The food additive E171 and titanium dioxide nanoparticles indirectly alter the homeostasis of human intestinal epithelial cells in vitro†
Received
24th October 2018
, Accepted 22nd November 2018
First published on 11th April 2019
Abstract
Environmental contamination with TiO2 and the use of TiO2 as a food additive (E171) or in cosmetics result in human exposure to TiO2via inhalation, ingestion, and through skin contact. When inhaled, most TiO2 particles are cleared via the mucociliary escalator and are then swallowed. Together with the ingestion of E171, this process results in a significant exposure of the human gastro-intestinal tract to TiO2. One of the functions of the intestine is to protect the body from external aggression, via the so-called intestinal barrier function. The aim of this study was to determine whether, and through which mechanisms, TiO2 affects this function. Caco-2 and HT29-MTX cells were co-cultured to reconstitute an in vitro mucus-secreting intestinal epithelium. This epithelium was exposed to TiO2-NPs, either pure anatase or mixed anatase/rutile, or to E171. Two exposure scenarii were used: acute exposure for 6 h or 48 h after cell differentiation (21 days post-seeding), or repeated exposure during the course of cell differentiation, i.e., twice a week for 21 days post-seeding. Epithelial cells repeatedly exposed to TiO2 developed an inflammatory profile, together with increased mucus secretion. Epithelial integrity was unaltered, but the content of ATP-binding cassette (ABC) family xenobiotic efflux pumps was modified. Taken together, these data show that TiO2 moderately but significantly dysregulates several features that contribute to the protective function of the intestine.
Environmental significance
TiO2 is widely used in many everyday products. As a result, it may be released into the environment. While TiO2 nanoparticles (TiO2-NPs) are mainly used as catalysts, the highest tonnage of TiO2 production is for pigment-grade particles, for use as whitening and opacifying agents in paints or food, where it is referred to as E171. Due to its multiple uses, TiO2 can be ingested by the population both when consuming food and when inhaling contaminated air, followed by mucociliary clearance and then swallowing. This article reports data on the intestinal impact of TiO2, both as nanoparticles and as the E171 food additive, when ingested. It therefore deals with the consequences of environmental contamination by TiO2.
|
Background
TiO2 is widely used in many everyday products.1 As a result, it may be released into the environment. While TiO2 nanoparticles (TiO2-NPs) are mainly used as catalysts, the highest tonnage of TiO2 production is for pigment-grade particles, for use as whitening and opacifying agents in paints or food (where it is referred to as E171 in the European Union). Pigment-grade TiO2 is composed of particles with a mean diameter >100 nm, thus it is not a nanomaterial as defined according to the EU Recommendation on the definition of a nanomaterial.2 However, recent reports indicate that it can contain up to 55% of nanoparticles.3–6 Due to its multiple uses, nano- or micro-sized TiO2 can be ingested by the population both when consuming food and when inhaling contaminated air, followed by mucociliary clearance and swallowing.7 Human oral exposure to TiO2 is estimated to range between 0.2 and 0.4 mg per kg of body weight (b.w.) in infants and the elderly, and up to 5.5–10.4 mg per kg b.w. in children, depending on the exposure scenario.8 TiO2 intake occurs mostly via the ingestion of candy, coffee creamer and sauces, and toothpaste by young children.9
In vivo, some studies have shown that pigment-grade TiO2 does not induce significant toxic effects when administered orally to rodents,10–12 whereas others have indicated that food-grade TiO2 enhances tumor formation in a colitis-associated cancer model13 and it spontaneously triggers the formation of preneoplastic lesions in the normal colonic mucosa, while it promoted their development in a carcinogenesis model.14 These effects are associated with the deregulated expression of genes involved in inflammatory, immunological, and specific cancer-related pathways.15 Moreover, in vitro, E171 and TiO2-NPs induce damage to DNA, including base oxidation,3 strand breaks, and chromosomal damage,16via oxidative mechanisms.3,16
The role of the intestine is to absorb nutrients and water, and to protect the organism from external pathogens and harmful substances. This protective function, known as the intestinal barrier function (IBF), relies on several lines of defense. The intestine is populated by commensal bacteria, collectively named the microbiota, which stimulate intestinal immunity and produce anti-microbial substances that eliminate pathogenic bacteria.17 Intestinal epithelial cells are covered with mucus, composed of mucins secreted by goblet cells, which forms a protective blanket on the surface of the epithelium, creating a physical and chemical barrier to pathogens and xenobiotics.18 The intestinal epithelium is composed of cells that are linked together by tight junctions, which strictly control any transfer from the gut lumen into internal tissues.19 Moreover, enterocytes express specific membrane transporters, which actively transfer xenobiotics from the intracellular compartment to the gut lumen for elimination.20 Finally, the gut-associated lymphoid tissue (GALT) is where the intestinal immune response is launched as the last line of defense.21 The IBF is impaired in inflammatory bowel diseases (IBD), including ulcerative colitis (UC) and Crohn's disease (CD). IBD consists in an abnormal response to the host's luminal bacteria, characterized by increased epithelial barrier permeability, a defective mucus layer, alteration of the so-called pattern-recognition receptors, including nucleotide-binding oligomerization domain (NOD)-like receptors (NLRs) and toll-like receptors (TLRs), and a disturbance of antimicrobial peptide production.22 IBF defects trigger direct contact between the intestinal epithelium and bacteria, which destabilizes mucosal immune mechanisms and causes inflammation. The ingestion of food contaminants and food additives has been suggested as a potential causative factor in these diseases.23,24 This hypothesis is supported by several recent reports showing that TiO2-NPs and food-grade TiO2 may disturb tight junctions after oral administration to mice, leading to increased intestinal permeability.25 TiO2 particles accumulate in mucus-secreting cells in vitro25 and cause their number to decrease in vivo.13 TiO2-NPs also increase the intracellular Ca2+ content,26 which promotes mucus secretion.27 However, a recent study rather showed that the sub-chronic oral exposure of rats to E171 had little or no impact on the quantity and quality of intestinal mucus.28 Overall, the impact of TiO2 on intestinal microbiota is limited. Waller et al. demonstrated a modification of the microbial composition and biochemical responses of the microbiota after exposure to food-grade TiO2 particles in an in vitro model human colon reactor.29 Chen et al. reported no disturbance of mice microbiota after exposure to TiO2-NPs,30 while Dudefroi et al. reported a limited effect of E171 on a MET-1 model intestinal bacterial community.31 Finally, E171 and TiO2-NPs destabilized immune homeostasis in orally exposed rats,14 whereas TiO2-NPs induced a pro-inflammatory response in peripheral blood mononuclear cells,32 macrophages,33 and buccal cells34in vitro.
The aim of the present study was to determine whether exposure to TiO2 alters the intestinal barrier function, with a focus on enterocytes (responsible for epithelial barrier integrity) and on mucus-producing goblet cells. It also aimed at identifying the molecular mechanisms involved in the altered epithelial cell homeostasis, if any. To address these questions, enterocyte-like (Caco-2) and goblet cells (HT29-MTX) were co-cultured in a ratio of 70
:
30 Caco-2
:
HT29-MTX. This co-culture mimics the mucus-secreting epithelium in the large intestine.35,36 Two exposure scenarii were tested: i) cells were exposed continuously for 21 days, during the differentiation of Caco-2 cells to enterocytes, or ii) differentiated cells were exposed to acute doses of TiO2 for 6 h or 48 h. Exposure concentrations were chosen as 10 or 50 μg mL−1. The daily intake of TiO2 from food is estimated by EFSA to range between 0.5 and 5.7 mg per kg b.w. per day in adults, when considering the refined level exposure assessment scenario in a brand-loyal scenario.37 Considering that the weight of a male adult is approximately 70 kg, and that their intestine surface is 250 m2, the high range value corresponds to the ingestion of ∼400 mg TiO2 per day, i.e., 0.16 μg TiO2 cm−2 of intestine. In our exposure conditions, 10 μg mL−1 corresponds to 2.5 μg cm−2 of cells, i.e., 15-fold more than the human daily exposure. This is a high dose compared to human exposure, but such high doses are not unusual in in vitro assays. Moreover, this calculation is a rough estimate because it does not take into account the dynamic flows of food in the intestine. In the chronic exposure scenario, Caco-2 and HT29-MTX cells are still proliferative during the first week of exposure, until they reach confluence. Then during the two following weeks of exposure, epithelial cells forming the monolayer epithelium start to differentiate into enterocytes and goblet cells, as in the intestine. TiO2 was presented in the form of E171, P25 TiO2-NPs (mixed anatase/rutile, 25 nm, NM105 from the Joint Research Center's (JRC) nanomaterial library), or A12 TiO2-NPs (pure anatase, 12 nm), as in our previous study.3 In this previous study, E171 and TiO2-NPs were shown to induce moderate damage to these cells, including an accumulation of reactive oxygen species, downregulation of the expression of antioxidant enzymes, and oxidative damage to DNA.3 E171 was shown to induce more damage than P25, while A12 had nearly no effect. No endoplasmic reticulum stress was observed.3 The main molecular mechanism of E171 and TiO2-NP toxicity was therefore via oxidative stress.3 As a follow-up, the inflammatory profile and impact on mucus secretion as well as on epithelial integrity and on the expression of xenobiotic efflux pumps were assessed in exposed cells, as indicators of intestinal barrier function.
Material and methods
Chemicals
Unless otherwise indicated, all chemicals were purchased from Sigma-Aldrich and were >99% pure. Cell culture media and serum were purchased from Thermo Fisher Scientific.
Particle dispersion and characterization
E171 was purchased from a French commercial source. A12 TiO2-NPs were produced in our laboratory38 and P25 TiO2-NPs were obtained from the European JRC (Ispra, Italy), where they are referenced as NM105 in the nanomaterial library. The TiO2 particles were the same as those used and characterized in our previous studies.3,25,39 TiO2 particles (10 mg mL−1) were suspended in ultrapure sterile water and dispersed using an indirect cup-type sonicator (Vibracell 75041, Bioblock Scientific), operated in continuous mode for 30 min at 80% amplitude, and maintaining the temperature at 4 °C. After dispersion, the zeta potential and agglomeration state of these suspensions were characterized by dynamic light scattering on a ZetaSizer nanoZS (Malvern Instrument). The physicochemical characteristics of E171, A12, and P25 were described previously.3 Briefly, E171 was anatase, with traces of rutile (<5%), as previously reported. Its specific surface area was 9.4 m2 g−1. Its mean primary diameter was 119 nm, with 47% of the particles (by number) having a diameter <100 nm. Its hydrodynamic diameter in water was 415.4 ± 69.5 nm (polydispersity index: 0.48 ± 0.071). This value increased to 739.3 ± 355.3 nm in the exposure medium (polydispersity index: 0.64 ± 0.221) as the particles agglomerated. A12 was pure anatase, and P25 was 86% anatase and 14% rutile; their diameters were 12 and 21 nm, respectively.3 As for E171, the water suspensions of these particles were stable, and slight agglomeration occurred when the suspensions were diluted in cell culture medium.3
Cell culture
Caco-2 (ATCC HTB-37, passages 49 to 60) and HT29-MTX (kindly provided by T. Lesuffleur, INSERM U843, Paris, France) were grown in Dulbecco's modified Eagle medium + GlutaMAX™ (Life Technologies) supplemented with 10% heat-inactivated fetal bovine serum, 1% non-essential amino acids, 50 units per ml penicillin, and 50 μg per ml streptomycin. They were maintained at 37 °C under 5% CO2. Depending on the experiment, the cells were seeded at a density of 24
000 cells per cm2 in Petri dishes, multi-well plates, or Transwell-Clear® inserts (polyester, 0.4 μm pores, Costar). Cells were grown as a co-culture of Caco-2 and HT29-MTX (70
:
30 CaCo-2
:
HT29-MTX), which mimics the distal colon (intestinal epithelium comprises approximately 10% goblet cells in the duodenum, with this proportion increasing along the intestine to reach 24% in the distal colon40). To monitor the release of cytokines and for TEER measurement on the monolayers, Caco-2 cells were also grown as a mono-culture. The characteristics of these cell models were reported in our previous article,3 including the presence of tight junctions and microvilli, as observed by transmission electron microscopy and by the secretion of alkaline phosphatase.
Exposure to particles
For acute exposure experiments, cells were grown to confluence and allowed to differentiate by maintaining them in culture for 21 days post-confluence, changing the cell culture medium three times a week. HT29-MTX cells secreted mucus as soon as they reach confluence, while Caco-2 cells completed their differentiation to enterocytes at 21 days post-confluence. Differentiated cells were then exposed for 6 h or 48 h to 50 μg per mL of particles, after dilution in complete cell culture medium, at pH 7.4. For the chronic (repeated) exposure experiments, two days after seeding, the cell culture medium was replaced by a suspension of TiO2 particles prepared in complete cell culture medium. Depending on the experiment, the TiO2 concentration was 50 μg mL−1 (monitoring of cell differentiation, inflammation), 10 and 50 μg mL−1 (efflux pumps and mucin gene expression and protein level identification), or 1, 10, 50, 100, and 200 μg mL−1 (mucus secretion). In this exposure scenario, in the first days of exposure, the Caco-2 cells were not fully differentiated to enterocytes, i.e., they did not have any microvilli and showed a low value of TEER. The TiO2-containing medium was renewed twice a week for 3 weeks, i.e., cells received 8 doses (a schematic representation of the exposure procedure is presented in the Fig. S1†).
Monitoring cellular differentiation to enterocytes
Tight junction formation and epithelial integrity were monitored by TEER measurement, using an Electrical Resistance System Millicell® ERS-2, equipped with a chopstick electrode (Millipore). Cells were grown on Transwell-Clear inserts (polyester, 0.4 μm pore size), with 4.7 cm2 of growth area, then exposed to TiO2 particles repeatedly for 21 days as described in the previous section. The resistance was measured at days 2, 4, 7, 9, 11, 15, and 21 post-seedling, and TEER was calculated as TEER (Ω cm2) = [sample resistance – blank resistance (Transwell without cells)] × Transwell membrane area. At these time-points, microvilli formation was also probed, by alkaline phosphatase (ALP) activity monitoring, using an alkaline phosphatase activity fluorometric assay kit (Biovision) following the supplier's instructions. Finally, cell differentiation was monitored using RT-qPCR analysis of the expression of genes involved in tight and adherens junctions (claudin 1 (CLDN1), occludin (OCLN), tight junction protein 1 (TJP1), beta-catenin (CTNNB1)), and in microvilli differentiation (sucrase isomaltase (SI), and alkaline phosphatase (ALPI)).
Gene expression
Gene expression was measured by real-time-quantitative polymerase chain reaction (RT-qPCR). Cells were harvested in the lysis buffer supplied in the RNA extraction kit and stored at −80 °C. RNA was then extracted using the GenElute™ mammalian total RNA Miniprep kit (Sigma-Aldrich). Total RNA concentration and purity were determined by measuring the absorbance (abs) at 260 nm, as well as at abs 260/abs 280 nm and abs 260/abs 230 nm ratios. Total RNA (2 μg) was reverse transcribed to produce cDNA with 100 ng μL−1 random primers, 10 mM dNTP, and SuperScript III Reverse Transcriptase (Invitrogen). Total cDNA concentration and purity were determined by measuring abs at 260 nm as well as the abs 260/abs 280 nm and abs 260/abs 230 nm ratios. MESA Blue qPCR Mastermix for SYBR Assay (Eurogentec) with the ROX reference was used to perform the quantitative PCR in an MX3005P multiplex quantitative PCR system (Stratagene). The primer sequences are listed in the Table S1,† except for MUC2, which was purchased from Bio-Rad. Primer efficiencies were determined, and only primers with an efficiency between 1.8 and 2.2 were used. The relative expression values were calculated as 2−ΔΔCq, where ΔCq is the difference between the cycle threshold (Cq) values for the target and reference genes for each condition, and ΔΔCq is the difference between the ΔCq measured for one exposure condition and that for the respective control condition (unexposed cells). CYCLOA and B, S18 and GAPDH were used as reference genes for data normalization. Their variability of expression was assessed using Bestkeeper, which is an Excel-based pairwise mRNA correlation tool.41 Gene expression levels were determined and statistical analyses performed using the Relative Expression Software Tool (REST2009).42
Western blot
Quantification of ABC transporters was assessed by Western blot. Total proteins were extracted from cells using CelLytic M reagent (Sigma-Aldrich) and quantified. Denatured proteins (20 μg) were deposited on three independent stain-free 7.5% polyacrylamide gels (TGX Stain-Free FastCast Acrylamide kit, 7.5%, Bio-Rad). Four replicates were performed for each condition, and control samples were deposited on each gel. Electrophoresis was performed at 200 V for 40 min; then the proteins were transferred onto nitrocellulose membranes using a 0.2 μm Nitrocellulose Midi Trans-Blot Turbo Transfer Pack (Bio-Rad). Specific antibodies (MDR1: ab170904 1/2500; MRP1: ab24102 1/500; MRP2: ab3373 1/200; BCRP: ab108312 1/2500, all from Abcam) were prepared in TBS containing 0.5% Tween-20 and 5% non-fat dried milk, and applied to the membranes. Proteins were revealed using the Clarity™ Western ECL Substrate (Bio-Rad) and the band-intensity was quantified by chemiluminescence (Bio-Rad, ChemiDoc™ XRS+). The intensity of each band was normalized with respect to the total protein content in the lane and divided by the normalized intensity measured for the control cells.
Analysis of mucus secretion
For qualitative identification of mucus secretion, epithelial monolayers were stained with Alcian blue. After 21 days of growth in 6-well plates, the cells were carefully washed three times with PBS. Then, a fixative solution composed of 3 volumes of 90% ethanol mixed with 1 volume of 3% acetic acid was applied on the cells, which were then incubated for 20 min at RT. This solution was then replaced by Alcian blue, pH 2.5, dissolved in 3% acetic acid. After incubation for 30 min at RT in the dark, under gentle agitation, the cells were washed twice, before observation and image capture using an optical microscope (Axiovert 25 Zeiss). Mucus secretion was quantified using the periodic acid-Schiff assay on cells seeded in 12-well plates (n = 3). After 21 days, the cells were harvested and simultaneously lysed in PBS + Triton (1%). Duplicate aliquots (25 μL) of each cell lysate were deposited in 96-well plates, and then 120 μL of 0.6% periodic acid was added to each well and the mixture was incubated for 90 min at 37 °C. Finally, 100 μL of Schiff reagent was added to each well and incubated for 5 min with agitation at RT, in the dark, followed by 40 min without agitation. Absorbance was measured at 550 nm using a SpectraMax M2 (Molecular Devices). A positive control was prepared with increasing concentrations of porcine stomach mucin (100–5000 μg mL−1). No interference of NPs with the assay was detected when 10, 50, or 100 μg per mL of particles were added to empty wells.
Inflammation
The Bio-Plex Pro Human Cytokine Assay multiplex immunoassay (BioPlex MagPix™ Bio-Rad) was used to characterize the inflammatory profile of cells exposed to TiO2 particles. This measures the content of 27 cytokines, which can be classified as interleukins (IL-1β, 2, 4, 5, 6, 7, 9, 10, 12, 13, 15, 17A), chemokines (IL-8, eotaxin, RANTES, IP-10, MCP-1, MIP-1α, MIP-1β), tumor necrosis factor (TNF-α), interferon (IFN-γ), colony stimulating factors (G-CSF and GM-CSF), and growth factors (PDGF-BB, bFGF, and VEGF). Although epithelial cells generally do not produce some of the cytokines in this panel, we opted to screen most of them, because some authors have reported their production by Caco-2 cells.43 We however did not investigate the production of IL-2, IL-4, IL-5, IL-9, IL-12, IL-13, and IFNγ, for which the probability of production by Caco-2 and HT29-MTX was very low. Cytokine concentration was measured in the supernatants of exposed cells, in triplicate for each condition. The limits of detection (LD) and quantification (LQ) were determined for each cytokine, according to the manufacturer's instruction. Briefly, for each cytokine, a blank as well as the lowest and highest detectable values were determined from the standard curve.
Statistical analysis
Statistical analyses were performed using Statistica 8.0 software (Statsoft, Chicago, USA). Unless indicated otherwise, statistical significance was assessed based on a non-parametric one-way analysis of variance on ranks approach (Kruskal–Wallis) followed by pairwise comparison using the Mann–Whitney u-test. Results were considered statistically significant when the p-value was <0.05.
Results
Repeated exposure to TiO2 particles does not affect the differentiation of Caco-2 cells to enterocytes
As previously reported, repeated exposure to A12, P25, or E171 for 21 days did not affect cell viability in the Caco-2/HT29-MTX co-culture, assessed via trypan blue assay.3 We monitored enterocyte differentiation all along the 21 days of exposure to E171 and P25, by measuring the expression of genes encoding proteins involved in adherens and tight junction (CLDN1, OCLN, TJP1, CTNNB1), and in microvilli differentiation (SI, ALPI) (Fig. 1), as well as the TEER and alkaline phosphatase activity (Fig. 2). Although in some conditions, mRNA expression levels for some of these genes were moderately altered in exposed cells compared to control cells (Fig. 1), the TEER value and alkaline phosphatase activity were unchanged (Fig. 2). The TEER, which reflects tight junction development, increased progressively from day 2 to day 7, reaching a plateau at 600–800 Ω cm2 (Fig. 2A). Alkaline phosphatase activity, which reflects microvilli development, also increased progressively from day 4 to day 14, finally reaching a plateau at 0.2 U ALP per g protein (Fig. 2B). This indicates that neither tight junction development nor microvilli formation was affected by the continuous exposure to E171 or P25.
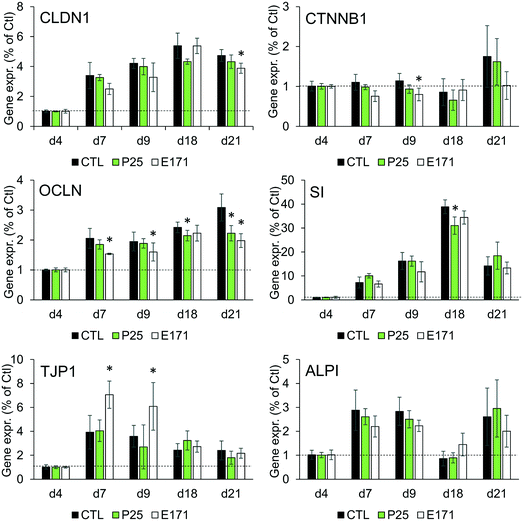 |
| Fig. 1 Impact of E171 and TiO2-NPs on the mRNA level of genes involved in enterocytic differentiation. The mRNA expression of claudin 1 (CLDN1), occludin (OCLN), ZO-1 (TJP1), catenin (CTNNB1), sucrase isomaltase (SI), and intestinal alkaline phosphatase (ALPI) were monitored by RT-qPCR during the 21 days necessary for Caco-2/HT29-MTX co-culture to differentiate to enterocytes and goblet cells. During these 21 days, cells were exposed to 50 μg per mL of E171 or TiO2-NPs three times per week. Results are expressed as fold-increase relative to the control (cells four days after seedling); mean ± standard deviation, statistical significance *p < 0.05 exposed vs. control at each time-point, n = 3. | |
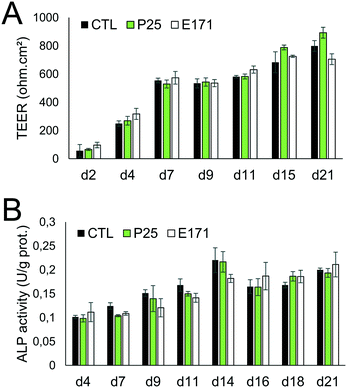 |
| Fig. 2 E171 and TiO2-NPs do not affect epithelial differentiation. Epithelial differentiation was monitored via TEER measurement (A), which is a marker of tight junction formation, and alkaline phosphatase (ALP) activity measurement (B), which is a marker of microvilli development. Caco-2/HT29-MTX cells were repeatedly exposed to 50 μg per mL of P25 TiO2-NPs or E171, and harvested at day 4, 7, 9, 11, 14, 16, 18, and 21 post-seeding. At each time-point, TEER and ALP activity were measured. Results are expressed as mean ± standard deviation, statistical significance *p < 0.05 exposed vs. control at each time-point, n = 3. | |
Repeated exposure to TiO2 particles triggers an inflammatory profile
We monitored the cytokine profile in cells exposed to TiO2-NPs using the BioPlex MagPix™ technology. The results are represented as a heat map, showing fold-changes for cytokine levels in exposed cells, compared to their respective controls (Fig. 3). Fold-changes lower than 1 (decreased cytokine secretion in exposed cells, compared to unexposed cells) are indicated in green, while fold-changes higher than 1 (increased secretion) are indicated in red. When the cytokine level is unchanged, the box appears black. Exposure to TiO2 particles induced an overall decrease in cytokine release by Caco-2/HT29-MTX cells. This suggests an overall decrease in cellular functions (e.g., due to decreased cellular metabolism), or of the cytokine release process (e.g., impact on the secretory pathway). In addition, continuous exposure to E171 induced an inflammatory profile in the Caco-2/HT29-MTX co-culture, with the increased release of IL-1β (fold-change 10.91), IL-17A (fold-change 7.2), eotaxin (fold-change 3.02), and RANTES (fold-change 2.13). P25 induced a comparable response, with increased levels of IL1-β (fold-change 10.5), IL-17A (fold-change 3.8), and eotaxin (fold-change 6.81). P25 also triggered significant release of the chemokine CXCL-8 (IL-8, fold-change 3.83). In contrast, no inflammatory profile was detected in cells continuously exposed to A12. Since no inflammatory response was measured in a monoculture of Caco-2 cells exposed to A12 or E171, this suggest that the presence of mucus-secreting cells, or of mucus islands on top of the cells, is required to promote the development of an inflammatory response.
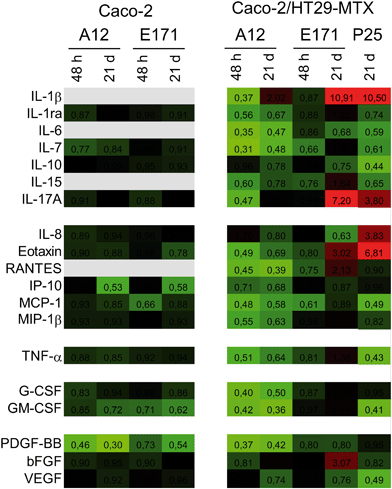 |
| Fig. 3 E171 and TiO2-NPs trigger an inflammatory response. The BioPlex MagPix™ technology was used to screen cells for the expression of the following 27 cytokines: interleukins (IL-1β, 2, 4, 5, 6, 7, 9, 10, 12, 13, 15, 17A), chemokines (IL-8, eotaxin, RANTES, IP-10, MCP-1, MIP-1β, and MIP-1α, the latter was under the LQ in both cell models and is therefore absent from this heat map), tumor necrosis factor family (TNF-α), interferon (IFN-γ), colony stimulating factors (G-CSF and GM-CSF), and growth factors (PDGF-BB, basic FGF, and VEGF). Caco-2 and Caco-2/HT29-MTX were exposed to 50 μg per mL of E171 or A12, either acutely for 48 h or repeatedly twice a week for 21 days. In addition, the secretion of these cytokines was measured in Caco-2/HT29-MTX co-cultures repeatedly exposed to 50 μg per mL of P25. Results represent fold-change of protein concentration in exposed cells vs. control cells. They are presented as a heat map, with the color scale ranging from light green to black (fold-change: 0 to 1) and from black to light red (fold-change: 1 to 11); gray: below the limit of detection. Numbers indicated in boxes correspond to fold-changes. | |
TiO2 affects mucus secretion
Since environmental stimuli, including inflammatory cytokines, have been reported to modulate the release of mucins in the intestine and their glycosylation level,18 the mucus content was analyzed in cells chronically exposed to TiO2 particles. Alcian blue is a cationic dye that binds to negatively charged mucins, i.e., the acidic mucins. Staining with this dye showed that mucus was distributed as discrete islands in unexposed Caco2/HT29-MTX cells (Fig. 4A), certainly on top of HT29-MTX as previously reported.36 When cells were repeatedly exposed to E171, staining also showed islands of mucus (Fig. 4B–F). Periodic acid-Schiff (PAS), which quantitatively stains acidic and neutral mucins by reacting with glycols from their carbohydrate chains, showed that higher levels of mucins were detected in HT29-MTX cells continuously exposed to 100 μg per mL of E171, compared to the control cells (Fig. 4G). In Caco-2/HT29-MTX cells, significantly increased mucin staining was observed after exposure to 100 μg per mL of A12, or 10, 50, or 100 μg per mL of P25 or E171 (Fig. 4H). This effect may be due to the increased production or secretion of acidic and/or neutral mucins. To explore this hypothesis, mucin mRNA expression was monitored in Caco-2/HT29-MTX cells exposed to TiO2. The mRNA expression levels for MUC1, MUC2, MUC12, and MUC17 was either unchanged, or moderately downregulated in exposed cells compared to the control cells (Fig. 5). In addition, the expression of trefoil factor 3 (TFF3) and Kruppel-like factor 4 (KLF4), which are involved in goblet cell differentiation44 and in controlling mucus rheology,18 were also moderately downregulated in cells continuously exposed to E171 or TiO2-NPs (Fig. 5). This suggests that the increased PAS staining in cells chronically exposed to TiO2 results from the increased secretion of stored mucins, rather than from a transcriptional response.
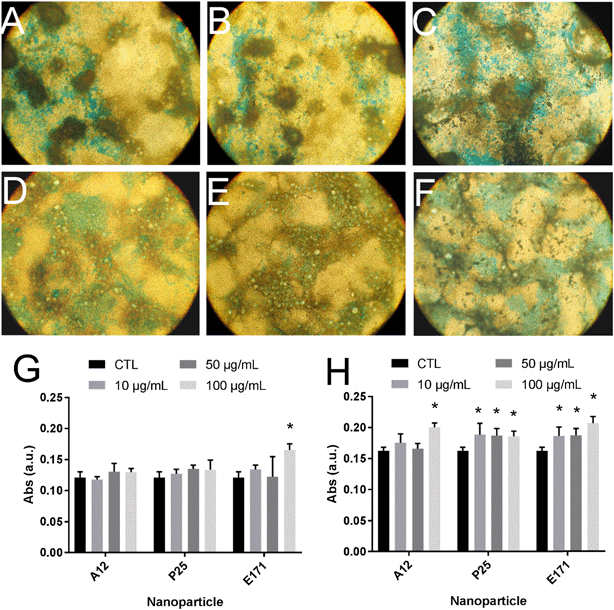 |
| Fig. 4 E171 and TiO2-NPs increase mucus staining intensity. Mucus was imaged after Alcian blue staining (×10 magnification) of Caco-2/HT29-MTX co-cultures either not exposed (A) or repeatedly exposed to 1 (B), 10 (C), 50 (D), 100 (E), or 200 (F) μg per mL of E171 for 21 days. Alternatively, it was quantified using the periodic acid-Schiff technique in HT29-MTX monoculture (G) or Caco-2/HT29-MTX co-culture (H), repeatedly exposed to 1, 10, 50, or 100 μg per mL of A12 or P25 or E171 for 21 days. In G and H, the results are expressed as fold-change relative to the control (unexposed cells); mean ± standard error of the mean, statistical significance *p < 0.05, exposed vs. control, n = 4. | |
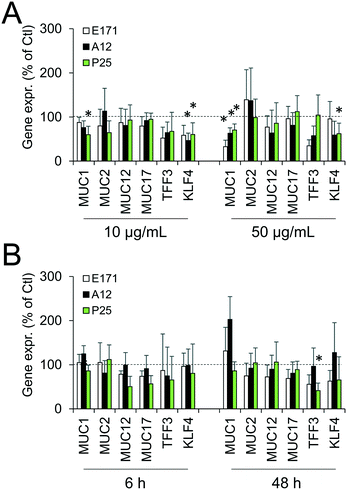 |
| Fig. 5 E171 and TiO2-NPs do not alter mRNA expression levels for genes encoding mucus proteins. mRNA expression levels were measured for 6 proteins and transcription factors involved in mucus composition and secretion by quantitative PCR in the Caco-2/HT29-MTX co-culture. The products monitored were: mucin 1 (MUC1), mucin 2 (MUC2), mucin 12 (MUC12), mucin 17 (MUC17), trefoil factor 3 (TFF3), Kruppel-like-factor 4 (KLF4). Cells were either repeatedly exposed to E171, A12, or P25 NPs at 10 and 50 μg mL−1 twice a week for 21 days (A), or acutely exposed to E171, A12 or P25 NPs at 50 μg mL−1 for 6 h and 48 h (B). Results are expressed as fold-increase relative to the control (unexposed cells); mean ± standard deviation, statistical significance *p < 0.05 exposed vs. control (unexposed cells), n = 3. | |
TiO2 modulates the expression of xenobiotic efflux pumps without altering epithelial integrity
Cellular levels of BCRP, MRP1, MRP2, and MDR1—membrane transporters belonging to the ABC family, responsible for the efflux of xenobiotics—were modulated (Fig. 6). Acute exposure of Caco-2/HT29-MTX to E171 for 6 h increased the content of BCRP, MRP1, and MDR1, while acute exposure to P25 only increased the content of MRP1. In contrast, cells repeatedly exposed to E171 showed a trend for decreased levels of BCRP, MRP2, and MDR1, whereas P25 rather increased the content of MRP2 and MDR1. No response was recorded with A12 in either acute or chronic exposure conditions. Only a moderate modulation of the mRNA expression for these efflux pumps was noted, with a trend for downregulated expression (Fig. 7).
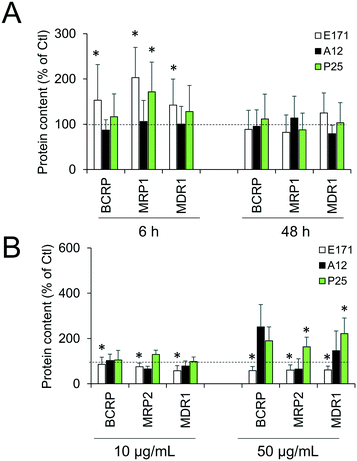 |
| Fig. 6 E171 and TiO2-NPs alter levels of ABC transporters. Protein content was quantified by Western blot. Caco-2/HT29-MTX co-culture were either acutely exposed to 50 μg per mL of E171, A12, or P25 for 6 h or 48 h (A) or repeatedly-exposed twice a week for 21 days to 10 or 50 μg per mL of E171, A12, or P25 (B). Results are expressed as fold-increase relative to the control (unexposed cells); mean ± standard deviation; statistical significance *p < 0.05 exposed vs. control, n = 4. | |
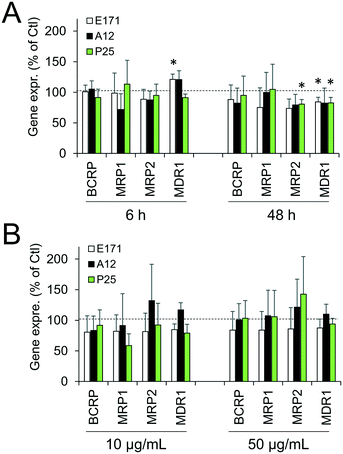 |
| Fig. 7 E171 and TiO2-NPs do not alter mRNA expression levels for ABC transporters. mRNA expression levels for four ABC transporters involved in xenobiotic efflux were measured by RT-qPCR: BCRP, MRP1, MRP2, and MDR1. Caco-2/HT29-MTX cells were either acutely exposed to 50 μg per mL of E171, A12, and P25 for 6 h or 48 h (A) or repeatedly-exposed twice a week for 21 days to 10 or 50 μg per mL of E171, A12, or P25 (B). Results are expressed as fold-increase relative to the control (unexposed cells); mean ± standard deviation, statistical significance *p < 0.05 exposed vs. control, n = 3. | |
Discussion
In this study, we sought to decipher the molecular mechanisms of the impact of TiO2 on IBF, which relies on: i) the mucus coating the intestinal epithelium, which forms a physical and chemical barrier against pathogenic bacteria and xenobiotics, ii) the intestinal epithelium, which strictly controls the absorption of nutrients and water from the intestinal lumen and can expel xenobiotics, and iii) the cytokinic response of intestinal epithelial cells, which participate in intestinal immune system. IBF also relies on the microbiota, defined as the community of commensal bacteria present in the intestine, but this component was not assessed here.
Although the mRNA expression of some markers of intestinal epithelial differentiation was significantly changed at some time-points, repeated exposure of Caco-2/HT29-MTX cells to E171 or P25 over 21 days did not affect the TEER and alkaline phosphatase activity measurements, throughout the duration of TiO2 exposure. In some conditions, claudin 1, occludin and catenin β1 mRNA expressions were decreased, which would reflect decreased tightness of the junctions. Conversely, TJP1 mRNA expression was increased in other conditions, which would contrarily reflect increased tightness. The rates of gene expression change were low, which questions their physiological relevance, and indeed no phenotypical modification was observed, proving that TiO2 particles did not alter the process of enterocytic differentiation.
TiO2 particles triggered an inflammatory response in epithelial monolayers formed by the co-culture of Caco-2 and HT29-MTX cells. This response was characterized by an increased release of IL-1β, IL-8, IL-17A, eotaxin, and RANTES. An increased secretion of IL-1β is an indicator that the NLRP3 inflammasome is activated, as previously reported for TiO2-NPs in a number of cell models.1 Activation of this pathway was observed only in the co-culture with HT29-MTX cells, suggesting that either these cells, or the presence of mucus, was required. This result is consistent with previous reports showing that HT29-MTX respond to pro-inflammatory stimuli,45 while Caco-2 cells do not.46 An increased secretion of IL-1β has been reported to stimulate RANTES production in Caco-2 cells,47 which could explain the associated increased secretion of RANTES observed here. We detected relatively high levels of IL-17A in Caco-2/HT29-MTX (up to 128 pg mL−1), even if this was unexpected. IL-17A is reputedly only secreted by TH17 cells,48 but some reports indicate that it can also be secreted by Caco-2 cells.43 Its upregulation in cells exposed to E171 or P25, in addition to being a sign of intestinal inflammation,49 also suggests that these particles stimulate the intestinal barrier defenses, since IL-17A triggers the production of some protective factors, including defensins, mucins, and lipopolysaccharide-binding proteins.49 The secretion of eotaxin, which specifically attracts eosinophils, which are involved in allergic reactions, tumor immunity, and inflammatory diseases, including IBD, was also affected.50 Finally, CXCL-8 secretion was induced only in the Caco-2/HT29-MTX co-culture, and only upon exposure to P25. This result suggests that nanoparticles are more potent inducers of CXCL-8 release than microparticles, as previously demonstrated in lung epithelial cells.51 TiO2 also induces the release of this chemokine in undifferentiated Caco-2 cells,52,53 which are more sensitive than the differentiated Caco-2 cells used in the present study.54
Interestingly, a broad array of inflammatory cytokines, including IL-1β and IL-17A, modulate the release of mucins in the intestine.18 For this reason, the mucus content was explored in cells repeatedly exposed to TiO2 particles. By using Alcian blue, which exclusively stains acidic mucins, no significant change of mucus content was observed. Conversely, when using the periodic acid Schiff technique, which is quantitative and reveals both acidic and neutral mucins as well as some glycoproteins and carbohydrates,55 increased staining was observed. This would suggest that TiO2 increased the content of only neutral mucins or other glycoproteins or carbohydrates. However, this interpretation must be considered with caution, since Alcian blue staining relies on observation by the naked eye, which can easily be biased. This impact on mucus was not due to increased mucin gene expression since the mRNA expression for mucins was unchanged. Rather, it would reflect the increased release of mucins stored in cytoplasmic granules. Mucins and TFF genes are expressed long before proteins are secreted and detectable in the medium. This latency period is devoted to different steps of mucin biosynthesis, including N-glycosylation and other post-translational modifications, which are necessary for mucins folding, dimerization, O-glycosylation, multimerization, and storage in vesicles and secretory granules before their secretion.56 Then, mucin exocytosis occurs via two distinct mechanisms: constitutive secretion and regulated secretion. Constitutive secretion leads to a continuous release of mucin granules, while regulated secretion occurs via external stresses, including exposure to bacteria or inflammatory mediators.57 Likewise, TiO2 particles may trigger this stimulated secretion, as already shown in bronchial cells, where it was triggered by changes in cytosolic calcium concentration.27
Since mucus secreted by HT29-MTX has recently been shown to trap TiO2 particles, including E171,28 increased mucus secretion would increase TiO2 entrapment in the vicinity of cells. In similar experimental conditions, we previously showed that Ti intracellular accumulation is not significantly different in Caco-2 cells and in Caco-2/HT29-MTX cells.3 This suggests that even if some TiO2 particles are trapped in the mucus, some can also reach the epithelium as efficiently as in the absence of mucus. From a more general point of view, this increased release of mucins suggests enhanced protection of the epithelium. Furthermore, since mucus is a niche for bacteria, increasing the amount of mucus might increase the amount of commensal bacteria, which use mucins as attachment sites and as an energy source.58
We also reported that the repeated exposure to TiO2 downregulated KLF4, which controls the proliferation and differentiation of goblet cells.59 Consequently, HT29-MTX would be less proliferative and less differentiated. A reduced number of goblet cells has previously been demonstrated in mice orally exposed to E171.13 However, Talbot et al. recently showed that the sub-chronic oral exposure of rats to E171 had little or no impact on the quantity and quality of intestinal mucus, and concluded that the protective function of mucus remained intact.28 Therefore, this suggests that the reduced number of goblet cells reported by Urrutia-Ortega et al. would have a limited physiological consequence for healthy persons.
Epithelial homeostasis is preserved following repeated exposure to TiO2, with tight junctions remaining intact, as demonstrated by maintenance of the high transepithelial resistance. This finding concurs with our previous study performed with TiO2-NPs,25 which showed that they did not alter the TEER in vitro, while causing significant increase in the paracellular permeability in vivo. Moreover, an increase in efflux pump levels was observed in cells exposed to E171 and TiO2-NPs, as previously demonstrated in Caco-2 cells exposed to other types of TiO2-NPs.39 These pumps are gatekeepers in the intestine;60 thus a continuous exposure to ingested TiO2 could enhance the ability of intestinal epithelium to exclude potentially harmful substances, resulting in increased resistance toward xenobiotics.
Overall, as previously discussed for other endpoints,3 the impact of TiO2 particles on Caco-2/HT29-MTX is limited. E171 and P25 have a higher impact than A12, and this can be explained by the very low intracellular accumulation of A12, which is close to zero.3 Both E171 and P25 induce an inflammatory response and increase the secretion of mucus, suggesting that these cellular responses are triggered when the intracellular content of TiO2 reaches and exceeds a certain threshold. Regarding efflux pumps, we can speculate that the subtle differences observed in cells exposed to E171 compared to P25 can be explained by their different primary diameters or agglomerate sizes, and/or by their different crystal structures and/or by the different biocorona that form on their surface.
In the intestine, stem cells proliferate in the crypts of Lieberkühn to generate precursors of all the cellular lineages of the intestine.61 Precursors of enterocytes divide and then stop proliferating while moving upward along the crypt-villus axis, where their differentiation occurs. Finally villi are covered by fully-differentiated, post-mitotic cells, which are exposed to the intestinal luminal content before being exfoliated from the villus tip after 2 to 7 days.62 The chronic exposure scenario used here thereby mimics the chronic exposure received by an intestinal cell from the first day of its growth to the end of its differentiation, along the crypt-villus axis. Although in vivo this process is very rapid, in vitro full differentiation is obtained 21 days post-confluence, which is why this exposure duration was chosen here. However, one bias of this study is that the repeated exposure to NPs, twice a week for 3 weeks, implies that NPs progressively settle down on cells and are not washed out when the exposure medium is renewed. As a consequence, the quantity of NPs trapped in the mucus gel and in close contact with the cells could progressively increase from day 3 to day 21, and may be reaching a high level at the end of the exposure period. On top of HT29-MTX cells, TiO2 particles would be trapped in mucus islands.28 Conversely, on top of Caco-2 cells, which are not protected by mucus, agglomerates of TiO2 can cause damage to epithelial cells, including disruption of the brush border, because of the mass of TiO2 that settles down on the microvilli,63 but also because of the movement of TiO2 agglomerates in the vicinity of the microvilli, as previously demonstrated.4 This bias could be avoided by using inverted cultures or microgravity reactors.4,63 Nevertheless, even in the inverted configuration, TiO2 food additive is accumulated in Caco-2 cells and causes a disruption of the brush border,4 which shows that the impact of TiO2 particles is not only due to mechanical stress. Consequently, it is probable that some effects observed in this study would also occur in the inverted configuration. Another bias of this study is that in vitro cells were exposed to particles without any food matrix and microbiota, which differs from the situation where particles are orally absorbed during a meal. This could change the fate and impact of these particles, as previously demonstrated by others,64,65 and should be considered in future studies.
Conclusions
Our results show that repeated in vitro exposure of intestinal epithelial cells to TiO2 does not affect the differentiation of enterocytes, while it induces an inflammatory response. Mucus secretion is also modified, with the increased release of mucins. Finally, exposure to TiO2 does not directly impair epithelial homeostasis, but moderately increases the expression of some efflux pumps, members of the ABC family of transporters, exporting xenobiotics as well as chemotherapeutic agents from intestinal cells. Therefore, TiO2 particles, both micro- and nano-scaled, induce a moderate but significant dysregulation of multiple markers of the intestinal barrier function, probably mediated by the secretion of inflammatory cytokines, which rather evokes a protective response of the epithelium in vitro.
Funding sources
This work was supported by the French Atomic Energy and Alternative Energies Commission (CEA) through the ‘Toxicology’ research program (IMAGINATOX grant), the French Environment and Energy Management Agency (ADEME), the French Agency for Food, Environmental and Occupational Health & Safety (ANSES) within the framework of the National Research Program for Environmental and Occupational Health (PNR-EST, EST-2013/1/024, NanoGut grant) and the French National Research Agency (ANR, ANR-16-CE34-0011-01, PAIPITO grant). It is a contribution to the Labex Serenade (ANR-11-LABX-0064) funded by the French Government's “Investissements d'Avenir” ANR program, through the A*MIDEX project (ANR-11-IDEX-0001-02).
Authors' contributions
MD performed experiments and wrote the manuscript, DB contributed to RT-qPCR experiments, CT analyzed cellular differentiation, CMD analyzed the Luminex data, MDu performed the Luminex experiment, FB and TR critically assessed and interpreted the inflammation data and the study as a whole, EH critically interpreted the study as a whole, NHB performed the physico-chemical characterization of E171 and TiO2-NPs and synthesized A12, MC designed the whole study and was a major contributor to writing the manuscript.
Conflicts of interest
There are no conflicts to declare.
Acknowledgements
The authors would like to thank T. Lesuffleur (INSERM U938, Paris, France) for the generous gift of HT29-MTX cells and Maighread Gallagher-Gambarelli for advice on English usage.
References
- H. Shi, R. Magaye, V. Castranova and J. Zhao, Titanium dioxide nanoparticles: a review of current toxicological data, Part. Fibre Toxicol., 2013, 10, 15 CrossRef CAS PubMed.
-
European Commission, Recommendation on the definition of a nanomaterial, Official Journal of the European Union, 2011, 2011/696/EU Search PubMed.
- M. Dorier, D. Beal, C. Marie-Desvergne, M. Dubosson, F. Barreau, E. Houdeau, N. Herlin-Boime and M. Carriere, Continuous in vitro exposure of intestinal epithelial cells to E171 food additive causes oxidative stress, inducing oxidation of DNA bases but no endoplasmic reticulum stress, Nanotoxicology, 2017, 11, 751–761 CAS.
- J. J. Faust, K. Doudrick, Y. Yang, P. Westerhoff and D. G. Capco, Food grade titanium dioxide disrupts intestinal brush border microvilli in vitro independent of sedimentation, Cell Biol. Toxicol., 2014, 30, 169–188 CrossRef CAS PubMed.
- A. Weir, P. Westerhoff, L. Fabricius, K. Hristovski and N. von Goetz, Titanium dioxide nanoparticles in food and personal care products, Environ. Sci. Technol., 2012, 46, 2242–2250 CrossRef CAS PubMed.
- Y. Yang, K. Doudrick, X. Bi, K. Hristovski, P. Herckes, P. Westerhoff and R. Kaegi, Characterization of food-grade titanium dioxide: the presence of nanosized particles, Environ. Sci. Technol., 2014, 48, 6391–6400 CrossRef CAS PubMed.
- H. Bouwmeester, M. van der Zande and M. A. Jepson, Effects of food-borne nanomaterials on gastrointestinal tissues and microbiota, Wiley Interdiscip. Rev.: Nanomed. Nanobiotechnol., 2018, 10 DOI:10.1002/wnan.1481.
- EFSA, ANS Panel, Scientific Opinion on the re-evaluation of titanium dioxide (E 171) as a food additive, EFSA J., 2016, 14, 4545–4628 Search PubMed.
- C. Rompelberg, M. B. Heringa, G. van Donkersgoed, J. Drijvers, A. Roos, S. Westenbrink, R. Peters, G. van Bemmel, W. Brand and A. G. Oomen, Oral intake of added titanium dioxide and its nanofraction from food products, food supplements and toothpaste by the Dutch population, Nanotoxicology, 2016, 10, 1404–1414 CrossRef CAS PubMed.
- D. B. Warheit, R. Boatman and S. C. Brown, Developmental toxicity studies with 6 forms of titanium dioxide test materials (3 pigment-different grade & 3 nanoscale) demonstrate an absence of effects in orally-exposed rats, Regul. Toxicol. Pharmacol., 2015, 73, 887–896 CrossRef CAS PubMed.
- D. B. Warheit, S. C. Brown and E. M. Donner, Acute and subchronic oral toxicity studies in rats with nanoscale and pigment grade titanium dioxide particles, Food Chem. Toxicol., 2015, 84, 208–224 CrossRef CAS PubMed.
- D. B. Warheit and E. M. Donner, Risk assessment strategies for nanoscale and fine-sized titanium dioxide particles: Recognizing hazard and exposure issues, Food Chem. Toxicol., 2015, 85, 138–147 CrossRef CAS PubMed.
- I. M. Urrutia-Ortega, L. G. Garduno-Balderas, N. L. Delgado-Buenrostro, V. Freyre-Fonseca, J. O. Flores-Flores, A. Gonzalez-Robles, J. Pedraza-Chaverri, R. Hernandez-Pando, M. Rodriguez-Sosa, S. Leon-Cabrera, L. I. Terrazas, H. van Loveren and Y. I. Chirino, Food-grade titanium dioxide exposure exacerbates tumor formation in colitis associated cancer model, Food Chem. Toxicol., 2016, 93, 20–31 CrossRef CAS PubMed.
- S. Bettini, E. Boutet-Robinet, C. Cartier, C. Comera, E. Gaultier, J. Dupuy, N. Naud, S. Tache, P. Grysan, S. Reguer, N. Thieriet, M. Refregiers, D. Thiaudiere, J. P. Cravedi, M. Carriere, J. N. Audinot, F. H. Pierre, L. Guzylack-Piriou and E. Houdeau, Food-grade TiO2 impairs intestinal and systemic immune homeostasis, initiates preneoplastic lesions and promotes aberrant crypt development in the rat colon, Sci. Rep., 2017, 7, 40373 CrossRef CAS PubMed.
- H. Proquin, M. J. Jetten, M. C. M. Jonkhout, L. G. Garduno-Balderas, J. J. Briede, T. M. de Kok, Y. I. Chirino and H. van Loveren, Gene expression profiling in colon of mice exposed to food additive titanium dioxide (E171), Food Chem. Toxicol., 2018, 111, 153–165 CrossRef CAS PubMed.
- H. Proquin, C. Rodriguez-Ibarra, C. G. Moonen, I. M. Urrutia Ortega, J. J. Briede, T. M. de Kok, H. van Loveren and Y. I. Chirino, Titanium dioxide food additive (E171) induces ROS formation and genotoxicity: contribution of micro and nano-sized fractions, Mutagenesis, 2017, 32, 139–149 CrossRef CAS PubMed.
- F. Sommer and F. Backhed, The gut microbiota - masters of host development and physiology, Nat. Rev. Microbiol., 2013, 11, 227–238 CrossRef CAS PubMed.
- M. A. McGuckin, S. K. Linden, P. Sutton and T. H. Florin, Mucin dynamics and enteric pathogens, Nat. Rev. Microbiol., 2011, 9, 265–278 CrossRef CAS PubMed.
- K. R. Groschwitz and S. P. Hogan, Intestinal barrier function: molecular regulation and disease pathogenesis, J. Allergy Clin. Immunol., 2009, 124, 3–20 CrossRef CAS PubMed ; quiz 21-22.
- E. M. Leslie, R. G. Deeley and S. P. C. Cole, Multidrug resistance proteins: role of P-glycoprotein, MRP1, MRP2, and BCRP (ABCG2) in tissue defense, Toxicol. Appl. Pharmacol., 2005, 204, 216–237 CrossRef CAS PubMed.
- A. M. Mowat, Anatomical basis of tolerance and immunity to intestinal antigens, Nat. Rev. Immunol., 2003, 3, 331–341 CrossRef CAS PubMed.
- L. Antoni, S. Nuding, J. Wehkamp and E. F. Stange, Intestinal barrier in inflammatory bowel disease, World J. Gastroenterol., 2014, 20, 1165–1179 CrossRef PubMed.
- A. N. Ananthakrishnan, Epidemiology and risk factors for IBD, Nat. Rev. Gastroenterol. Hepatol., 2015, 12, 205–217 CrossRef PubMed.
- M. C. Lomer, R. P. Thompson and J. J. Powell, Fine and ultrafine particles of the diet: influence on the mucosal immune response and association with Crohn's disease, Proc. Nutr. Soc., 2002, 61, 123–130 CrossRef PubMed.
- E. Brun, F. Barreau, G. Veronesi, B. Fayard, S. Sorieul, C. Chaneac, C. Carapito, T. Rabilloud, A. Mabondzo, N. Herlin-Boime and M. Carriere, Titanium dioxide nanoparticle impact and translocation through ex vivo, in vivo and in vitro gut epithelia, Part. Fibre Toxicol., 2014, 11, 13 CrossRef PubMed.
- C. Gitrowski, A. R. Al-Jubory and R. D. Handy, Uptake of different crystal structures of TiO2 nanoparticles by Caco-2 intestinal cells, Toxicol. Lett., 2014, 226, 264–276 CrossRef CAS PubMed.
- E. Y. Chen, M. Garnica, Y. C. Wang, C. S. Chen and W. C. Chin, Mucin secretion induced by titanium dioxide nanoparticles, PLoS One, 2011, 6, e16198 CrossRef CAS PubMed.
- P. Talbot, J. M. Radziwill-Bienkowska, J. B. J. Kamphuis, K. Steenkeste, S. Bettini, V. Robert, M. L. Noordine, C. Mayeur, E. Gaultier, P. Langella, C. Robbe-Masselot, E. Houdeau, M. Thomas and M. Mercier-Bonin, Food-grade TiO2 is trapped by intestinal mucus in vitro but does not impair mucin O-glycosylation and short-chain fatty acid synthesis in vivo: implications for gut barrier protection, J. Nanobiotechnol., 2018, 16, 53 CrossRef PubMed.
- T. Waller, C. Chen and S. L. Walker, Food and Industrial Grade Titanium Dioxide Impacts Gut Microbiota, Environ. Eng. Sci., 2017, 34, 537–550 CrossRef CAS.
- H. Q. Chen, R. F. Zhao, B. Wang, C. X. Cai, L. N. Zheng, H. L. Wang, M. Wang, H. Ouyang, X. Y. Zhou, Z. F. Chai, Y. L. Zhao and W. Y. Feng, The effects of orally administered Ag, TiO2 and SiO2 nanoparticles on gut microbiota composition and colitis induction in mice, NanoImpact, 2017, 8, 80–88 CrossRef.
- W. Dudefroi, K. Moniz, E. Allen-Vercoe, M. H. Ropers and V. K. Walker, Impact of food grade and nano-TiO2 particles on a human intestinal community, Food Chem. Toxicol., 2017, 106, 242–249 CrossRef PubMed.
- B. C. Schanen, A. S. Karakoti, S. Seal, D. R. Drake, W. L. Warren and W. T. Self, Exposure to Titanium Dioxide Nanomaterials Provokes Inflammation of an in Vitro Human Immune Construct, ACS Nano, 2009, 3, 2523–2532 CrossRef CAS PubMed.
- M. Giovanni, J. Q. Yue, L. F. Zhang, J. P. Xie, C. N. Ong and D. T. Leong, Pro-inflammatory responses of RAW264.7 macrophages when treated with ultralow concentrations of silver, titanium dioxide, and zinc oxide nanoparticles, J. Hazard. Mater., 2015, 297, 146–152 CrossRef CAS PubMed.
- C. Y. Tay, W. Fang, M. I. Setyawati, S. L. Chia, K. S. Tan, C. H. Hong and D. T. Leong, Nano-hydroxyapatite and nano-titanium dioxide exhibit different subcellular distribution and apoptotic profile in human oral epithelium, ACS Appl. Mater. Interfaces, 2014, 6, 6248–6256 CrossRef CAS PubMed.
- T. Lesuffleur, A. Barbat, E. Dussaulx and A. Zweibaum, Growth adaptation to methotrexate of HT-29 human colon carcinoma cells is associated with their ability to differentiate into columnar absorptive and mucus-secreting cells, Cancer Res., 1990, 50, 6334–6343 CAS.
- E. Walter, S. Janich, B. J. Roessler, J. M. Hilfinger and G. L. Amidon, HT29-MTX/Caco-2 cocultures as an in vitro model for the intestinal epithelium: In vitro in vivo correlation with permeability data from rats and humans, J. Pharm. Sci., 1996, 85, 1070–1076 CrossRef CAS PubMed.
-
EFSA, ANS Panel, Re-evaluation of titanium dioxide (E171) as a food additive, EFSA J., 2016, vol. 14, pp. 4545–4628 Search PubMed.
- B. Pignon, H. Maskrot, V. G. Ferreol, Y. Leconte, S. Coste, M. Gervais, T. Pouget, C. Reynaud, J. F. Tranchant and N. Herlin-Boime, Versatility of laser pyrolysis applied to the synthesis of TiO2 nanoparticles - Application to UV attenuation, Eur. J. Inorg. Chem., 2008, 883–889, DOI:10.1002/ejic.200700990.
- M. Dorier, E. Brun, G. Veronesi, F. Barreau, K. Pernet-Gallay, C. Desvergne, T. Rabilloud, C. Carapito, N. Herlin-Boime and M. Carriere, Impact of anatase and rutile titanium dioxide nanoparticles on uptake carriers and efflux pumps in Caco-2 gut epithelial cells, Nanoscale, 2015, 7, 7352–7360 RSC.
-
J. F. Forstner and G. G. Forstner, in Physiology of the gastrointestinal tract, ed. L. R. Johnson, Raven Press, New York, 3rd edn, 1994, pp. 1255–1283 Search PubMed.
- M. W. Pfaffl, A. Tichopad, C. Prgomet and T. P. Neuvians, Determination of stable housekeeping genes, differentially regulated target genes and sample integrity: BestKeeper--Excel-based tool using pair-wise correlations, Biotechnol. Lett., 2004, 26, 509–515 CrossRef CAS PubMed.
- M. W. Pfaffl, G. W. Horgan and L. Dempfle, Relative expression software tool (REST) for group-wise comparison and statistical analysis of relative expression results in real-time PCR, Nucleic Acids Res., 2002, 30, e36 CrossRef PubMed.
- B. S. Harvey, T. C. Sia, D. A. Wattchow and S. D. Smid, Interleukin 17A evoked mucosal damage is attenuated by cannabidiol and anandamide in a human colonic explant model, Cytokine+, 2014, 65, 236–244 CrossRef CAS PubMed.
- D. Wei, M. Kanai, S. Huang and K. Xie, Emerging role of KLF4 in human gastrointestinal cancer, Carcinogenesis, 2006, 27, 23–31 CrossRef CAS PubMed.
- M. G. Smirnova, L. Guo, J. P. Birchall and J. P. Pearson, LPS up-regulates mucin and cytokine mRNA expression and stimulates mucin and cytokine secretion in goblet cells, Cell. Immunol., 2003, 221, 42–49 CrossRef CAS PubMed.
- T. Hosoi, R. Hirose, S. Saegusa, A. Ametani, K. Kiuchi and S. Kaminogawa, Cytokine responses of human intestinal epithelial-like Caco-2 cells to the nonpathogenic bacterium Bacillus subtilis (natto), Int. J. Food Microbiol., 2003, 82, 255–264 CrossRef CAS PubMed.
- C. Rodriguez-Juan, M. Perez-Blas, A. P. Valeri, N. Aguilera, A. Arnaiz-Villena, A. Pacheco-Castro and J. M. Martin-Villa, Cell surface phenotype and cytokine secretion in Caco-2 cell cultures: increased RANTES production and IL-2 transcription upon stimulation with IL-1beta, Tissue Cell, 2001, 33, 570–579 CrossRef CAS PubMed.
- M. Akdis, S. Burgler, R. Crameri, T. Eiwegger, H. Fujita, E. Gomez, S. Klunker, N. Meyer, L. O'Mahony, O. Palomares, C. Rhyner, N. Ouaked, A. Schaffartzik, W. Van De Veen, S. Zeller, M. Zimmermann and C. A. Akdis, Interleukins, from 1 to 37, and interferon-gamma: receptors, functions, and roles in diseases, J. Allergy Clin. Immunol., 2011, 127, 701–721.e701-770 CrossRef CAS PubMed.
- S. Bogaert, D. Laukens, H. Peeters, L. Melis, K. Olievier, N. Boon, G. Verbruggen, J. Vandesompele, D. Elewaut and M. De Vos, Differential mucosal expression of Th17-related genes between the inflamed colon and ileum of patients with inflammatory bowel disease, BMC Immunol., 2010, 11, 61 CrossRef CAS PubMed.
- E. A. Garcia-Zepeda, M. E. Rothenberg, R. T. Ownbey, J. Celestin, P. Leder and A. D. Luster, Human eotaxin is a specific chemoattractant for eosinophil cells and provides a new mechanism to explain tissue eosinophilia, Nat. Med., 1996, 2, 449–456 CrossRef CAS PubMed.
- C. Monteiller, L. Tran, W. MacNee, S. Faux, A. Jones, B. Miller and K. Donaldson, The pro-inflammatory effects of low-toxicity low-solubility particles, nanoparticles and fine particles, on epithelial cells in vitro: the role of surface area, Occup. Environ. Med., 2007, 64, 609–615 CrossRef CAS PubMed.
- T. E. Abbott Chalew and K. J. Schwab, Toxicity of commercially available engineered nanoparticles to Caco-2 and SW480 human intestinal epithelial cells, Cell Biol. Toxicol., 2013, 29, 101–116 CrossRef CAS PubMed.
- I. De Angelis, F. Barone, A. Zijno, L. Bizzarri, M. T. Russo, R. Pozzi, F. Franchini, G. Giudetti, C. Uboldi, J. Ponti, F. Rossi and B. De Berardis, Comparative study of ZnO and TiO2 nanoparticles: physicochemical characterisation and toxicological effects on human colon carcinoma cells, Nanotoxicology, 2013, 7, 1361–1372 CrossRef CAS PubMed.
- K. Gerloff, D. I. Pereira, N. Faria, A. W. Boots, J. Kolling, I. Forster, C. Albrecht, J. J. Powell and R. P. Schins, Influence of simulated gastrointestinal conditions on particle-induced cytotoxicity and interleukin-8 regulation in differentiated and undifferentiated Caco-2 cells, Nanotoxicology, 2013, 7, 353–366 CrossRef CAS PubMed.
- R. M. Fusaro and R. W. Goltz, A comparative study of the periodic acid-Schiff and alcian blue stains, J. Invest. Dermatol., 1960, 35, 305–307 CrossRef CAS PubMed.
- R. Bansil and B. S. Turner, The biology of mucus: Composition, synthesis and organization, Adv. Drug Delivery Rev., 2018, 124, 3–15 CrossRef CAS PubMed.
- M. A. McGuckin, R. Eri, L. A. Simms, T. H. Florin and G. Radford-Smith, Intestinal barrier dysfunction in inflammatory bowel diseases, Inflamm. Bowel Dis., 2009, 15, 100–113 CrossRef PubMed.
- M. E. V. Johansson, D. Ambort, T. Pelaseyed, A. Schutte, J. K. Gustafsson, A. Ermund, D. B. Subramani, J. M. Holmen-Larsson, K. A. Thomsson, J. H. Bergstrom, S. van der Post, A. M. Rodriguez-Pineiro, H. Sjovall, M. Backstrom and G. C. Hansson, Composition and functional role of the mucus layers in the intestine, Cell. Mol. Life Sci., 2011, 68, 3635–3641 CrossRef CAS PubMed.
- H. Clevers, Wnt/beta-catenin signaling in development and disease, Cell, 2006, 127, 469–480 CrossRef CAS PubMed.
- C. G. Dietrich, A. Geier and R. Elferink, ABC of oral bioavailability: transporters as gatekeepers in the gut, Gut, 2003, 52, 1788–1795 CrossRef CAS PubMed.
- H. Clevers, The intestinal crypt, a prototype stem cell compartment, Cell, 2013, 154, 274–284 CrossRef CAS PubMed.
- C. Crosnier, D. Stamataki and J. Lewis, Organizing cell renewal in the intestine: stem cells, signals and combinatorial control, Nat. Rev. Genet., 2006, 7, 349–359 CrossRef CAS PubMed.
- E. C. Cho, Q. Zhang and Y. Xia, The effect of sedimentation and diffusion on cellular uptake of gold nanoparticles, Nat. Nanotechnol., 2011, 6, 385–391 CrossRef CAS PubMed.
- J. W. Richter, G. M. Shull, J. H. Fountain, Z. Guo, L. P. Musselman, A. C. Fiumera and G. J. Mahler, Titanium dioxide nanoparticle exposure alters metabolic homeostasis in a cell culture model of the intestinal epithelium and Drosophila melanogaster, Nanotoxicology, 2018, 12, 390–406 CrossRef CAS PubMed.
- Z. M. Song, N. Chen, J. H. Liu, H. Tang, X. Deng, W. S. Xi, K. Han, A. Cao, Y. Liu and H. Wang, Biological effect of food additive titanium dioxide nanoparticles on intestine: an in vitro study, J. Appl. Toxicol., 2015, 35, 1169–1178 CrossRef CAS PubMed.
Footnote |
† Electronic supplementary information (ESI) available. See DOI: 10.1039/c8en01188e |
|
This journal is © The Royal Society of Chemistry 2019 |
Click here to see how this site uses Cookies. View our privacy policy here.