. Contreras, Ouabain induces endocytosis and degradation of tight junction proteins through ERK1/2-dependent pathways, Exp. Cell Res., 2014, 320, 108–118 CrossRef CAS.
Footnotes |
† Electronic supplementary information (ESI) available. See DOI: 10.1039/c9en00093c |
‡ Shared first authorship. |
|
This journal is © The Royal Society of Chemistry 2019 |
Click here to see how this site uses Cookies. View our privacy policy here.