NO2 and natural organic matter affect both soot aggregation behavior and sorption of S-metolachlor†
Received
24th July 2019
, Accepted 23rd August 2019
First published on 26th August 2019
Abstract
Soot is an important carbonaceous nanoparticle (CNP) frequently found in natural environments. Its entry into surface waters can occur directly via surface runoff or infiltration, as well as via atmospheric deposition. Pristine soot is likely to rapidly undergo aggregation and subsequent sedimentation in aquatic environments. Further, soot can sorb a variety of organic contaminants, such as S-metolachlor (log
KD = 3.25 ± 0.12). During atmospheric transport, soot can be chemically transformed by reactive oxygen species including NO2. The presence of natural organic matter (NOM) in surface waters can further affect the aquatic fate of soot. To better understand the processes driving the fate of soot and its interactions with contaminants, pristine and NO2-transformed model soot suspensions were investigated in the presence and absence of NOM. NO2-oxidized soot showed a smaller particle size, a higher number of particles remaining in suspension, and a decreased sorption of S-metolachlor (log
KD = 2.47 ± 0.40). In agreement with findings for other CNPs, soot stability against aggregation was increased for both pristine and NO2 transformed soot in the presence of NOM.
Environmental significance
Soot is an important carbonaceous nanoparticle (CNP) frequently found in natural environments with abundances up to 104 times higher compared to widely studied engineered CNPs. Although soot CNPs are highly relevant to surface water systems, to date, the nano-particulate properties of soot in the natural environment have received only a little attention. Here we investigate the effects of atmospheric transformation with NO2 on soot aggregation and contaminant sorption using the widely found herbicide S-metolachlor as a model contaminant. The findings of this study will help to better understand the colloidal behavior of pristine soot compared to atmospherically transformed soot and bring forward hitherto underexplored aspects of soot as a naturally occurring CNP.
|
1. Introduction
Due to climate change and shifting land usage, wildfires have increased in abundance and intensity in recent years and are expected to further increase in the future.1 Wildfires and incomplete combustion of biomass and fossil fuel can form a considerable amount of carbon nanoparticles (CNPs). One type of CNP is soot, the finest fraction of incomplete combustion residues formed via re-condensation and nucleation of organic compounds from the gas phase during the combustion process.2 Annual production of soot transportable via the atmosphere is estimated to be 17 Tg per year.3 Thus, soot emissions are expected to be at least 104 times more abundant compared to widely studied engineered CNPs including graphene oxides, fullerenes and carbon nanotubes.4
Once released into the atmosphere, soot can undergo chemical transformation, resulting in changes in the surface chemistry. Key processes for atmospheric soot transformation are photochemical redox reactions with NOx, SOx, O3 and other reactive oxygen species in the atmosphere.5,6 Over time NO is predominantly oxidized to NO2 in the atmosphere, thereby making NO2 the most abundant NOx reactive oxygen species in the atmosphere. The reaction of NO2 with soot was previously elucidated using spectroscopic techniques where infrared bands were attributed to acidic functional groups (C(
O)O–R, R–NO2, and R–ONO are the main functionalities).7,8
Soot – pristine or atmospherically transformed – can reach water bodies directly via atmospheric deposition and indirectly via surface runoff and infiltration. In suspension, soot can remain colloidally stable, i.e. dispersed, and be transported in the aqueous phase, or it can aggregate and deposit. The aggregation behavior of soot is expected to depend mainly on the soot surface charge, the presence of natural organic matter (NOM), and the ionic composition of the aqueous environment.4 A recent study by Chen et al.9 found that soot aggregation followed classic Derjaguin–Landau–Verwey–Overbeek (DLVO) theory,10–12 describing the aggregation behavior of particles as a combination of van der Waals attraction and electrostatic repulsion. Depending on the aqueous chemistry, soot particles can remain stable against homo-aggregation in typical freshwater environments but are likely to aggregate under saline conditions (e.g. estuaries) due to the compression of the electrical double layer at higher ionic strengths. The effect of chemical transformation (e.g. with NO2) on the aggregate size of soot particles has to date received little attention. But with an increasing negative surface charge and the presence of NOM soot particles are expected to form increasingly stable suspensions.4
In the water phase, soot has a strong adsorption affinity for a range of organic contaminants,13–16 and because of its higher abundance compared to other CNPs, soot may significantly alter the fate of organic contaminants occurring in surface waters. From other CNPs it is known that the presence of NOM strongly affects particle aggregation and size and may also influence the sorption of organic contaminants due to changes in accessibility of sorption sites. For instance, a number of studies have shown that the presence of NOM can decrease the sorption affinity of carbon nanotubes for organic contaminants by competing for sorption sites and blocking the accessibility to micropores.17–19 In contrast, both Wang et al.20 and Hou et al.21 found that NOM can increase the sorption affinity of organic contaminants to fullerene (nC60) aggregates. This can be explained by an increase in accessible sorption sites due to decreased aggregation of nC60. However, it remains unclear if similar effects occur with soot particle suspensions.
Pesticides are of environmental interest due to their widespread use and subsequent occurrence in natural waters. The herbicide S-metolachlor was selected as a model contaminant because of its (i) structural similarities to several other mobile organic contaminants, i.e. an aromatic ring substituted with polar functional groups, (ii) widespread use since 1978 in the USA and most European countries in corn and cotton production, and (iii) ecotoxicity in several aquatic species and birds.22,23
Sorption of organic compounds to “soot in water” is generally weaker than that to “soot in air”, indicating that surface coverage with water suppresses the sorption by soot.24 Thus, soot is expected to interact most strongly with organic contaminants when it is not fully wetted. Investigations into the pre-wetting kinetics of 1,2,4-trichlorobenzene and phenanthrene sorption by Nguyen et al.14 showed that the complete wetting of soot requires more than 20 days and the corresponding degree of water coverage of soot affects sorption of organic contaminants. In addition, most soot particles are expected to be in suspension directly after their introduction into water bodies, before they can sediment. Therefore, with the intent to simulate environmentally meaningful scenarios, this study tackled the behavior of soot freshly introduced into water.
With the aim to obtain a better understanding of soot aggregation and interactions with organic contaminants, pristine and NO2-transformed model soot was investigated in the presence and absence of NOM. We hypothesize that (i) NO2-transformation and interactions with NOM increase the aggregation stability of soot and (ii) sorption of the model contaminant S-metolachlor is affected by changes in soot surface chemistry and interactions with NOM.
2. Materials and methods
Analytical grade S-metolachlor, methanol, acetonitrile, HNO3, and CaCl2 were purchased from Sigma-Aldrich (Germany). Suwannee River NOM was purchased from the International Humic Substances Society (USA). Milli-Q water was obtained using a Millipore instrument (Elix 5 Milli-Q Gradient A10, USA).
2.1 Soot preparation
Printex U soot was purchased from Evonik Carbon Black GmbH (Germany). Printex U is industrial soot with similarities to diesel engine soot which has previously been well characterized and used as a model soot sample.26,27 To approximate transformation by NO2, the soot was treated according to a protocol adapted from Wei et al.28 In short, a thin layer of soot (approximately 2 mm) was exposed to saturated HNO3 vapor containing NO2 within a sealed glass desiccator under vacuum for 24 h at room temperature (25 °C) at a pressure of 200 mbar. The experimental setup did not allow for the measurement of NO2 and other radical species that could also have formed (i.e. OH˙). However, the degree of transformation was likely above the short term soot transformation expected in the atmosphere. Both pristine and NO2-transformed soot were thereafter individually rinsed with Milli-Q water (250 mL g−1, Fisher Scientific Phase Separating Filter Paper, Germany). Dry powdered soot samples were weighed into the respective background solution and vortexed for 30 s prior to any experiment.
2.2 Soot characterization
Sorbent suspensions of pristine and transformed soot were prepared in 1 mM CaCl2 with or without NOM (10 mg L−1 DOC) and shaken for 24 h to obtain similar conditions to those in the sorption batch experiment (see Section 2.3). For selected samples DOC concentrations were measured after 24 h of shaking to determine the DOC loading on soot in the experiments (centrifugation at 1000g for 30 min and filtration, <0.22 μm, Shimadzu L series TOC). The hydrodynamic diameter of the particles was calculated by dynamic light scattering (DLS) with a ZetaSizer Nano ZS (Malvern Instruments Ltd., UK) with a laser of 632.8 nm wavelength at a fixed angle of 173°. Soot suspensions were vortexed for 10 s after the soot was transferred into the respective background solution. Including sample transfer and automatic adjustment of measurement settings by the instrument, the first measurement point was obtained after 80 s. Data for individual measurements of 10 s each for 30 min and for 1 min after 24 h of shaking were collected automatically. The autocorrelation function was fitted using the cumulant method, assuming a refractive index of 2.4. The polydispersity index (PdI) ranged from 0.2 to 0.8. Thereafter, the electrophoretic mobility of the particles remaining in suspension after a settling period of 1 min was measured and converted to the ζ potential using 3 measurements with 20 runs each applying Henry's equation. The electrical conductivity was above 200 μS cm−1. All samples were run in duplicate. The ash content was determined by weighing samples before and after heating at 750 °C for 6 h. The total C, H, and N contents were determined using an elemental analyzer (Elementar Vario Macro, Germany), and the O content was calculated by mass balance: O% = 100 − (C + H + N + ash). The BET specific surface area and pore size distribution were determined using N2 physisorption following protocols previously described in Sigmund et al.29 For scanning electron microscopy (Zeiss Sigma VP 300, acceleration voltage of 5 kV, working distance 3.6 mm, magnification 1.56k), single drops (20 μL) of the four soot suspensions after shaking for 24 h and settling for 1 min were placed on aluminum foil and dried in a desiccator. Fourier transform infrared spectra of dried samples (FTIR Bruker Tensor 27) were measured with a resolution of 4 cm−1 and 128 scans.
2.3 Sorption experiments
Sorption experiments were conducted at room temperature using the batch method based on OECD guideline 106 at 1 mM CaCl2 and at eight different S-metolachlor concentrations in the range of 1 to 50 mg L−1 (remaining at least one order of magnitude below solubility).30 Experiments for sorption of S-metolachlor and soot aggregation were carried out in suspensions comprising 10 mL aqueous phase loaded with 10 mg soot (i.e. 1 g soot per L), which is likely above most concentrations that may occur in the natural environment. The pH ranged between 6.8 and 7.4 throughout all experiments and did not significantly differ between pristine and NO2-transformed soot. S-Metolachlor is a polar but non-ionizable compound and pH is thus not expected to strongly affect sorption even though functional groups on the soot surface may have been partially dissociated. The samples were spiked with S-metolachlor using a methanolic stock solution (volume < 1% to avoid co-solvent effects) and equilibrated on a shaker at 125 rpm for 24 h to reach equilibrium (pre-experiments showed that equilibrium was reached after 16 h; see Fig. S1 in the ESI†). Thereafter, a small volume of the suspension was filtered (<0.20 μm) and analyzed by HPLC (Phenomenex Luna C18 50 × 2.00 mm 5 μm column, detection at 210 nm with a Shimadzu DAD SPD-M20A detector). For the mobile phase a solvent mixture of Milli-Q water and acetonitrile was used (20/80 by volume). The limit of quantification for S-metolachlor was calculated to be tenfold the background noise and was 0.10 mg L−1. Measured concentrations ranged between 0.4 and 40 mg L−1 and recoveries were calculated based on control samples without soot and used for concentration correction (recoveries ranged from 90 to 115% over the concentration range investigated). Sorbent loading was calculated via mass balance and fractions of S-metolachlor sorbed ranged from 6.5 to 85%.
2.4 Data analysis
Sorption isotherms were fitted with the Freundlich model:where q (mg kg−1) is the sorbent loading, Caq (mg L−1) is the aqueous concentration of the sorbate, KF is the Freundlich coefficient, and n is the Freundlich exponent representing isotherm non-linearity (non-linearity increases as n deviates from unity). For a comparison among the soot suspensions and a prediction model, individual sorption coefficients (KD, L kg−1) were calculated with the Freundlich equation at a fixed aqueous concentration of 10 mg L−1. Standard deviations (σ) of the Freundlich fit based KD values at a given aqueous concentration were calculated using the uncertainty propagation equation.2 |  | (2) |
Standard deviations for ppLFER-based KD values at a given aqueous concentration were calculated using linear uncertainty propagation.
3. Results and discussion
3.1 Particle size decreased after transformation with NO2 and interaction with NOM
The measured ζ-potential increased upon transformation from −8.5 ± 0.8 mV for pristine soot to −19.4 ± 0.4 mV for transformed soot (see Fig. 1), indicating the formation of negatively charged functional groups on the NO2-transformed soot surface (e.g. R–C
O and/or R–COOH as indicated in FTIR spectra; see Fig. S2 in the ESI†). The formation of nitrogen- and oxygen-containing surface functional groups (C(
O)O–R, R–NO2, and R–ONO) has previously been reported in the literature7,8 and is additionally confirmed by an increase in the nitrogen and oxygen content (Table 2).
 |
| Fig. 1 (A) Particle size and ζ-potential of pristine soot decreased after NO2-transformation and/or interaction with NOM. (B) Particle count increased with NO2 transformation and in the presence of NOM. | |
Table 1 Selected properties of S-metolachlor. KOW is the octanol water partition constant, V is the McGowan volume [m3/100 mol], E is the excess molar refractivity, and A and B are the hydrogen bond acidity and basicity, respectively. The Abraham solute parameters V, E, A and B were obtained from the UFZ-LSER database25
Table 2 Elemental composition of soot particles upon transformation with NO2
|
C [%] |
H [%] |
N [%] |
O [%] |
Ash [%] |
Pristine |
93.76 ± 0.11 |
0.04 ± 0.09 |
0.47 ± 0.08 |
0.69 ± 0.56 |
5.12 ± 0.31 |
NO2 |
84.91 ± 0.11 |
0.07 ± 0.09 |
1.19 ± 0.08 |
9.36 ± 1.26 |
2.74 ± 1.01 |
The increase in negative surface charge induced a decrease in size of the suspended soot particles based on DLS measurements (Fig. 1A). The measurements presented were obtained after 24 h of shaking to be comparable with sorption batch experiments. In addition, aggregation kinetics for the first 30 min after introduction of the soot into the water phase were determined (Fig. S3†).
The exposure of soot to NOM did not significantly change the elemental composition of the material (p > 0.05). This is consistent with the measured DOC loading of soot (17.7 ± 0.3 mg DOC per g soot). However, it increased the negative ζ-potential of soot to a similar degree as the NO2 transformation, stabilizing the soot particles electrostatically against aggregation and decreasing their particle size (Fig. 1A). In contrast to the effect on pristine soot, NOM could not increase the negative surface charge of NO2-transformed soot.
The number of particles remaining in suspension increased both after NO2 transformation and after interaction with NOM (Fig. 1B), with the particle number concentration increasing up to 30-fold compared to pristine soot. The aggregate size decreased and the number of particles remaining in suspension was higher for NO2-transformed soot with NOM compared to NO2-transformed soot without NOM. This indicates that in addition to electrostatic repulsion, steric effects might also play a role in the aggregation of soot in the presence of NOM.4
In good agreement with DLS measurements and particle mass remaining in suspension, scanning electron microscopy images confirmed the higher number and smaller size of suspended particles for NO2 transformed soot compared to pristine soot. The stabilizing effect of NOM on soot aggregation could also be observed when comparing suspensions with and without NOM (Fig. 2), where differences in particle aggregation were more pronounced for pristine soot than for NO2-transformed soot. It should be noted that relatively high soot concentrations were used in this study, and in many scenarios hetero-aggregation with other suspended particles in the natural environment is expected to outweigh the importance of the homo-aggregation investigated here.4,31,32 However, the investigation of hetero-aggregation was not in the scope of this study and may be an interesting subject of further investigations building on the results presented here.
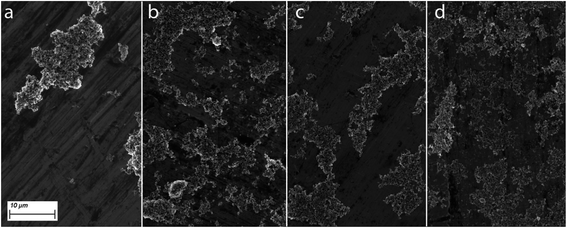 |
| Fig. 2 Scanning electron microscopy images of soot particles with different surface chemistry and aggregate sizes for (a) pristine soot, (b) pristine soot with NOM, (c) NO2-transformed soot, and (d) NO2-transformed soot with NOM (same magnification of 1.56k for all). | |
3.2 Sorption of S-metolachlor decreased upon NO2-transformation and was not affected by the presence of NOM
Sorption (KD) of S-metolachlor decreased by more than one order of magnitude after NO2-transformation of soot and was not significantly affected (p > 0.05) by the presence of NOM (see Fig. 3 and Table 3). The decrease in sorption of S-metolachlor upon NO2-transformation can be explained by a lower hydrophobicity of the soot surface and a less favorable accessibility of surface sorption sites.4,33 Therefore, the higher number of O-containing surface functional groups on the NO2-transformed soot is energetically favorable for H-bond interactions with water molecules, which increases the competitiveness of water molecules for sorption sites.34,35 A decrease of organic contaminant sorption with an increase of O-functional groups is in good agreement with the literature findings on other carbonaceous materials, including carbon nanotubes,36,37 fullerenes,33,37 and biochars.38,39
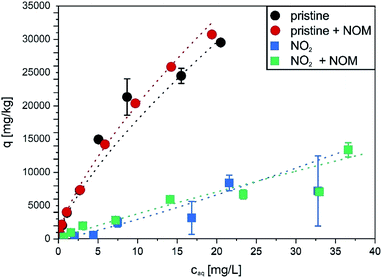 |
| Fig. 3 Sorption of S-metolachlor to NO2-transformed soot was weaker than that to pristine soot. Filled symbols (●, ■) are sorption data, dotted lines are Freundlich sorption isotherm fits. | |
Table 3 Freundlich fitting parameters (KF and n) for S-metolachlor with sorption to NO2-transformed soot being more linear and weaker compared to that to pristine soot
|
K
F [mg kg−1 (mg L)−n] |
n [—] |
R
2
|
Pristine |
3382 ± 52 |
0.72 ± 0.01 |
0.991 |
Pristine + NOM |
3898 ± 70 |
0.71 ± 0.02 |
0.991 |
NO2 |
475 ± 46 |
0.90 ± 0.05 |
0.956 |
NO2 + NOM |
198 ± 22 |
1.17 ± 0.04 |
0.994 |
The change in particle size was not reflected in the change in sorption of S-metolachlor. For instance, the particle size decreased for pristine soot after interaction with NOM, but did not significantly change the sorption. The BET specific surface area was 84.7 ± 3.1 and 89.9 ± 4.0 m2 g−1 for pristine and NO2 treated soot, respectively. Thus, the total specific surface area, which did not differ significantly between the materials, was not a limiting factor for S-metolachlor sorption under the conditions investigated. Also, under the conditions investigated, the presence of NOM did not suppress sorption due to competition for sorption sites, possibly because the high specific surface area offered enough sorption sites for both sorbates. This is consistent with previous observations on pyrene sorption to carbon nanotubes, which was only substantially suppressed by NOM at concentrations > 20 mg L−1.40 It should be noted that both the salt (1 mM CaCl2) and the NOM concentration (10 mg L−1 DOC) used in this study correspond to concentrations that can be found in natural aquatic environments,41 and a higher concentration of NOM (e.g. 100 mg L−1) may have been able to suppress sorption to soot surfaces via competition for sorption sites.40
A recently published sorbate-activity-dependent poly-parameter linear free-energy relationship (ppLFER) for pristine diesel soot15 found that the difference in dispersive interactions with the soot versus with the water was the dominant factor encouraging adsorption, and H-bonding interactions discouraged this process. We applied the ppLFER proposed by Lu et al.15 using Abraham solute parameters for S-metolachlor from the UFZ-LSER database25 as presented in Table 1 (calculation in Table S1†). The ppLFER predicted that KD value ranges were in good agreement with the values determined in this study but could not predict the differences observed between pristine soot and NO2 transformed soot (see Table 4). The discrepancy of KD values between the ppLFER and this study may partially be explained by differences in the surface chemistry (e.g. oxygen containing surface functional groups); however, such data were not reported in the study by Lu et al.15
Table 4 The sorption coefficient (KD) range calculated based on the ppLFER proposed by Lu et al.15 is in the same range as the coefficients derived in this study, but the ppLFER was not able to differentiate between pristine and NO2-transformed soot. All parameters were calculated at an aqueous concentration of 10 mg L−1
|
log KD from ppLFER |
log KD from Freundlich fit |
Pristine |
2.86 ± 0.49 |
3.25 ± 0.12 |
Pristine + NOM |
3.31 ± 0.23 |
NO2 |
2.81 ± 0.49 |
2.47 ± 0.40 |
NO2 + NOM |
2.58 ± 0.40 |
4. Conclusion
Soot is an important naturally occurring CNP that can be found ubiquitously in the environment. During atmospheric transport, soot can be chemically transformed by reactive oxygen species, including NO2. Our results show that reaction of soot with NO2 has significant impacts on aggregation and sorption of soot in aquatic environments. Pristine soot is a strong sorbent for S-metolachlor, but is likely to rapidly aggregate when introduced directly into surface waters. In contrast, soot that is oxidized by NO2 shows a decreased sorption for S-metolachlor, but exhibits a higher stability against aggregation. In agreement with findings on other CNPs, soot aggregation decreased in the presence of NOM. NO2-transformed soot can act as a contaminant vector; however, due to the significantly lower sorption potential for S-metolachlor, the expected risk is lower than that for pristine soot or soot stabilized by NOM.
Future research on soot CNP properties should focus on additional transformations and changes in soot properties that can occur in the natural environment. For instance, hetero-aggregation of soot and NO2-transformed soot with mineral particles remains an underexplored aspect and should be the subject of further investigations.
Conflicts of interest
There are no conflicts to declare.
Acknowledgements
The authors would like to thank Christine Sternkopf (Technical University of Munich) for her help with electron microscopy, as well as Christoph Haisch (Technical University of Munich) and Mehdi Gharasoo (Institute of Groundwater Ecology, Helmholtz Center, Munich) for fruitful discussions. This study was partly funded by the Austrian Science Fund (FWF) [project number P27689-N28] and by the European Research Council (ERC) under the European Union's Seventh Framework Programme (FP7-IDEAS-ERC) [ERC Grant Agreement No. 616861 (MicroDegrade)].
References
-
O. Hoegh-Guldberg, D. Jacob and M. Taylor, et al., Impacts of 1.5 °C global warming on natural and human systems, Glob Warm 15 °C - IPCC's Spec Assess Rep., 2018 Search PubMed.
- H. Wang, Formation of nascent soot and other condensed-phase materials in flames, Proc. Combust. Inst., 2011, 33(1), 41–67 CrossRef CAS.
- M. I. Bird, J. G. Wynn, G. Saiz, C. M. Wurster and A. McBeath, The Pyrogenic Carbon Cycle, Annu. Rev. Earth Planet. Sci., 2015, 43(1), 273–298 CrossRef CAS.
- G. Sigmund, C. Jiang, T. Hofmann and W. Chen, Environmental transformation of natural and engineered carbon nanoparticles and implications for the fate of organic contaminants, Environ. Sci.: Nano, 2018, 2500–2518 RSC.
-
J. H. Seinfeld and S. N. Pandis, Atmospheric Chemistry and Physics: From Air Pollution to Climate Change, Wiley, 2016 Search PubMed.
- N. Riemer, H. Vogel and B. Vogel, Soot aging time scales in polluted regions during day and night, Atmos. Chem. Phys., 2004, 4(7), 1885–1893 CrossRef CAS.
- H. Muckenhuber and H. Grothe, The heterogeneous reaction between soot and NO2 at elevated temperature, Carbon, 2006, 44(3), 546–559 CrossRef CAS.
- H. Muckenhuber and H. Grothe, A DRIFTS study of the heterogeneous reaction of NO2 with carbonaceous materials at elevated temperature, Carbon, 2007, 45(2), 321–329 CrossRef CAS.
- C. Chen and W. Huang, Aggregation Kinetics of Diesel Soot Nanoparticles in Wet Environments, Environ. Sci. Technol., 2017, 51(4), 2077–2086 CrossRef CAS PubMed.
- E. J. W. Verwey, N. Laboratorium and D. Waals, Theory of the Stability of Lyophobic Colloids, J. Phys. Colloid Chem., 1947, 51(3), 631–636 CrossRef CAS PubMed.
- B. Derjaguin and L. Landau, Theory of the stability of strongly charged lyophobic sols and of the adhesion of strongly charged particles in solutions of electrolytes, Prog. Surf. Sci., 1993, 43(1), 30–59 CrossRef.
- S. Bhattacharjee, C. Ko and M. Elimelech, DLVO Interaction between Rough Surfaces, Langmuir, 1998, 7463(26), 3365–3375 CrossRef.
- F. Schwab, L. Camenzuli, K. Knauer, B. Nowack, A. Magrez and L. Sigg,
et al., Sorption kinetics and equilibrium of the herbicide diuron to carbon nanotubes or soot in absence and presence of algae, Environ. Pollut., 2014, 192, 147–153 CrossRef CAS PubMed.
- T. H. Nguyen, I. Sabbah and W. P. Ball, Sorption nonlinearity for organic contaminants with diesel soot: method development and isotherm interpretation, Environ. Sci. Technol., 2004, 38(13), 3595–3603 CrossRef CAS PubMed.
- Z. Lu, J. K. MacFarlane and P. M. Gschwend, Adsorption of Organic Compounds to Diesel Soot: Frontal Analysis and Polyparameter Linear Free-Energy Relationship, Environ. Sci. Technol., 2016, 50(1), 285–293 CrossRef CAS PubMed.
- P.-H. Su, D. T. Fu Kuo, Y. Shih and C. Chen, Sorption of organic compounds to two diesel soot black carbons in water evaluated by liquid chromatography and polyparameter linear solvation energy relationship, Water Res., 2018, 144, 709–718 CrossRef CAS PubMed.
- T. Hüffer, S. Schroth and T. C. Schmidt, Influence of humic acids on sorption of alkanes by carbon nanotubes - implications for the dominant sorption mode, Chemosphere, 2015, 119, 1169–1175 CrossRef PubMed.
- M. Kah, X. Zhang and T. Hofmann, Sorption behavior of carbon nanotubes: changes induced by functionalization, sonication and natural organic matter, Sci. Total Environ., 2014, 497–498, 133–138, DOI:10.1016/j.scitotenv.2014.07.112.
- J. Y. Chen, W. Chen and D. Zhu, Adsorption of nonionic aromatic compounds to single-walled carbon nanotubes: effects of aqueous solution chemistry, Environ. Sci. Technol., 2008, 42(19), 7225–7230 CrossRef CAS PubMed.
- L. Wang, Y. Huang, A. T. Kan, M. B. Tomson and W. Chen, Enhanced Transport of 2,2′,5,5′-Polychlorinated Biphenyl by Natural Organic Matter (NOM) and Surfactant-Modified Fullerene Nanoparticles (nC60), Environ. Sci. Technol., 2012, 46(10), 5422–5429 CrossRef CAS PubMed.
- L. Hou, J. D. Fortner, X. Wang, C. Zhang, L. Wang and W. Chen, Complex interplay between formation routes and natural organic matter modification controls capabilities of C60 nanoparticles (nC60) to accumulate organic contaminants, J. Environ. Sci., 2017, 51, 315–323 CrossRef PubMed.
- A. Tsaboula, E. N. Papadakis, Z. Vryzas, A. Kotopoulou, K. Kintzikoglou and E. Papadopoulou-Mourkidou, Environmental and human risk hierarchy of pesticides: a prioritization method, based on monitoring, hazard assessment and environmental fate, Environ. Int., 2016, 91, 78–93 CrossRef CAS PubMed.
- National Institutes of Health - Health & Human Services, Hazardous Substances Data Bank (HSDB), 2019, https://toxnet.nlm.nih.gov/cgi-bin/sis/htmlgen?HSDB.
- S. Endo, P. Grathwohl, S. B. Haderlein and T. C. Schmidt, Effects of Native Organic Material and Water on Sorption Properties of Reference Diesel Soot, Environ. Sci. Technol., 2009, 43, 3187–3193 CrossRef CAS PubMed.
-
N. Ulrich, S. Endo, T. N. Brown, N. Watanabe, G. Bronner and M. H. Abraham, et al., UFZ-LSER database v 3.2, Helmholtz Zentrum für Umweltforschung - UFZ, Leipzig, Deutschland, 2017, http://www.ufz.de/lserd Search PubMed.
- A. Sadezky, H. Muckenhuber, H. Grothe, R. Niessner and U. Pöschl, Raman microspectroscopy of soot and related carbonaceous materials: spectral analysis and structural information, Carbon, 2005, 43(8), 1731–1742 CrossRef CAS.
- T. Boger, D. Rose, P. Nicolin, N. Gunasekaran and T. Glasson, Oxidation of Soot (Printex® U) in Particulate Filters Operated on Gasoline Engines, Emiss. Control Sci. Technol., 2015, 1(1), 49–63 CrossRef CAS.
- Y. Wei, Q. Zhang and J. E. Thompson, The Wetting Behavior of Fresh and Aged Soot Studied through Contact Angle Measurements, Atmos. Clim. Sci., 2017, 07(01), 11–22 CAS.
- G. Sigmund, T. Hüffer, T. Hofmann and M. Kah, Biochar total surface area and total pore volume determined by N2 and CO2 physisorption are strongly influenced by degassing temperature, Sci. Total Environ., 2017, 580, 770–775 CrossRef CAS PubMed.
-
OECD, Guideline 106 for the testing of chemicals - Adsorption-Desorption Using a Batch Equilibrium Method, 2000, pp. 1–44 Search PubMed.
- A. Clavier, A. Praetorius and S. Stoll, Determination of nanoparticle heteroaggregation attachment efficiencies and rates in presence of natural organic matter monomers. Monte Carlo modelling, Sci. Total Environ., 2019, 650, 530–540 CrossRef CAS PubMed.
- R. J. Williams, S. Harrison, V. Keller, J. Kuenen, S. Lofts and A. Praetorius,
et al., Models for assessing engineered nanomaterial fate and behaviour in the aquatic environment, Current Opinion in Environmental Sustainability, 2019, 36, 105–115 CrossRef.
- T. Hüffer, H. Sun, J. D. Kubicki, T. Hofmann and M. Kah, Interactions between aromatic hydrocarbons and functionalized C60 fullerenes – insights from experimental data and molecular modelling, Environ. Sci.: Nano, 2017, 4(5), 1045–1053 RSC.
- R. W. Coughlin and F. S. Ezra, Role of Surface Acidity in the Adsorption of Organic Pollutants on the Surface of Carbon, Environ. Sci. Technol., 1968, 2(4), 291–297 CrossRef CAS.
- T. J. Bandosz, J. Jagieƚƚo and J. A. Schwarz, Effect of Surface Chemical Groups on Energetic Heterogeneity of Activated Carbons, Langmuir, 1993, 9(10), 2518–2522 CrossRef CAS.
- H. H. Cho, B. A. Smith, J. D. Wnuk, D. H. Fairbrother and W. P. Ball, Influence of surface oxides on the adsorption of naphthalene onto multiwalled carbon nanotubes, Environ. Sci. Technol., 2008, 42(8), 2899–2905 CrossRef CAS PubMed.
- K. Yang and B. Xing, Adsorption of organic compounds by carbon nanomaterials in aqueous phase: Polanyi theory and its application, Chem. Rev., 2010, 110(10), 5989–6008 CrossRef CAS PubMed.
- S. E. Hale, H. P. H. Arp, D. Kupryianchyk and G. Cornelissen, A synthesis of parameters related to the binding of neutral organic compounds to charcoal, Chemosphere, 2016, 144, 65–74 CrossRef CAS PubMed.
- M. Kah, H. Sun, G. Sigmund, T. Hüffer and T. Hofmann, Pyrolysis of waste materials: characterization and prediction of sorption potential across a wide range of mineral contents and pyrolysis temperatures, Bioresour. Technol., 2016, 214, 225–233 CrossRef CAS PubMed.
- X. Zhang, M. Kah, M. T. O. Jonker and T. Hofmann, Dispersion state and humic acids concentration-dependent sorption of pyrene to carbon nanotubes, Environ. Sci. Technol., 2012, 46(13), 7166–7173 CrossRef CAS PubMed.
-
R. Salminen, M. J. Batista, M. Bidovec, A. Demetriades, B. De Vivo and W. De Vos, et al., Geochemical Atlas of Europe. Part 1: Background Information, Methodology and Maps - Statistical data of analytical results, 2005, p. 526 Search PubMed.
Footnote |
† Electronic supplementary information (ESI) available. See DOI: 10.1039/c9em00354a |
|
This journal is © The Royal Society of Chemistry 2019 |
Click here to see how this site uses Cookies. View our privacy policy here.