Graphene oxide as a multifunctional synergist of insecticides against lepidopteran insect†
Received
17th August 2018
, Accepted 25th November 2018
First published on 26th November 2018
Abstract
Application of nanotechnology in plant protection via formulating nanomaterial-based pesticides to improve pesticide bioactivity has attracted significant research interest in recent years. In this study, the synergistic activity and mode of GO for insecticides against a lepidoptera insect Asian corn borer (ACB) were investigated. The results demonstrated excellent synergistic activities of GO for the three types of insecticides, namely, β-cyfluthrin (Cyf), Monosultap (Mon) and Imidacloprid (Imi). GO–Cyf, GO–Mon and GO–Imi mixtures exhibited 2.1-, 1.51- and 1.83-fold higher contact toxicities than the individual insecticides of Cyf, Mon and Imi, respectively. Our results indicated that the synergistic mechanism may involve the following phenomena: (i) GO can cause ‘physical damage’ to the cement layer of insects, resulting in rapid water loss for the insects, (ii) the disrupted cement layer provides a new channel for the penetration of insecticides, and (iii) the GO delivery system can enhance the efficacy of the insecticides. This multifunctional mechanism that allows GO to serve as a synergist for the existing insecticides demonstrates the excellent application potential of GO in pest management.
Environmental significance
The intensive and repeated use of pesticides has aroused increasing concerns about their negative impacts on the environment and humans. Therefore, simultaneous enhancement in the efficiency and decrease in the side effects of pesticides has been an important topic in both agricultural and environmental chemistry. Our study indicates that the multifunctional synergistic mechanism of GO with pesticides may involve the following phenomena: (i) GO can cause ‘physical damage’ to the cement layer of insects, resulting in a rapid water loss of the insects, (ii) the disrupted cement layer provides a new channel for the penetration of pesticides, and (iii) the GO delivery system can enhance the efficacy of the pesticides. Combination of the multifunctional synergistic effect of GO and the toxicity of pesticides may contribute to stronger insecticidal effects against both resistant and non-resistant pests, thus reducing environmental pollution caused by the frequent use of pesticides.
|
1. Introduction
Nanotechnology has applications in physics, chemistry, pharmaceutical science, material science, medicine, and agriculture due to the small size, high surface-to-volume ratio and unique optical properties of nanomaterials.1–3 Recently, with the development of a wide range of plant protection products, the potential application of nanotechnology in pesticides termed as ‘nanopesticides’ has attracted significant interest.4–8 Meanwhile, graphene oxide (GO) has also attracted considerable attention owing to its unique electronic, mechanical, and thermal properties.9–13 Currently, surface-modified GO has been shown to enhance the utilization efficiency of pesticides when used to deliver a variety of pesticides.14,15 For instance, it has been reported that GO decorated with copper selenide can hold 40% (w/w) of the pesticide and remain as a reservoir in the leaf without any drift loss, thus enhancing Pieris rapae larval mortality by more than 35% through the mechanism of reduced drift loss and targeted release.14 Tong et al. revealed that polydopamine-coated GO can serve as a pesticide carrier to enhance utilization efficiency and reduce wash-off, especially for water-soluble pesticides.15 In such research, GO was only used for the single function of pesticide delivery to enhance the efficiency of the pesticide. However, limited information is available about other functions of GO as a synergist to enhance the bioactivity of pesticides.
Insecticides are extensively used in agriculture to boost agricultural productivity as they are considered as the most effective way for the prevention and management of important agricultural pests.16 β-Cyfluthrin (Cyf), Monosultap (Mon) and Imidacloprid (Imi) are three different types of insecticides that are registered globally for the control of a wide range of pests.17 However, the intensive and repeated use of these insecticides has become increasingly restricted due to the developing insect resistance and residual on the non-target organisms, which eventually leads to invalid control of the target insects.18,19 One facile approach to solve these problems is the combined use of existing insecticides with other novel materials such as GO to formulate new GO–insecticides with different modes of action against pests.
Thus, for better practical applications of GO–insecticide nanocomposites, in this study, we aim to fabricate a novel nanoformulation of GO-based insecticides and explore the potential synergistic effects of GO when combined with insecticides. Cyf is a nonsystemic, contact and stomach insecticide; Mon is a systemic, contact, and stomach insecticide, whereas Imi is a systemic but non-contact and non-stomach insecticide. Here, Cyf and Mon were used as the positive control, and Imi was used as the negative control to study the synergistic effect of GO on the contact activity of insecticides. An important lepidopteran insect Asian corn borer (ACB), namely, Ostrinia furnacalis (Guenée) was chosen as the model insect in the test. Synergistic effects of GO on the contact toxicity of Cyf, Mon, and Imi were evaluated in detail. Additionally, the multifunctional synergistic mode of GO for insecticides was illustrated. Our results demonstrate that the combination of GO with insecticides can result in highly potent insecticidal activity, and the findings may provide important implications for better design of graphene-based pesticides or other pesticide applications.
2. Experimental section
2.1 Materials
All chemicals were of analytical grade and were used as received without further purification. Graphite was purchased from Qingdao Tianhe Graphite Co. Ltd. with an average particle diameter of 4 mm (99.95% purity). All other reagents were obtained from the Tianjin No. 3 Chemical Plant. Cyf (analytical standard grade) and Imi (analytical standard grade) were purchased from Sigma-Aldrich. Mon (analytical standard grade) was purchased from Tian Jin Alta Scientific Co., Ltd.
2.2 Preparation and characterization of GO
Commercially available graphite powder was oxidized and exfoliated to GO by a modified Hummers method.20 Typically, the natural graphite powders were initially oxidized by concentrated sulfuric acid (H2SO4) to produce graphite oxide (GtO). The GtO sample was washed with HCl (10% v/v) and DI water to remove chemical residues, followed by centrifugal washing until neutrality to remove unexfoliated GtO and acids; it was finally subjected to dialysis with Spectra/Por3 dialysis membranes (molecular weight cut off 3500 Da) against deionized (DI) water for 7 days for additional purification. The DI water was changed every day. To obtain GO, the product was sonicated with a bath-sonicator (Elam-sonic S60H, 100 W) for 1 h, followed by centrifugation at 5000 rpm for 15 min to remove the unexfoliated GO.
Infrared absorption spectra of GO were measured on a Fourier transform infrared (FTIR) spectroscope (IRAffinity-1, Shimadzu, Japan) at room temperature. Transmission electron microscopy (TEM) was carried out on a Tecnai G20 microscope (FEI, Czech) with an accelerating voltage of 200 kV. The size and morphology of GO were investigated with a tapping mode atomic force microscope (AFM, Nanoscope IIIa, Digital Instrument Inc.). The particle stability and zeta potential of GO were measured using a Zetasizer Nano ZS90 dynamic light scattering (DLS) system (Malvern, England).
2.3 Insects and rearing
Asian corn borer (ACB) neonates used in this study were obtained from a laboratory colony maintained on a regular artificial diet for multiple generations in the Institute of Plant Protection, Chinese Academy of Agricultural Science. ACB larvae were reared on an artificial diet at 28 °C under relative humidity of 70–90% and a photoperiod of 16 h light and 8 h darkness.21
2.4 Pesticides
We used active ingredients in all test solutions. As mother dispersion, 100 mg of Cyf and Imi were first dispersed in 2 mL of acetone and then diluted to the final concentration 100 μg mL−1 with distilled water containing 0.1% Tween-80 as the surfactant. Then, two mother dispersions were obtained by 30 min of sonication using a sonicator bath at 37 kHz 100 W. The two mother dispersions were diluted to a series of concentrations with distilled water containing 0.1% Tween-80. Mon (50 mg) was dissolved in 100 mL distilled water to reach a final concentration of 500 μg mL−1 and then diluted to a series of concentrations with distilled water. Doses were chosen based on a mortality range of 0–100%.
2.5 Preparation of GO–insecticide formulation
Preliminary investigations showed that the combination of insecticide and GO at the ratio of 2
:
1 in dose exhibited the highest insecticidal activity. Thus, for synergism experiments, GO was mixed with different insecticides at this ratio. Briefly, 6.67 mg of pesticide (Cyf or Imi) dispersed in 2 mL of acetone and 0.1 mL Tween 80 solution was mixed with 3.33 mg of GO, which was then ultrasonicated in water (100 mL) and stirred for 24 h in the dark. Mon (6.67 mg) and GO (3.33 mg) were mixed and dissolved in 100 mL distilled water directly, which was then diluted to a series of concentrations with distilled water.
2.6 Contact toxicity of formulated insecticides
The synergistic effects of GO on the contact toxicity of Cyf, Mon, and Imi were examined. The third instar larva was placed on a Petri dish (twenty insects for each) with four replicates. The test insects were treated with 1.5 mL of individual insecticides and GO at different concentrations using a hand micro-applicator and maintained at 25 ± 2 °C with a 16/8 h light/dark photoperiod. The corresponding concentrations of insecticide–GO mixtures (Cyf–GO, Mon–GO, and Imi–GO) were used for comparison purposes. Insects treated with distilled water containing 2% acetone and 0.1% Tween-80 were used as the control for Cyf and Imi. Distilled water-treated insects served as the control for Mon. Initially, data were taken at intervals of 30 min for the first 2 h and subsequently at intervals of 8 h. A test insect was considered dead if it was unable to move and could not right itself when prodded with a probe. The mortality of the treated insects was recorded at 24 h post-treatment.22
2.7 Observation of structural changes in the morphology of ACB
To demonstrate how GO exerts synergistic effects on insecticides, SEM images were used to illustrate the dynamic changes in the ultra-structure of cement layer in response to GO synergism action. The third instar larva was treated with distilled water, GO, Cyf, Cyf–GO, Mon, Mon–GO, Imi, and Imi–GO. Then, the larva was fixed with 2.5% glutaraldehyde with a vacuum pump in an ice bath for 30 min, followed by 4 h of incubation at 4 °C and three times PBS washing. After that, the larva was post-fixed with 1% aqueous OsO4 (Fluka) for 1 h at 4 °C and washed twice with 0.1 mol L−1 pH 7.0 phosphate buffer. Subsequently, the larva was dehydrated with an ethanol series and examined under a scanning electron microscope (SEM, Japan JSM-6390).
2.8 Data analysis
Mortality data from the bioassays were corrected for control mortality using Abbott's formula.23 The corrected mortality was normalized by arcsine square-root transformation before being subjected to a one-way analysis of variance (ANOVA), followed by a least significant difference (LSD) test at P < 0.05 using SAS Institute (2002; SAS Institute, Cary, NC). The 95% confidence limits (CL) for each LC50 value were also determined using the same procedures. The χ2 value was used to measure the goodness-of-fit of the probit regression equation. To assess the degree of synergism, the synergistic ratio (SR) was calculated by dividing LC50 of the insecticide by LC50 of the insecticide plus synergist.24
3. Results and discussion
3.1 Preparation and characterization of GO
The functional groups of GO were investigated with FT-IR spectra, as shown in Fig. 1A. The absorption peak at 3417 cm−1 was ascribed to the stretching of O–H.25 The peaks at 1725, 1625 and 1399 cm−1 corresponded to carbonyl C
O stretching vibrations, aromatic C
C stretching, and carboxyl O
C–O stretching mode of the sp2 carbon skeletal network; the bands at 1221 and 1065 cm−1 were associated with C–O stretching of epoxy and alkoxy groups, respectively.26 The typical morphology of GO is displayed in Fig. 1B and the images of GO were observed using TEM. As can be seen, the free-standing two-dimensional GO sheets displayed flake-like shapes with high transparency and some wrinkles. The 3D dimension of GO is shown in Fig. 1C, and the single-layer GO was about 1–3 nm thick, as observed from the representative AFM images.
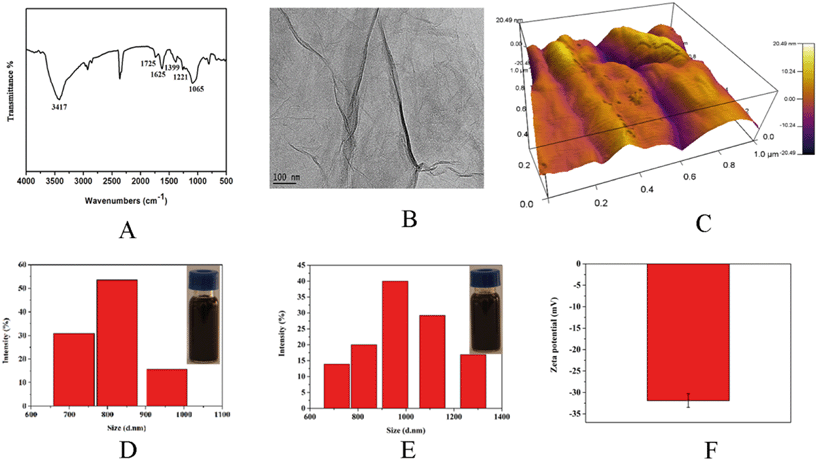 |
| Fig. 1 Characterization of GO. FT-IR spectra (A), TEM image (B), and AFM image (C). Dispersibility and stability of GO (D) and GO (E) after being stored for 14 days. The insets in D and E are the photos of GO before and after storage for 14 days. (F) Detection of the zeta potential of GO. | |
The particle stability of GO could also significantly influence the interaction between GO and organisms.27 The dispersibility and stability of GO were further characterized by DLS. DLS data indicated that the diameters of the particles in GO ranged from 712 nm to 955 nm (Fig. 1D). After storage for 14 days, the GO particle diameters increased and were in the range from 712 nm to 1280 nm (Fig. 1E). However, no stratification was observed in GO dispersion, suggesting that the stored GO solution was relatively stable. Besides, the zeta potential of GO was measured to be −31.9 mV (Fig. 1E).
3.2 Characterization of the formulated GO–insecticide
As shown in Fig. 2A, GO sheets were thin, transparent and smooth with small wrinkles. However, the GO–Cyf, GO–Mon, and GO–Imi surfaces (Fig. 2B–D) appeared to have some black spots, which were not observed on the surface of GO. It has been demonstrated that the surface of GO is generally favorable for the adsorption of molecules with poor water solubility via electrostatic attraction, hydrophobic interaction and π–π stacking.28 Considering the different forms of Cyf, Mon, and Imi, we speculated that the appearance of these black spots was due to the adsorption of Cyf, Mon, and Imi on GO sheets. As can be seen from Fig. 2, different numbers and shapes of black spots on GO may be due to differences in the dispersibilities of the insecticides.
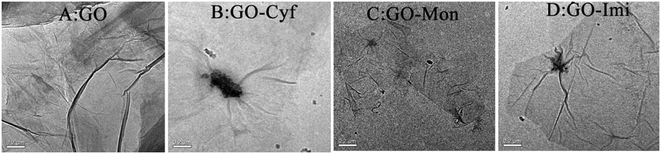 |
| Fig. 2 Characterization of the formulated GO–insecticides. TEM images of GO (A), GO–Cyf (B), GO–Mon (C) and GO–Imi (D). | |
3.3 Pest control of GO–insecticide
Synergistic effects of GO on the contact toxicities of Cyf, Mon, and Imi are shown in Fig. 3. No instances of death were observed for ACB treated with distilled water and distilled water containing 2% acetone and 0.1% Tween-80 at 24 h. Only 3.33% mortality was observed for ACB treated with 25 μg mL−1 GO, whereas treatments with other concentrations of GO did not cause death of ACB, indicating good biocompatibility of GO as a synergist. The mortality values of ACB under Cyf treatment were 38.33%, 48.65%, 60.11% and 60.67% at different tested concentrations. Even at the highest concentration, the mortality of ACB was only 60.67%. However, in contrast to both Cyf and GO, the Cyf–GO mixture showed higher efficacy in controlling ACB. Even at the lowest GO–Cyf concentration of 12.5 μg mL−1, the mortality reached 60%. At the highest GO–Cyf concentration of 100 μg mL−1, the mortality was as high as 90%. However, dosage as high as 100 μg mL−1 of single Cyf was required to achieve low control efficacy of the Cyf–GO mixture (mortality: 60%). These results show that GO has superior synergistic effect on the toxicity of Cyf. Furthermore, the synergistic effect of GO on the contact toxicity of Mon was also investigated. As shown in Fig. 3B, the mortality of ACB after treatment with GO was less than 5% at any tested concentration. Under treatment with various concentrations of Mon, the mortality of ACB varied from 33.6% to 55.4%. Particularly, after treatment with the GO–Mon mixture, the mortality of ACB varied from 53.7% to 80.1%. Compared with single Mon treatment, GO–Mon treatment increased the mortality of ACB by more than 20%, indicating that the toxicity of Mon increased significantly after combination with GO.
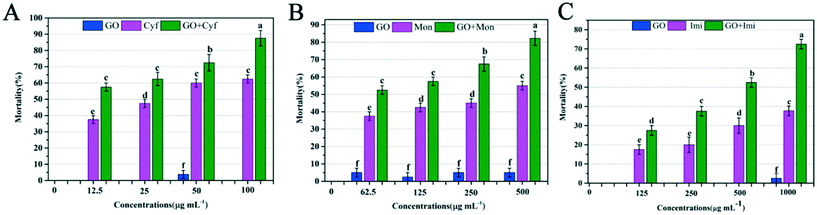 |
| Fig. 3 Synergistic effect of GO on the contact toxicity of Cyf, Mon, and Imi against ACB at 24 h. Percentage mortality of ACB caused by (A) GO, Cyf, and GO–Cyf, (B) GO, Mon, and GO–Mon, and (C) GO, Imi, and GO–Imi. Error bars represent the standard deviation (N = 4). Different letters above the error bars denote significant differences between treatments. | |
Moreover, the synergistic effect of GO on the insecticidal activity of Imi was also investigated. After treatment with various concentrations of Imi, the mortality of ACB varied from 16.7% to 37.4%. In contrast, treatment with GO–Imi mixtures increased the mortality to 26.37–72.6% (Fig. 3C). These results suggest that the supplementation of GO to Imi can improve insecticidal performance. It should be noted that Imi is a systemic pesticide that allows residues to be absorbed and then transported in the treated plants via xylem transport and translaminar (between leaf surfaces) movement.17 The contact toxicity effect of insecticides must be achieved by the penetration of insecticides through the cuticle of insects.29 In our experiment, there was no transhipment of plants because we treated the cuticle of ACB by GO–Imi directly. However, GO–Imi exhibited superior insecticidal activity, suggesting that GO can increase the penetration ability of Imi through the cuticle of insects.
In our experiment, 2% acetone and 0.1% Tween-80 were used as adjuvants to improve the dispersibility of Cyf and Imi. These adjuvants did not show any toxic effects on ACB compared with distilled water treatment (the control for Mon). In general, formulation of pesticides involves addition of various adjuvants to improve the ability of the pesticide to penetrate, target or protect the target organisms. For production, the active ingredient of GO–pesticide must be formulated with other adjuvants before use in enhancing the efficiency of pesticides.
To assess the synergistic effect of GO on insecticides, complete bioassays were performed to obtain the LC50 values for Cyf, Mon, and Imi against ACB (Table 1). LC50 is a basic parameter to evaluate the effect and synergism of the combination of synergist and insecticides.30 The lethal concentrations of individual insecticides and GO–insecticide mixtures for ACB larvae are shown in Table 1. The observed effects were expressed as LC50 values derived from probit analysis with 95% confidence limit. When applied to ACB third-instar larvae, the LC50 value of single Cyf was 1.32 μg mL−1, whereas that of Cyf–GO mixture was 0.62 μg mL−1, and SR was 2.10, indicating that the Cyf–GO mixture was 2.10-fold more potent than single Cyf.
Table 1 Synergistic effects of GO on the contact toxicity of Cyf, Mon, and Imi against ACB at 24 h
Treatment |
Slope ± SEa |
χ2b |
df
|
P
|
LC50 (μg mL−1) (95% CL)c |
SRd |
Slope of the probit mortality line.
Goodness-of-fit test.
LC50 values and 95% confidence limits (CL).
Synergism ratio at LC50 values.
|
Cyf |
0.79 ± 0.30 |
45.18 |
4 |
0.0001 |
1.32(1.02–1.63) |
— |
Cyf + GO |
0.82 ± 0.12 |
60.06 |
4 |
0.0001 |
0.62(0.55–1.02) |
2.10 |
Mon |
0.68 ± 0.22 |
41.20 |
4 |
0.0001 |
1.96(1.69–2.24) |
— |
Mon + GO |
0.73 ± 0.34 |
57.89 |
4 |
0.0001 |
1.29(1.22–2.01) |
1.51 |
Imi |
0.31 ± 0.10 |
12.94 |
4 |
0.0116 |
4.23(3.20–5.26) |
— |
Imi + GO |
1.29 ± 0.08 |
44.28 |
4 |
0.0001 |
2.31(2.27–2.45) |
1.83 |
The LC50 values of Mon and Imi were 1.96 and 4.23 μg mL−1, whereas those of Mon–GO and Imi–GO mixtures were 1.29 and 2.31 μg mL−1, respectively, showing that the LC50 value significantly decreased after the supplementation of GO. The SR values for the LC50 values of the Mon–GO and Imi–GO mixtures were 1.51 and 1.83, respectively, indicating 1.51-fold and 1.83-fold increase in insecticidal activity compared with that for single Mon and Imi, respectively. When the insecticidal activities were compared by a t-test, all mixtures demonstrated statistically significant differences from individual insecticides (P < 0.05), corroborating the conclusion that all the assayed combinations acted synergistically. These results suggest that the combination of GO and insecticides can reduce the use of insecticides and improve the pest control effect.
3.4 Effect of GO on larval cuticle
The cuticle is a barrier between the insect and the environment, and exogenous chemicals such as pesticides can penetrate the epidermis under certain conditions.31 The cement layer is the outermost layer of the cuticle of insects. As shown in the SEM images in Fig. 4A, after 4 h of distilled water treatment, the cement layer of the cuticle was in a neat arrangement. After 8 h of distilled water treatment, the cuticular cement layer showed slight embossment (Fig. 4B). After 24 h of distilled water treatment, the cement layer began to shrink and wrinkle (Fig. 4C). These results showed that with the extension of treatment time, the cuticle gradually shrank. Under GO treatment (Fig. 4D–F), the shrinking of the cuticle was more clear when compared with that of the control. Compared with Cyf (Fig. 4G–I), Mon (Fig. 4M–O) and Imi (Fig. 4S–U) treatments, Cyf–GO (Fig. 4J–L), Mon–GO (Fig. 4P–R) and Imi–GO treatments (Fig. 4V and W) resulted in more clear wrinkling of the cuticle as well as more severe damage to the cement layer structure. These findings suggested that the combination of GO with insecticides can cause more serious wrinkling of the cuticle compared with single insecticides. Cyf, Mon and Imi are neurotoxic agents, and the typical poisoning symptoms of insects for these three pesticides are insect exciting, convulsing, paralysis, and death.32 There is limited information about the effects of insecticides on the structure and morphology of the cuticle. However, for the effects of insecticides on the cuticle of insects, our results are consistent with those of a previous study, in which the larvae of Mythmina separata were treated with organophosphorus, neonicotinoid, phenylpyrazole and oxadiazine insecticides, and the integument of the larvae shrank.33 Moreover, we believe that the effect of GO–insecticides on the cuticle of insects is dependent on the structure of cuticle and the types of insecticides, which will be the subject of our follow-up study.
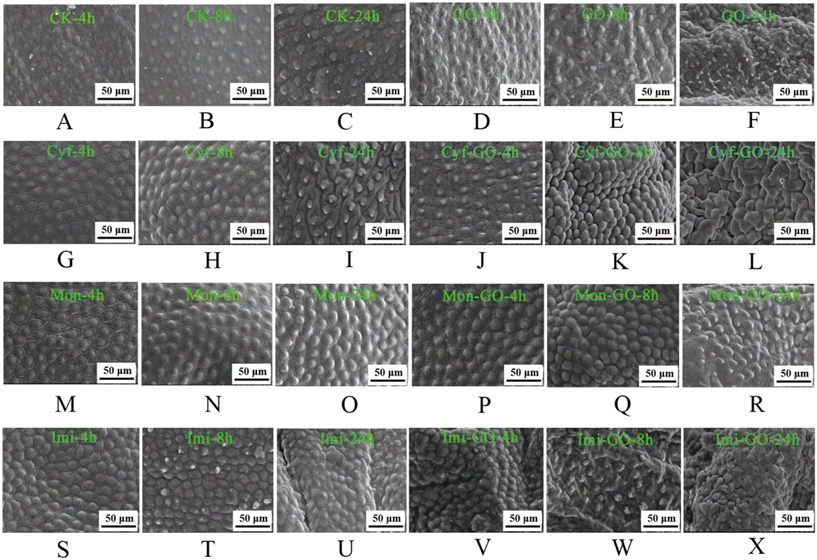 |
| Fig. 4 SEM images of the structural changes in the morphology of ACB cuticle induced by insecticides, GO–insecticide mixtures, and GO. Cuticles of control (A–C), GO-treated (D–F), Cyf-treated (G–I), Cyf–GO-treated (J–L), Mon-treated (M–O), Mon–GO-treated (P–R), Imi-treated (S–U), and Imi–GO-treated ACB (V–X) after treatment for 4 h, 8 h, and 24 h, respectively. | |
Evidently, more than cuticle wrinkling or shrinking, we observed coalescence of the cuticle, as shown in Fig. 4J, L, P and Q. Through observation and analysis of the SEM images, we assumed that there are three processes of change in the cuticle in the interaction between ACB and GO–insecticide: coalescence, followed by shrinkage and finally destruction of the cuticle structure. Because of the different insecticide treatments, different response rates of ACB were observed to the three GO–insecticides; due to limited SEM sampling time point, the captured images were also different. Therefore, coalescence of the cuticle was also found (Fig. 4J and L, P and Q). As can be seen from Fig. 4, the reaction rate for ACB with Imi–GO was faster than that with Mon–GO and Cyf–GO. Therefore, the cuticle of ACB showed more clear shrinking at the observed time point, whereas most of the images of ACB treated with Mon–GO and Cyf–GO showed coalescence of the cuticle.
According to our experiments, GO has very weak or no insecticidal activity. Thus, the enhanced insecticidal activity after the mixing of GO and insecticides can be ascribed to the synergistic effect of GO with insecticides. The cuticle is one of the main barriers to the movement of water, ions, and other substances between the internal and exterior tissues of insects.34 Generally, the wrinkling and shrinking of cuticle may be related to water loss in insects.35 It has been reported that the loss of a large proportion of water in many insects occurs via the cuticle due to cuticular damage.36 A previous study reported that the arrangement of cement layer in the cuticle of insects can be disrupted with fine mineral powder to increase the water evaporation rate, thus causing the death of insects.37 Moreover, it has been shown that artificial abrasion of the cuticle can increase the water loss rate of insects.38 Thus, we speculate that one reason for the enhancing effect of GO on insecticide toxicity is that GO can damage the cuticle structure in insects, leading to rapid water loss for the insects. On the other hand, the damage of cuticle induced by GO can provide a new channel as an ‘easy access’ for insecticides to penetrate through the cuticle. The prerequisite for mechanical damage to the cuticle of insects is that GO can be adsorbed onto the cuticle. To prove this possibility, we further examined the behaviors of GO on the cuticle of insects.
3.5 GO can be adsorbed on the cement layer of cuticle of ACB
Fig. S1† shows the SEM images of the interactions between GO and ACB. We did not observe adsorption of GO on the cuticle of ACB (Fig. S1B†) when compared with that for the control (Fig. S1A†). We speculate that the elution process for SEM slicing might have led to the removal of GO from the cuticle of ACB. This phenomenon may be ascribed to the cuticle structure of ACB. In another experiment reported by our group, GO was used to treat mites (the same treatment as ACB) and the adsorption behavior of GO on the cuticle of mites was observed by SEM (data not shown). The process for SEM slicing was the same as that of ACB. GO could be adsorbed on the cuticle of mites, as observed by SEM. Most of the small-sized GO remained on the ravine-like cuticle of mites, whereas the cuticle of the ACB was relatively smooth, which did not allow much GO retention. Therefore, the reason for no observation of GO by SEM may be due to the smooth structure of the ACB cuticle. However, we could still evaluate the interactions between GO and ACB using the changes in the color of ACB cuticle after treatment with GO. The color of GO dispersion was brown, whereas that of the larval cuticle of ACB was white. When ACB was treated with GO and when GO was adsorbed on the cuticle of ACB, the color of the cuticle became darker. Therefore, to determine whether GO could be adsorbed on the cuticle of ACB, a dissecting microscope was used to observe the color changes of the ACB cuticle. Fig. S1D† shows that the cuticle color of ACB treated with GO is brown, and it was close to the color of GO dispersion (Fig. S1E†); also, the color of ACB treated with distilled water was still white (Fig. S1C†). These results show that GO can be adsorbed onto the cuticle of ACB.
3.6 GO–insecticides destroy the cement layer of ACB cuticle
The cement layer plays an important role in protecting the wax layer.34 To understand the underlying synergistic mechanism of this strong insecticidal activity, SEM was used to study the detailed interactions of GO and GO–insecticides with the cement layer of ACB. The changes in the morphology of the ACB cement layer are shown in Fig. 5. Fig. 5A shows that the cement layer was arranged in a close and smooth manner under distilled water treatment. Although a wrinkled and raised cement layer was found under Cyf, Mon and Imi treatments, the structure of the cement layer remained intact and undamaged (Fig. 5B–D). However, under GO treatment, the structure of the cement layer was destroyed (Fig. 5E). The structural arrangement of the cement layer was disrupted and the integrity was damaged by GO–Cyf, GO–Mon and GO–Imi treatments (Fig. 5F–H). It should be noted that the exact timing of each image could not be accurately determined due to the limit of resolution and diversity among individual insects. Nevertheless, these rough but representative images help us understand the process of this GO-induced degradation of cement layer at the cellular level to some degree. This finding suggests that the structure of cement layer can be disrupted by both GO and GO–insecticide mixtures.
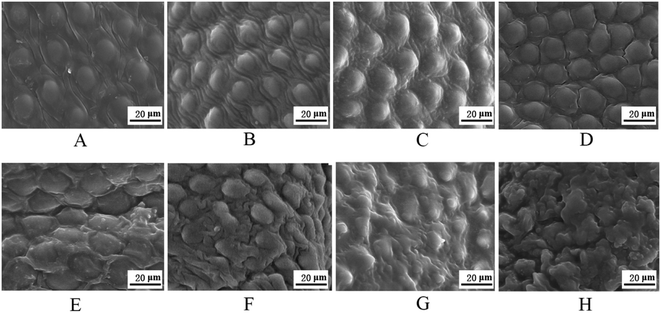 |
| Fig. 5 Representative SEM images of cement layer of ACB exposed to insecticides, GO–insecticide mixtures, and GO. Cement layers of ACB treated by distilled water and GO: (A) and (E); Cyf and Cyf–GO: (B) and (F); Mon and Mon–GO: (C) and (G); and Imi and Imi–GO: (D) and (H), respectively. | |
It is well-known that the cement layer, which is mainly composed of lipids, is the main barrier against the penetration of water and other aqueous solutions or suspensions.34 The lipids in the cement layer also play a key role in the restriction of transpiration and maintenance of water balance in the insect; some abrasive dusts were applied to the insecticides due to their desiccating effects that destroy the cement layer, which leads to water loss for the insects.38 Furthermore, many studies have demonstrated that there are strong physical interactions between lipid membranes of bacterial cells or other lipid membranes and GO nanosheets, which may cause membrane perturbations due to the piercing of the sharp edges of atomically thin GO nanosheets.39–41 It has been reported that GO can extract large amounts of phospholipids from the cell membranes of bacteria because of strong dispersion interactions between GO and lipid molecules, which may be a molecular basis for the cytotoxicity and antibacterial activity of GO.39 Similarly, in our experiment, the lipids in the cement layer of insects can also be disrupted by GO because GO first contacts the cement layer after treatment with GO–insecticide mixtures. Next, the strong interaction between GO and the lipids leads to the disruption of the cement layer. Finally, the disrupted cement layer cannot restrict the water loss caused by transpiration, resulting in a rapid water loss for the insects. Thus, ‘physical damage’ to the cement layer leads to enhanced water loss for the insects under treatments with GO–insecticides, which could be one reason for the synergistic effect of GO with insecticides. A similar insecticidal mechanism was observed in another report. Barik et al. reported that nano-silica is absorbed into the cuticular lipids of mustard beetle and thereby causes death purely by physical damage when applied on leaf and stem surface.42
Additionally, it was also shown that the penetration of insecticides occurs more rapidly through thinner and non-sclerotised regions of the cuticle.34 It has also been shown that the removal of the cuticle layer with solvents is correlated with enhanced insecticide penetration and insect mortality.43 Apparently, the disrupted cuticle is thinner than the normal cuticle and loses its protective activity to the insect body; thus, the penetration ability of insecticides into the insect can be increased by GO. In addition, the synergistic effect of GO with Imi also demonstrated that GO increases the penetration ability of pesticides against insect cuticle. Therefore, we speculate that the disrupted cement layer also provides a ‘green’ channel for the penetration of pesticides into the cuticle, which could be the second reason for the synergistic effect of GO with insecticides.
Many reports have shown that GO has efficient synergistic effects on anticancer drugs such as doxorubicin and chlorin e6 when used in the drug delivery system in medical science applications.44–46 In agriculture science, GO can be used as a copper and selenide carrier and remains as a reservoir in the leaf, thus significantly enhancing Pieris rapae larval mortality by the mechanism of reduced drift loss and targeted release.14 Polydopamine-coated GO can serve as an insecticide carrier to enhance the utilization efficiency and reduce wash-off, especially for water-soluble pesticides.15 Similarly, in our results, Fig. 2 indicates that Cyf, Mon, and Imi can also be adsorbed on the surface of GO. Fig. S1† shows that GO can be adsorbed on the cuticle of ACB. Therefore, we speculate that GO can serve as an insecticide carrier to enhance the efficacy of pesticides, which can be the third reason for the synergistic effect of GO with insecticides.
The wide and frequent use of insecticides in agriculture for pest management has led to the development and prevalence of resistant pest populations.47,48 It has been well established that synergists applied in insecticides can increase the lethality of insecticides by inhibiting insecticide detoxifying enzymes.49 However, our results show that the synergistic action mode of GO results in ‘physical damage’ to the cement layer of insects, which disrupts the cuticle integrity and provides a new channel for insecticide penetration. This distinctive action mode of GO may contribute to the reduction of insecticide resistance of pests. Moreover, it is well-known that the outmost layer of insects is generally composed of cement layers.38 Resistant and non-resistant pests have the same cuticle structure, and the difference between them lies in the molecular resistant mechanism towards the insecticides. Therefore, we can assume that GO also has great synergistic effects on other pesticides for pest management, suggesting potential applications of in vivo synergistic activity to counteract the limitations of existing insecticides caused by pest resistance.
4. Conclusions
In this study, GO was demonstrated to have multifunctional synergistic activity towards insecticides against lepidoptera insects. The synergistic mechanism of GO can be summarized as follows: the disruption of cement layer of insects results in a rapid water loss of the insects and provides a new channel for insecticides to penetrate into the cuticle; besides, the GO delivery system can enhance the efficacy of the pesticides. Combination of the multifunctional synergistic mechanism of GO and the toxicity of insecticides may contribute to stronger insecticidal effects against both resistant and non-resistant pests. Thus, our current findings might provide implications for the design of novel insecticides and other pest management applications. In particular, we speculate that GO has the potential to become a new type of ‘green’ synergist for pest management, which will counteract the insect resistance due to its multifunctional synergistic mechanism. This study might also stimulate and facilitate toxicity studies for other nanomaterials in the emerging field of nano-pesticides. Future studies can include other important agricultural insects into the bioassay to expand the spectrum of insecticidal activities of the GO–insecticides. The fundamental research on the synergistic activity of GO will lay the groundwork for extending the potential application of GO in insecticidal materials for plant protection. At the same time, there are still several key challenges such as the possible unknown toxic effects of GO on non-target organisms and the subsequent environmental risks in the application of GO for plant protection. Addressing these challenges may help ensure safe and sustainable design, development, and implementation of nano-pesticides.
Conflicts of interest
The authors declare no competing financial interest.
Acknowledgements
The authors gratefully acknowledge the support for this research from the National Key Research and Development Program of China (2017YFD0201802), China Agriculture Research System (CARS-02), and National Natural Science Foundation of China (31501680).
Notes and references
- L. Sun, T. Du, C. Hu, J. Chen and J. Lu, Antibacterial activity of graphene oxide/g-C3N4 composite through photocatalytic disinfection under visible Light, ACS Sustainable Chem. Eng., 2017, 5, 8693–8701 CrossRef CAS.
- K. A. Abd-Elsalam, Nanoplatforms for plant pathogenic fungi management, Fungal Genomics Biol., 2012, 2, 2 CrossRef.
- J. S. Duhan, V. Kumar, N. Kumar, P. Kaur, K. Nehra and S. Duhan, The new perspective in precision agriculture, Biotechnol. Rep., 2017, 15, 11–23 CrossRef PubMed.
- M. Kah, S. Beulke, K. Tiede and T. Hofmann, Nanopesticides: state of knowledge, environmental fate, and exposure modeling, Crit. Rev. Environ. Sci. Technol., 2013, 16, 1823–1867 CrossRef.
- A. Gogos, K. Knauer and T. D. Bucheli, Nanomaterials in plant protection and fertilization: current state, foreseen applications, and research priorities, J. Agric. Food Chem., 2012, 60, 9781–9792 CrossRef CAS PubMed.
- S. R. Choudhurya, K. K. Nair, R. Kumar, R. Gogoi, C. Srivastava, M. Gopal, B. S. Subhramanyamd, C. Devakumar and A. Goswami, Nanosulfur: a potent fungicide against food pathogen, Aspergillus niger, AIP Conf. Proc., 2010, 1276, 154–157 CrossRef.
-
F. Torney, Emerging technologies in plant science research. Interdepartmental Plant Physiology Major Fall Seminar Series Phys., 2009, p. 696 Search PubMed.
- N. Kumar, R. Kumar, N. A. Shakil and T. K. Das, Nanoformulations of pretilachlor herbicide: preparation, characterization and activity, J. Sci. Ind. Res., 2016, 75, 676–680 CAS.
- M. R. Shurin, N. Yanamala, E. R. Kisin, A. V. Tkach, G. V. Shurin, A. R. Murray, H. D. Leonard, J. S. Reynolds, D. W. Gutkin, A. Star, B. Fadeel, K. Savolainen, V. E. Kagan and A. A. Shvedova, Graphene oxide attenuates Th2-Type immune responses, but augments airway remodeling and hyperresponsiveness in a murine model of asthma, ACS Nano, 2014, 8, 5585–5599 CrossRef CAS PubMed.
- M. Bramini, S. Sacchetti, A. Armirotti, A. Rocchi, E. Vázquez, V. L. Castellanos, T. Bandiera, F. Cesca and F. Benfenati, Graphene oxide nanosheets disrupt Lipid composition, Ca2+ homeostasis, and synaptic transmission in primary cortical neurons, ACS Nano, 2016, 10, 7154–7171 CrossRef CAS PubMed.
- S. Kim, K. C. Kwon, J. Y. Park, H. W. Cho, I. Lee, S. Y. Kim and J. L. Lee, Challenge beyond graphene: metal oxide/graphene/metal oxide electrodes for optoelectronic devices, ACS Appl. Mater. Interfaces, 2016, 8, 12932–12939 CrossRef CAS PubMed.
- V. Georgakilas, J. N. Tiwari, K. C. Kemp, J. A. Perman, A. B. Bourlinos and K. S. Kim, Noncovalent functionalization of graphene and graphene oxide for energy materials, biosensing, catalytic, and biomedical applications, Chem. Rev., 2016, 116, 5464–5519 CrossRef CAS PubMed.
- M. Soikkeli, K. Kurppa, M. Kainlauri, S. Arpiainen, A. Paananen, D. Gunnarsson, J. J. Joensuu, P. Laaksonen, M. Prunnila, M. B. Linder and J. Ahopelto, Graphene biosensor programming with genetically engineered fusion protein monolayers, ACS Appl. Mater. Interfaces, 2016, 8, 8257–8264 CrossRef CAS PubMed.
- S. Sharma, S. Singh, A. K. Ganguli and V. Shanmugam, Anti-drift nano-stickers made of graphene oxide for targeted pesticide delivery and crop pest control, Carbon, 2017, 115, 781–790 CrossRef CAS.
- Y. J. Tong, L. H. Shao, X. L. Li, J. Q. Lu, H. L. Sun, S. Xiang, Z. H. Zhang, Y. Wu and X. M. Wu, Adhesive and stimuli-responsive
polydopamine-coated graphene oxide system for pesticide loss control, J. Agric. Food Chem., 2018, 66, 2616–2622 CrossRef CAS PubMed.
- H. A. Khan, W. Akram and S. A. Shad, Genetics, cross-resistance and mechanism of resistance to spinosad in a field strain of Musca domestica L. (Diptera: Muscidae), Acta. Trop., 2014, 130, 148–154 CrossRef CAS PubMed.
- G. S. Chahil, K. Mandal, S. K. Sahoo, R. S. Battu and B. Singh, Risk assessment of β-cyfluthrin and imidacloprid in chickpea pods and leaves, Ecotoxicol. Environ. Saf., 2014, 101, 177–183 CrossRef CAS PubMed.
- D. M. Kristensen, J. Huang, C. L. Qiao and J. B. Jespersen, Variation of Musca domestica L. acetylcholinesterase in Danish housefly populations, Pest Manage. Sci., 2006, 62, 738–745 CrossRef PubMed.
- K. S. Chang, H. C. Kim, T. A. Klein and Y. R. Ju, Insecticide resistance and cytochrome-P450 activation in unfed and blood-fed laboratory and field populations of Culex pipiens pallens, J. Pestic. Sci., 2017, 90, 1–13 Search PubMed.
- J. W. S. Hummers and R. E. Offeman, Preparation of graphitic oxide, J. Am. Chem. Soc., 1958, 80, 1339 CrossRef.
-
D. R. Zhou, K. L. He, Z. Y. Wang, Z. H. Ye, L. P. Wen, Y. X. Gao and Y. Y. Song, Asian corn borer and its integrated management, Golden Shield Press, Beijing, 1995 Search PubMed.
- J. Guillén and P. Bielza, Thiamethoxamacts as a target-site synergist of spinosad in resistant strains of Frankliniella occidentalis, Pest Manage. Sci., 2013, 69, 188–194 CrossRef PubMed.
- W. S. Abbott, A method of computing the effectiveness of an insecticide, J. Econ. Entomol., 1925, 18, 265–267 CrossRef CAS.
- M. Ahmad and R. M. Hollingworth, Synergism of insecticides provides evidence of metabolic mechanisms of resistance in the obliquebanded leafroller Choristoneura rosaceana (Lepidoptera: Tortricidae), Pest Manage. Sci., 2004, 60, 465–473 CrossRef CAS PubMed.
- L. Y. Zhang, X. R. Li, M. R. Wang, Y. J. He, L. Y. Chai, J. Y. Huang, H. Y. Wang, X. W. Wu and Y. K. Lai, Highly flexible and porous nanoparticle-loaded films for dye removal by graphene oxide−fungus interaction, ACS Appl. Mater. Interfaces, 2016, 8, 34638–34647 CrossRef CAS PubMed.
- C. H. Deng, J. L. Gong, G. M. Zeng, C. G. Niu, Q. Y. Niu, W. Zhang and H. Y. Liu, Inactivation performance and mechanism of Escherichia coli in aqueous system exposed to iron oxide loaded graphene nanocomposites, J. Hazard. Mater., 2014, 276, 66–76 CrossRef CAS PubMed.
- C. Lin, B. Fugetsu, Y. B. Su and F. Watari, Studies on toxicity of multi walled carbon nanotubes on Arabidopsis T87 suspension cells, J. Hazard. Mater., 2009, 170, 578–583 CrossRef CAS PubMed.
- S. M. Maliyekkal, T. S. Sreeprasad, D. Krishnan, S. Kouser, A. K. Mishra, U. V. Waghmare and T. Pradeep, Graphene: a reusable substrate for unprecedented adsorption of pesticides, Small, 2013, 9, 273–283 CrossRef CAS PubMed.
- F. L. Fernandes, L. Bacci and M. S. Fernandes, Impact and selectivity of insecticides to predators and parasitoids, EntomoBrasilis, 2010, 3, 1–10 CrossRef.
- A. Grzybowski, M. Tiboni, M. A. Silva, R. F. Chitolina, M. Passos and J. D. Fontana, Synergistic larvicidal effect and morphological alterations induced by ethanolic extracts of Annona muricata and Piper nigrum against the dengue fever vector Aedes aegypti, Pest Manage. Sci., 2013, 69, 589–601 CrossRef CAS PubMed.
- K. H. Lockey, Lipids of the insect cuticle: origin, composition and function, Comp. Biochem. Phys. B, 1988, 89, 595–645 CrossRef.
- M. P. Broderick, L. D. Leake, M. G. Ford, R. Greenwood and R. Crichton, Simultaneous recording of the neurophysiological effects and toxicological symptoms of cypermethrin poisoning in intact larvae of Spodoptera littoralis boisd, Pestic. Sci., 2010, 32, 57–72 CrossRef.
-
Z. Q. Ma, Doctorial thesis, Studies on the relationship between the symptoms
and mechanism of different kinds of insecticides, Northwest A & F University, Xian, China, 2012 Search PubMed.
- J. Noble-Nesbitt, Structural aspects of penetration through insect cuticles, Pestic. Sci., 1970, 1, 204–208 CrossRef CAS.
- B. A. Kenneth Mellanby, The evaporation of water from insects, Biol. Rev., 2008, 10, 317–333 CrossRef.
- B. D. Rohitha Prasantha, Ch. Reichmuth, C. Adler and D. Felgentreu, Lipid adsorption of diatomaceous earths and increased water permeability in the epicuticle layer of the cowpea weevil Callosobruchus maculatus (F.) and the bean weevil Acanthoscelides obtectus (Say) (Chrysomelidae), J. Stored Prod. Res., 2015, 64, 36–41 CrossRef.
-
W. Z. Cai, X. F. Pang, B. Z. Hua, G. W. Liang and D. L. Song, General Entomology, China Agricultural University Press, Beijing, 2011, p. 105 Search PubMed.
- R. A. Johnson, A. Kaiser, M. Quinlan and W. Sharp, Effect of cuticular abrasion and recovery on water loss rates in queens of the desert harvester ant Messor pergandei, J. Exp. Biol., 2011, 214, 3495–3506 CrossRef PubMed.
- Y. S. Tu, M. Lv, X. Peng, T. Huynh, M. Zhang, M. Castelli, Z. R. Liu, Q. Huang, C. H. Fan, H. P. Fang and R. H. Zhou, Destructive extraction of phospholipids from Escherichia coli membranes by graphene nanosheets, Nat. Nanotechnol., 2013, 8, 594–601 CrossRef CAS PubMed.
- R. Frost, S. Svedhem, C. Langhammer and B. Kasemo, Graphene oxide and lipid membranes: size-dependent interactions, Langmuir, 2016, 32, 2708–2717 CrossRef CAS PubMed.
- R. Frost, G. E. Jönsson, D. Chakarov, S. Svedhem and B. Kasemo, Graphene oxide and lipid membranes: Interactions and nanocomposite structures, Nano Lett., 2012, 12, 3356–3362 CrossRef CAS PubMed.
- T. K. Barik, B. Sahu and V. Swain, Nano-silica-from medicine to pest control, Parasitol. Res., 2008, 103, 253–258 CrossRef CAS PubMed.
- M. P. Juárez, Inhibition of cuticular lipid synthesis and its effect on insect survival, Arch. Insect Biochem. Physiol., 1994, 25, 177–191 CrossRef PubMed.
- G. Liu, H. Shen, J. Mao, L. Zhang, Z. Jiang, T. Sun, Q. Lan and Z. Zhang, Transferrin modified graphene oxide for glioma-targeted drug delivery: In vitro and in vivo evaluations, ACS Appl. Mater. Interfaces, 2013, 5, 6909–6914 CrossRef CAS PubMed.
- Z. Ma, Y. F. Qiu, H. H. Yang, Y. M. Huang, J. J. Liu, Y. Lu, C. Zhang and P. Hu, Effective synergistic effect of dipeptide-polyoxometalate-graphene oxide ternary hybrid materials on peroxidase-like mimics with enhanced performance, ACS Appl. Mater. Interfaces, 2015, 7, 22036–22045 CrossRef CAS PubMed.
- C. Wu, Q. He, A. Zhu, D. Li, M. Xu, H. Yang and Y. Y. Liu, Synergistic anticancer activity of photo- and chemoresponsive nanoformulation based on polylysine-functionalized graphene, ACS Appl. Mater. Interfaces, 2014, 6, 21615–21623 CrossRef CAS PubMed.
- J. C. Hsu, H. T. Feng and W. J. Wu, Resistance and synergistic effects of insecticides in Bactrocera dorsalis (Diptera: Tephritidae) in Taiwan, J. Econ. Entomol., 2004, 97, 1682–1688 CrossRef CAS PubMed.
- M. B. S. Afzal and S. A. Shad, Resistance inheritance and mechanism to emamectin benzoate in Phenacoccus solenopsis (Homoptera: Pseudococcidae), Crop Prot., 2015, 71, 60–65 CrossRef.
- J. J. Wang, D. Wei, W. Dou, F. Hu and W. F. Liu, Toxicities and synergistic effects of Several insecticides against the oriental fruit fly (Diptera: Tephritidae), J. Econ. Entomol., 2013, 106, 970–978 CrossRef CAS PubMed.
Footnotes |
† Electronic supplementary information (ESI) available. See DOI: 10.1039/c8en00902c |
‡ Xiuping Wang and Haicui Xie contributed equally to this work. |
|
This journal is © The Royal Society of Chemistry 2019 |