Emerging investigator series: towards a framework for establishing the impacts of pharmaceuticals in wastewater irrigation systems on agro-ecosystems and human health
Received
11th January 2019
, Accepted 21st March 2019
First published on 1st April 2019
Abstract
Use of reclaimed wastewater for agricultural irrigation is seen as an attractive option to meet agricultural water demands of a growing number of countries suffering from water scarcity. However, reclaimed wastewater contains pollutants which are introduced to the agro-environment during the irrigation process. While water reuse guidelines do consider selected classes of pollutants, they do not account for the presence of pollutants of emerging concern such as pharmaceuticals and the potential risks these may pose. Here we use source–pathway–receptor analysis (S–P–R) to develop a holistic framework for evaluating the impacts of pharmaceuticals, present in wastewater used for agricultural irrigation, on human and ecosystem health and evaluate the data availability for the framework components. The developed framework comprised of 34 processes and compartments but a good level of knowledge was available for only five of these suggesting that currently it is not possible to fully establish the impacts of pharmaceuticals in wastewater irrigation systems. To address this, work is urgently needed to understand the fate and transport of pharmaceuticals in arable soil systems and the effects of chronic low-level exposure to these substances on microbes, invertebrates, plants, wildlife and humans. In addition, research pertaining to the fate, uptake and effects of pharmaceutical mixtures and metabolites is lacking as well as data on bio-accessibility of pharmaceuticals after ingestion. Scientific advancements in the five areas prioritised in terms of future research are needed before we are able to fully quantify the agricultural and human health risks associated with reclaimed wastewater use.
Environmental significance
Reclaimed wastewater irrigation presents a route by which pharmaceuticals can enter, and become, omnipresent in agricultural systems. Due to the biological potency of pharmaceuticals, uptake into receptors such as plants, livestock, and wildlife presents a risk to agricultural and human health. A holistic risk framework that considers the sources of pharmaceuticals and the pathways by which these chemicals can impact receptors has been proposed. Source–pathway–receptor analysis revealed that it is currently impossible to fully understand the risks of pharmaceuticals in agricultural systems due to a number of significant knowledge gaps. By identifying and prioritising these knowledge gaps, we envisage these findings will inform future regulatory and policy developments around the management of pharmaceutical contamination of reclaimed wastewater.
|
Introduction and background
Water management is an issue of global concern; a recent World Bank report concluded that water scarcity, exacerbated by climate change, could cost some regions up to 6% of their GDP, spur migration and spark conflict.1 To meet the growing demand for water, treated wastewater (reclaimed wastewater) is sometimes reclaimed and used to irrigate agricultural land, golf courses and various other landscapes. Use of reclaimed wastewater is an especially attractive option in countries suffering from water shortages that have a typically warmer and dryer climate such as in the Middle East and Southern Europe.2–5 In Israel, for example, more than 85% of the produced reclaimed wastewater is currently used for irrigation. This accounts for over 50% of the total irrigation volume.6,7 Comparatively, use of reclaimed wastewater for agricultural irrigation in California only amounts for about 4% of the irrigation volume, but this has been increasing steadily. Due to anticipated water shortages, the state has developed a policy calling for a three-fold increase in the total reuse of reclaimed wastewater by 2030.8
Despite recent advances in technologies to treat wastewater, some pollutants of emerging concern such as pharmaceuticals and personal care products are not removed by wastewater treatment.9,10 These chemicals are frequently detected in both raw influent and treated effluents of wastewater treatment plants at concentrations ranging from ng L−1 to μg L−1.11,12 Concentrations of pharmaceuticals in wastewater vary across the globe. For example, the concentrations of a vast majority of antibiotics in effluents are generally higher in most Asian countries than those reported in European and North America10,13–15 with high concentrations explained by: high consumption and the fact that these compounds are readily available in these regions; and poor wastewater treatment technologies in some regions. In addition, different treatment technologies remove different chemicals to different extents thereby resulting in a range of concentrations in effluents. Concentrations of acetaminophen, for example, have been observed to range between below method detection limits to 62
000 ng L−1 across wastewater treatment plants sampled in North America and Asia.12
An increasing number of studies have documented the presence of a wide range pharmaceuticals destined for land application.16–18 With the anticipated future increases in reclaimed wastewater reuse expected on a global scale (e.g. FAO report “Coping with water scarcity: an action framework for agriculture and food security”),19 the introduction of pharmaceuticals to agro-environments is also expected to increase. At the EU level it has been acknowledged that we need to develop minimum requirements to manage the human and environmental risks from reclaimed wastewater to irrigate crops (COM(2018)337).20 Classification of reclaimed wastewater destined for agricultural irrigation has therefore been proposed and this is based on monitoring for the presence of pathogens and physico-chemical constituents that may pose a risk to human and environmental health, and to environmental matrices (e.g. E. coli, biological oxygen demand, turbidity, and suspended solids).21 The proposed quality requirements for the EU are similar to the WHO Guidelines for the Safe Use of Wastewater, Excreta and Greywater22 and the Australian Guidelines for Water Recycling (Phase 1)23 when wastewater is used to irrigate of urban, recreational and open space, and agriculture and horticulture.
While, in some regions, the human health risks of pharmaceuticals are accounted for when wastewater is used for drinking water purposes (e.g. Australian Guidelines for Water Reuse: Augmentation of Drinking Water Supplies),24 there are currently no quality standards with regards to concentrations of pharmaceuticals in reclaimed wastewater used for irrigation. Given the current demand, and potential for future widespread use of reclaimed wastewater, it is essential we are able to adequately assess this risk. In order to contribute to the safe reuse of reclaimed wastewater in agriculture, policies and guidelines may need to be updated, and mitigation measures put in place to minimise environmental and human health impacts. There is therefore a need to develop new frameworks for determining the impacts of pharmaceuticals present in wastewater irrigation systems on agro-ecosystem and human health. In this paper, we therefore present a Source–Pathway–Receptor (S–P–R) analysis to establish a framework to describe how pharmaceuticals originating from reclaimed wastewater could impact on human health and the health of agricultural systems. We then assess the availability of knowledge, data, models and methods required to populate different components of the S–P–R diagram.
Source–pathway–receptor analysis
The S–P–R diagram, consisting of 34 compartments (A–K), represents the different pathways in which agro-ecosystems are exposed to pharmaceuticals receiving wastewater irrigation (Fig. 1). The primary source of pharmaceuticals is reclaimed wastewater used as a source of irrigation (A; Fig. 1) according to agricultural water management systems (B; Fig. 1). This results in the contamination of a number of environmental compartments identified as the soil, surface water and groundwater (C, D and F; Fig. 1). A range of receptors can then be exposed to pharmaceuticals in these compartments through a variety of direct and indirect pathways. The main receptors identified include terrestrial wildlife, people, livestock, terrestrial plants, soil fauna and aquatic species (F–K; Fig. 1), with a number of receptors themselves identified as potential routes (secondary sources) of exposure for pharmaceutical contamination via food web transfer to higher vertebrates in the food chain (e.g. fish as a source of food for birds). Whilst this analysis considers the use of reclaimed wastewater as the route for pharmaceuticals to enter agroecosystems, the use of organic soil amendments (sludges and/or livestock manures) are also significant pathways by which pharmaceuticals can enter, and become omnipresent, in soils.25,26 A number of associated risks and knowledge gaps highlighted in this analysis are therefore also relevant to these additional pathways (Table 1).
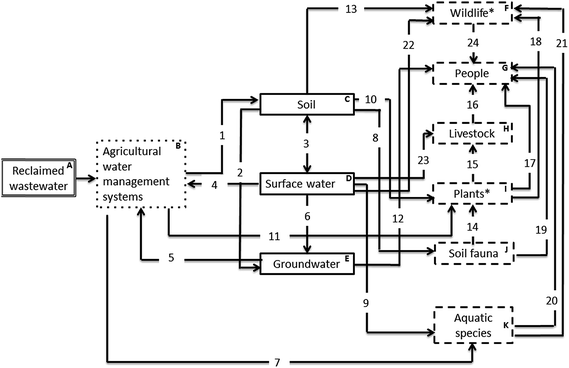 |
| Fig. 1 Source–pathway–receptor diagram to define how pharmaceuticals originating from reclaimed wastewater (source) can impact on human health and the health of agricultural systems via soil, surface water and ground water exposure (pathways). Receptors include wildlife, people, livestock, plants, soil fauna and aquatic species. *Wildlife and plants are terrestrial species for this analysis. | |
Table 1 Summary of existing knowledge and knowledge gaps pertaining to reclaimed wastewater irrigation practices (sources and pathways are denoted in bold, receptors are underlined and processes connecting the S–P–R components are italicised). Where existing knowledge is in place – example references are supplied to support this. Our knowledge pertaining to each compartment or process classified according to whether we have a ‘High’, ‘Moderate or ‘Low’ understanding of the issue
Number |
Description |
Existing knowledge |
Knowledge gaps |
Classification |
A |
Reclaimed wastewater
|
– Concentrations reported for many compounds33,35 |
– Models often limited by availability of input data (e.g. usage data, WWTP removal data) |
High |
– Good models to predict effluent concentrations38 |
– Future scenarios (e.g. impact of climate change) |
– Presence of metabolites not well characterised |
B |
Agriculture water management systems |
– Data available on what the systems are and where they are for select countries7 |
– Limited information of chemical fate processes during piping (e.g. anaerobic conditions, biofilm processes, chlorination, reservoir storage, effects of temperature) |
Moderate |
1 |
Irrigation
|
– Good data on irrigation practices for select countries (location, volumes, frequency)121 |
– Fate processes in drip irrigation systems |
High |
C |
Soil
|
– Experimental protocols exist to measure fate processes122 |
– Predictive models are poor if input data is missing |
Moderate |
– Measured data on fate (e.g. sorption, persistence) for many compounds51,52 |
– Bioavailability (pore water concentrations) |
– Models exist to predict soil concentrations and chemical fate processes123 |
– Monitoring data lacking for most compounds |
– Effect of reclaimed wastewater irrigation and hydroclimatic variables on soil fate processes |
– Measured data on biotic and/or transformation in soil for many compounds |
2 |
Leaching to groundwater
|
– Limited experimental data for a few compounds63 |
– No data for a majority of compounds |
Moderate |
– Models are available124,125 |
– Potential transformation processes during leaching |
E |
Groundwater compartment
|
– Aquifer maps exist126,127 |
– Limited information on chemical processes in the groundwater (including biotic and/or transformation) |
Moderate |
– Some monitoring data63,66,128 |
3 |
Runoff to surface waters
|
– Good hydrological understanding for many countries129 |
– Extreme events hard to predict (model scenarios not currently available) |
High |
– Experimental monitoring ongoing61,130 |
– Data on only a few compounds |
– Models are available131,132 |
D |
Surface water
|
– Monitoring data for many compounds33,35 |
– Monitoring efforts focussed on Europe and N. America |
Moderate |
– Data on in-stream processes are available for many compounds133,134 |
– Exposure models not available for specific scenarios (e.g. limited wastewater treatment) |
– Measured data on biotic and/or transformation in soil for many compounds |
4 and 5 |
Abstraction of contaminated surface and groundwater
|
– Data on volumes, locations, frequency where practices exist135 |
NA |
High |
6 |
Surface water to groundwater
|
NA |
– Very limited understanding |
Low |
7 |
Use of reclaimed wastewater for aquaculture |
– Known to be practiced in some areas136 |
– Limited knowledge on aquaculture practices (e.g. where, volumes of water used) |
Low |
8 |
Uptake into microbes
|
NA |
– Very limited understanding |
Low |
J |
![[E with combining low line]](https://www.rsc.org/images/entities/char_0045_0332.gif) ![[f with combining low line]](https://www.rsc.org/images/entities/char_0066_0332.gif) ![[f with combining low line]](https://www.rsc.org/images/entities/char_0066_0332.gif) ![[e with combining low line]](https://www.rsc.org/images/entities/char_0065_0332.gif) ![[c with combining low line]](https://www.rsc.org/images/entities/char_0063_0332.gif) ![[t with combining low line]](https://www.rsc.org/images/entities/char_0074_0332.gif) ![[o with combining low line]](https://www.rsc.org/images/entities/char_006f_0332.gif) ![[m with combining low line]](https://www.rsc.org/images/entities/char_006d_0332.gif) ![[i with combining low line]](https://www.rsc.org/images/entities/char_0069_0332.gif) ![[c with combining low line]](https://www.rsc.org/images/entities/char_0063_0332.gif) ![[r with combining low line]](https://www.rsc.org/images/entities/char_0072_0332.gif) ![[o with combining low line]](https://www.rsc.org/images/entities/char_006f_0332.gif) ![[b with combining low line]](https://www.rsc.org/images/entities/char_0062_0332.gif) ![[e with combining low line]](https://www.rsc.org/images/entities/char_0065_0332.gif) ![[s with combining low line]](https://www.rsc.org/images/entities/char_0073_0332.gif) |
– Knowledge of some effects on C and N transformation137,138 |
– No information on mechanisms |
Low |
– Limited molecular data139 |
– No data on many compounds and key endpoints |
– Studies are on-going |
– Effects of mixtures |
– Development and preservation of antimicrobial resistance |
9 |
Uptake into aquatic species
|
– Limited data for some species115,117 |
– Models and measured data for invertebrates are lacking |
Low |
– Some models are available (and account for ionisation)140–142 |
– Lots of compounds have no data |
– Experimental protocols exist143 |
K |
![[E with combining low line]](https://www.rsc.org/images/entities/char_0045_0332.gif) ![[f with combining low line]](https://www.rsc.org/images/entities/char_0066_0332.gif) ![[f with combining low line]](https://www.rsc.org/images/entities/char_0066_0332.gif) ![[e with combining low line]](https://www.rsc.org/images/entities/char_0065_0332.gif) ![[c with combining low line]](https://www.rsc.org/images/entities/char_0063_0332.gif) ![[o with combining low line]](https://www.rsc.org/images/entities/char_006f_0332.gif) ![[a with combining low line]](https://www.rsc.org/images/entities/char_0061_0332.gif) ![[q with combining low line]](https://www.rsc.org/images/entities/char_0071_0332.gif) ![[u with combining low line]](https://www.rsc.org/images/entities/char_0075_0332.gif) ![[a with combining low line]](https://www.rsc.org/images/entities/char_0061_0332.gif) ![[t with combining low line]](https://www.rsc.org/images/entities/char_0074_0332.gif) ![[i with combining low line]](https://www.rsc.org/images/entities/char_0069_0332.gif) ![[s with combining low line]](https://www.rsc.org/images/entities/char_0073_0332.gif) ![[p with combining low line]](https://www.rsc.org/images/entities/char_0070_0332.gif) ![[e with combining low line]](https://www.rsc.org/images/entities/char_0065_0332.gif) ![[c with combining low line]](https://www.rsc.org/images/entities/char_0063_0332.gif) ![[i with combining low line]](https://www.rsc.org/images/entities/char_0069_0332.gif) ![[e with combining low line]](https://www.rsc.org/images/entities/char_0065_0332.gif) ![[s with combining low line]](https://www.rsc.org/images/entities/char_0073_0332.gif) |
– Some data available for acute and chronic effects (mostly fish) with a focus on effects of hormones on the reproductive system in fish144,145 |
– Lots of compounds have no data |
Moderate |
– Some models are available146,148 |
– Influence of food chain transfer of chemicals |
– Effects of mixtures |
– Antimicrobial resistance |
10 |
Uptake from soil into terrestrial plants
|
– Data available for some compounds in some plants and soils73–75 |
– Models not designed for pharmaceuticals |
Low |
– Models exist for uptake of organic compounds into plants149–151 |
– Data lacking for most compounds at environmentally relevant concentrations (μg kg−1) |
– Limited number of plants studied |
– Transformation products/in-plant metabolism |
– Limited data on distribution in plant |
– Multi-generational exposures |
11 |
Foliar plant uptake
|
– Data exists for a few compounds72 |
– Models not available for pharmaceuticals |
Low |
– Processes not understood |
I |
![[E with combining low line]](https://www.rsc.org/images/entities/char_0045_0332.gif) ![[f with combining low line]](https://www.rsc.org/images/entities/char_0066_0332.gif) ![[f with combining low line]](https://www.rsc.org/images/entities/char_0066_0332.gif) ![[e with combining low line]](https://www.rsc.org/images/entities/char_0065_0332.gif) ![[c with combining low line]](https://www.rsc.org/images/entities/char_0063_0332.gif) ![[t with combining low line]](https://www.rsc.org/images/entities/char_0074_0332.gif) ![[o with combining low line]](https://www.rsc.org/images/entities/char_006f_0332.gif) ![[t with combining low line]](https://www.rsc.org/images/entities/char_0074_0332.gif) ![[e with combining low line]](https://www.rsc.org/images/entities/char_0065_0332.gif) ![[r with combining low line]](https://www.rsc.org/images/entities/char_0072_0332.gif) ![[r with combining low line]](https://www.rsc.org/images/entities/char_0072_0332.gif) ![[e with combining low line]](https://www.rsc.org/images/entities/char_0065_0332.gif) ![[s with combining low line]](https://www.rsc.org/images/entities/char_0073_0332.gif) ![[t with combining low line]](https://www.rsc.org/images/entities/char_0074_0332.gif) ![[r with combining low line]](https://www.rsc.org/images/entities/char_0072_0332.gif) ![[i with combining low line]](https://www.rsc.org/images/entities/char_0069_0332.gif) ![[a with combining low line]](https://www.rsc.org/images/entities/char_0061_0332.gif) ![[p with combining low line]](https://www.rsc.org/images/entities/char_0070_0332.gif) ![[l with combining low line]](https://www.rsc.org/images/entities/char_006c_0332.gif) ![[a with combining low line]](https://www.rsc.org/images/entities/char_0061_0332.gif) ![[n with combining low line]](https://www.rsc.org/images/entities/char_006e_0332.gif) ![[t with combining low line]](https://www.rsc.org/images/entities/char_0074_0332.gif) ![[s with combining low line]](https://www.rsc.org/images/entities/char_0073_0332.gif) |
– Limited data on effects90,95 |
– Limited data on most compounds at environmentally relevant concentrations |
Low |
– Effects of transformation products |
– Mechanisms not understood |
– Long term effects on plant productivity (sub-lethal effects) |
– Multi-generational exposures |
12 |
Consumption of drinking water
|
– Data on water consumption per capita and by livestock152,153 |
NA |
High |
G |
![[H with combining low line]](https://www.rsc.org/images/entities/char_0048_0332.gif) ![[u with combining low line]](https://www.rsc.org/images/entities/char_0075_0332.gif) ![[m with combining low line]](https://www.rsc.org/images/entities/char_006d_0332.gif) ![[a with combining low line]](https://www.rsc.org/images/entities/char_0061_0332.gif) ![[n with combining low line]](https://www.rsc.org/images/entities/char_006e_0332.gif) |
– Calculated permissible uptake (e.g. ADI, AOELs, TTC)70,73 |
– Long term, low level exposure |
Moderate |
– Plasma therapeutic concentrations154 |
– Mixtures |
– Health effects and side effects data (PK/PD)155 |
– Sensitive sub-populations |
– Metabolism data/drug–drug interactions157 |
– Metabolism at low concentrations |
– Mammalian toxicity data34,146 |
– Occupational exposure157 |
13 |
Soil to terrestrial wildlife
|
– Limited information for some pharmaceuticals69,158 |
– No data for most compounds |
Low |
– Simple models available (via earthworm)109,159 |
– Diets and routes of exposure poorly understood |
– Bioaccessibility |
F |
![[E with combining low line]](https://www.rsc.org/images/entities/char_0045_0332.gif) ![[f with combining low line]](https://www.rsc.org/images/entities/char_0066_0332.gif) ![[f with combining low line]](https://www.rsc.org/images/entities/char_0066_0332.gif) ![[e with combining low line]](https://www.rsc.org/images/entities/char_0065_0332.gif) ![[c with combining low line]](https://www.rsc.org/images/entities/char_0063_0332.gif) ![[t with combining low line]](https://www.rsc.org/images/entities/char_0074_0332.gif) ![[o with combining low line]](https://www.rsc.org/images/entities/char_006f_0332.gif) ![[t with combining low line]](https://www.rsc.org/images/entities/char_0074_0332.gif) ![[e with combining low line]](https://www.rsc.org/images/entities/char_0065_0332.gif) ![[r with combining low line]](https://www.rsc.org/images/entities/char_0072_0332.gif) ![[r with combining low line]](https://www.rsc.org/images/entities/char_0072_0332.gif) ![[e with combining low line]](https://www.rsc.org/images/entities/char_0065_0332.gif) ![[s with combining low line]](https://www.rsc.org/images/entities/char_0073_0332.gif) ![[t with combining low line]](https://www.rsc.org/images/entities/char_0074_0332.gif) ![[r with combining low line]](https://www.rsc.org/images/entities/char_0072_0332.gif) ![[i with combining low line]](https://www.rsc.org/images/entities/char_0069_0332.gif) ![[a with combining low line]](https://www.rsc.org/images/entities/char_0061_0332.gif) ![[w with combining low line]](https://www.rsc.org/images/entities/char_0077_0332.gif) ![[i with combining low line]](https://www.rsc.org/images/entities/char_0069_0332.gif) ![[l with combining low line]](https://www.rsc.org/images/entities/char_006c_0332.gif) ![[d with combining low line]](https://www.rsc.org/images/entities/char_0064_0332.gif) ![[l with combining low line]](https://www.rsc.org/images/entities/char_006c_0332.gif) ![[i with combining low line]](https://www.rsc.org/images/entities/char_0069_0332.gif) ![[f with combining low line]](https://www.rsc.org/images/entities/char_0066_0332.gif) ![[e with combining low line]](https://www.rsc.org/images/entities/char_0065_0332.gif) |
– Some data available for earthworms160 |
– No data for most compounds |
Low |
– Mammalian industry data available (rodents)34,146 |
– Sub-therapeutic doses and effects |
– Bird toxicity data exists if it is a veterinary drug161 |
– Long-term exposure and distribution in wildlife |
– Effects of mixtures and transformation products |
14 |
Microbe plant interactions
|
– Importance of microbes for plant systems162 |
NA |
Moderate |
– Information on soil microbiome100,163 |
15 |
Plant consumption by livestock
|
– General dietary information |
– Dietary information for different species |
Low |
– Some concentration data in forage crops164 |
– Effects of crop processing |
– Bioaccessibility |
H |
![[E with combining low line]](https://www.rsc.org/images/entities/char_0045_0332.gif) ![[f with combining low line]](https://www.rsc.org/images/entities/char_0066_0332.gif) ![[f with combining low line]](https://www.rsc.org/images/entities/char_0066_0332.gif) ![[e with combining low line]](https://www.rsc.org/images/entities/char_0065_0332.gif) ![[c with combining low line]](https://www.rsc.org/images/entities/char_0063_0332.gif) ![[t with combining low line]](https://www.rsc.org/images/entities/char_0074_0332.gif) ![[o with combining low line]](https://www.rsc.org/images/entities/char_006f_0332.gif) ![[l with combining low line]](https://www.rsc.org/images/entities/char_006c_0332.gif) ![[i with combining low line]](https://www.rsc.org/images/entities/char_0069_0332.gif) ![[v with combining low line]](https://www.rsc.org/images/entities/char_0076_0332.gif) ![[e with combining low line]](https://www.rsc.org/images/entities/char_0065_0332.gif) ![[s with combining low line]](https://www.rsc.org/images/entities/char_0073_0332.gif) ![[t with combining low line]](https://www.rsc.org/images/entities/char_0074_0332.gif) ![[o with combining low line]](https://www.rsc.org/images/entities/char_006f_0332.gif) ![[c with combining low line]](https://www.rsc.org/images/entities/char_0063_0332.gif) ![[k with combining low line]](https://www.rsc.org/images/entities/char_006b_0332.gif) |
– Some data exists if they are veterinary pharmaceuticals |
– Sub-therapeutic doses |
Low |
– Mammalian toxicity data available (rodents)34,146 |
– Long-term exposure |
– Mixtures and transformation products |
– Data lacking for most compounds |
16 |
Livestock to human
|
– Dietary information165 |
– Cooking/processing/storage effects |
Moderate |
– Dietary information for sub-populations is limited |
– Bioaccessibility |
17 |
Plant consumption by humans
|
– General dietary information161,166 |
– Dietary information for sub-populations |
Moderate |
– Some concentration data103 |
– Effects of cooking |
– Bioaccessibility |
– Trade of food (source) |
– Concentrations in edible part unknown for many crops |
18 |
Plant consumption by terrestrial wildlife
|
– Some data for birds and mammals167,168 |
– Very little known for many species |
Low |
– Bioaccessibility |
19 |
Microbes provide an ecosystem service to humans
|
– Importance known169 |
– AMR transfer |
Moderate |
20 |
Consumption of fish by humans
|
– Limited monitoring data on levels in fish consumed by humans170,171 |
– Dietary information for range of population (proportion of diet) |
Low |
– Could be high risk for small parts of the population |
21 |
Consumption of fish by terrestrial wildlife
|
– Feeding patterns of wildlife167,168 |
– Bioaccessibility and uptake |
Low |
– Some models are available109 |
– Species differences |
22 and 23 |
Consumption of surface water by wildlife and livestock
|
– Feeding patterns of wildlife and livestock167,168 |
– Not quantified for pharmaceuticals |
Moderate |
– Good ecological data172 |
24 |
Consumption of wildlife by humans
|
– General dietary information for humans161,166 |
– Not quantified for pharmaceuticals |
Low |
– Bioaccessibility |
– Could be high risk for small parts of the population |
Knowledge and data availability
Sources (A–B).
Reclaimed wastewater has been used as a source of agricultural irrigation in Asia, the Mediterranean and other arid and semi-arid regions for centuries (A; Fig. 1 and 2). Where there are policies in place to ensure food security and sustainable water management, the use of reclaimed wastewater comes under the broad umbrella of Agricultural Water Management Systems (B; Fig. 1). For example, Israel has one of the largest water recycling initiatives, where the use of reclaimed wastewater, comes under the Long Term National Water Sector Master Plan (LTN-MP).7 Direct use of reclaimed wastewater varies on a country-by-country basis. For example, it was estimated that reclaimed wastewater in China accounted for 1.26 × 109 m3 of agricultural irrigation water in 2013, which was approximately 10 times greater than reclaimed wastewater use in the United Arab Emirates at 0.14 × 109 m3.27
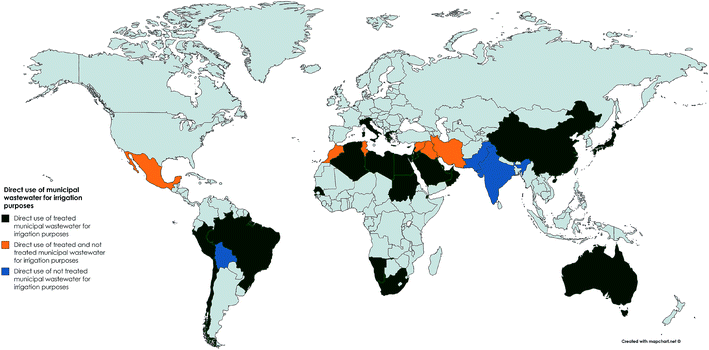 |
| Fig. 2 Direct use of municipal wastewater for agricultural irrigation purposes (data collated from AQUASTAT publications).27 | |
In an attempt to augment growing water demands, untreated wastewater is also used as a source of irrigation where wastewater infrastructure is lacking or where wastewater has undergone little to no treatment. For example in Mexico approximately 2
60
000 ha are irrigated with wastewater, most of which is untreated.2 There is an urgent need to identify where raw or partially treated wastewater is being used in agriculture to enable the risks to be properly assessed given that estimates indicate that at least 20 million hectares in 50 countries are irrigated with raw or partially wastewater.28,29
Through global monitoring campaigns we have a good understanding of the typical concentrations of pharmaceuticals in wastewater treatment plant (WWTP) effluent for some regions.30–32 According to a recent report by aus van der Beek,33 pharmaceuticals identified in treated wastewater comprise 28% of all global monitoring data with concentrations of a single pharmaceutical being reported up to 43
900 μg L−1. However, of the approximately 1500 pharmaceuticals estimated to be currently in use,34 monitoring studies have only identified ∼550 active pharmaceutical ingredients in wastewater effluent (according to data reported on UBA Database: Pharmaceuticals in the Environment) (Table 2).33,35 In addition, monitoring studies have primarily centred on the quantification of pharmaceutical parent compounds with little effort made to identify the presence of metabolites and other transformation products.36,37 To add further complexity, for some geographic regions there is also limited or non-existent monitoring data on concentrations of pharmaceuticals in wastewater.33 If we do not have a clear picture of the quantities and types of pharmaceuticals and their transformation products in reclaimed wastewater this poses a major challenge when trying to assess the global risks associated with wastewater reuse in agriculture.
Table 2 Occurrence of pharmaceuticals in the natural environment, aquatic species, plants, wildlife, livestock and people from published studies
Therapeutic class |
Rec. waste-water (A) |
Soil (C) |
Surface water (D) |
Ground-water (E) |
Wildlife (F) |
People (G) |
Livestock (H)a |
Plants (I) |
Soil fauna (J) |
Aquatic species (K) |
Examples of monitored drugs |
Accumulation in livestock may also occur through veterinary use.
|
Analgesic |
✓ |
✓ |
✓ |
✓ |
— |
— |
— |
✓ |
— |
✓ |
Acetaminophen |
Antibiotic |
✓ |
✓ |
✓ |
✓ |
— |
— |
— |
✓ |
— |
✓ |
Ciprofloxacin, clarithromycin |
Antidepressant |
✓ |
✓ |
✓ |
✓ |
— |
— |
— |
✓ |
— |
✓ |
Fluoxetine |
Antidiabetic |
✓ |
|
✓ |
✓ |
— |
— |
— |
✓ |
— |
✓ |
Metformin |
Antiepileptic |
✓ |
✓ |
✓ |
✓ |
— |
✓ |
— |
✓ |
— |
✓ |
Carbamazepine, lamotrigine |
Antihypertensive |
✓ |
✓ |
✓ |
✓ |
— |
— |
— |
✓ |
— |
✓ |
Atenolol, metoprolol |
Anti-inflammatory |
✓ |
✓ |
✓ |
✓ |
✓ |
— |
— |
✓ |
— |
✓ |
Naproxen, diclofenac |
Antineoplastic |
✓ |
— |
✓ |
✓ |
— |
— |
— |
— |
— |
✓ |
Carboplatin, 5-fluorouracil |
Antipsychotic |
✓ |
— |
✓ |
✓ |
— |
— |
— |
✓ |
— |
✓ |
Diazepam, oxazepam |
Antiviral |
✓ |
— |
✓ |
✓ |
— |
— |
— |
— |
— |
— |
Nevirapine, zidovudine |
Fibrates |
✓ |
✓ |
✓ |
✓ |
— |
— |
— |
✓ |
— |
✓ |
Gemfibrozil, bezafibrate |
Example references |
12, 32 and 173
|
47 and 48
|
33 and 35
|
62 and 174
|
113
|
103
|
N/A |
6, 70, 75 and 104
|
N/A |
115–117
|
— |
Predictive modelling approaches, such as the multimedia box model SimpleTreat, offer a means of generating data on concentrations of pharmaceuticals in wastewater effluent by estimating chemical fate in activated sludge WWTPs.38 A recent evaluation found that, in general, SimpleTreat 4.0 was able to predict concentrations of pharmaceuticals in effluents to within a factor of 10 of measured concentrations.39 However, predictive approaches such as this typically require a large amounts of input data, for example information on pharmaceutical usage, sorption to sludge, and degree of chemical biodegradability. The availability of this data, which currently exists for a limited number of compounds, constrains model use in the first instance. On-going research to develop Quantitative Structure Property Relationships (QSPRs) to describe pharmaceutical sludge sorption and biodegradability40,41 and high-throughput approaches to identify transformation products42 will enable us to better model wastewater effluent concentrations in the future. In addition, models such as SimpleTreat are parametrised under the assumption that the WWTP is functioning correctly, and in some regions (e.g. Palestine), this is probably not the case, which will alter concentrations of pharmaceuticals in wastewater and thus the exposure to the agro-environment. We need to therefore understand the performance of different treatment technologies in different regions and further develop models to account for these differences.
In order to regulate peak demands as well as fluctuations in effluent flow, reclaimed wastewater is often stored in a reservoir and the influence of storage on chemical fate for a range of pharmaceuticals is largely unknown.43,44 However, published models that account for dissipation in water could be utilised to explore this further in combination with improved QSPRs for persistence in the water column and a greater understanding of reclaimed wastewater storage e.g. depth of reservoir, residence time in reservoir. In addition, research is needed to explore the chemical fate processes of pharmaceuticals during pipe flow to agricultural fields and during the drip irrigation process itself (Fig. 1; pathway 1). Recent research has established that biofilms can form around and within the drip irrigation devices,45 which have the potential to influence pharmaceutical retardation and/or degradation however very little is known about these processes.
Compartments (C–E).
Reclaimed wastewater irrigation can result in the contamination of a number of environmental compartments including soils, surface water and groundwater (Table 2, Fig. 1). Continuous application of reclaimed wastewater to land has resulted in pharmaceuticals building up to detectable concentrations in soils (up to ca. 15 μg kg−1) (Fig. 1; pathway 1).46–48 Despite analytical challenges associated with quantifying pharmaceuticals in complex environmental samples49 (e.g. matrix interference, validated extraction methodologies) we have a good understanding of the fate of many pharmaceuticals in soils. A combination of experimental studies and modelling approaches have explored processes such as the sorption, leaching and degradation of pharmaceuticals.50–52 However, these efforts have typically focussed on the direct application of pharmaceuticals to soil and neglected to account for the presence of the reclaimed wastewater. The degradation of a limited number of pharmaceuticals in reclaimed wastewater-irrigated soils have so far been investigated.17,48,53 Treated effluents have been shown to increase the mobility of weakly acidic pharmaceuticals16 with microbial activity, dissolved matter, nutrients and particulate matter in reclaimed wastewater observed to affect the half-life of pharmaceuticals.54 However, further analysis is needed to explore the discrepancy between laboratory studies and results from field experiments when evaluating the fate of the pharmaceuticals in soils. Pharmaceuticals, such as sulfamethoxazole, are increasingly observed to persist in the field47 for much longer than expected based on half-lives generated from laboratory experiments would predict.51,55
Pollutants themselves can also alter soil microbial communities (as discussed under ‘Receptor’ section below) which have the potential to affect chemical degradability.52 This highlights the need to consider the composition of reclaimed wastewater and the alteration of the “reactivity” of soil microbial communities when evaluating the fate of pharmaceuticals after land application as these factors can have indirect effects on the fate of pollutants. In addition, long term reclaimed wastewater irrigation can lead to an alteration of soil properties (e.g. pH, heavy metals and nutrient content) which can in turn affect the fate of soil pollutants and this is something we know very little about.
Research has already highlighted the significant role soil properties play in the sorption and degradation of pharmaceuticals, and in particular ionisable pharmaceuticals (e.g. organic carbon content, cation exchange capacity, mineral content).51,52 With soil properties known to vary on a global scale (e.g. soil pH observed to range between 3.0 and 10.6 (ref. 56)), it is therefore imperative that future work to develop models to simulate fate processes in soils builds on recent research efforts such as the work of Droge and Goss57,58 and Franco et al.,41 to account for the significant role soil properties play in the fate of pharmaceuticals. In addition, fate modelling of pharmaceuticals should consider soil, hydrological and climatic factors that will alter in the future in response to a changing climate.
In addition to detecting pharmaceuticals in soils, monitoring campaigns have quantified the presence of pharmaceuticals in surface waters across the globe, however it is assumed this largely originates from the direct release of WWTP effluent.59,60 A small number of studies have demonstrated the mobilisation of pharmaceuticals following biosolids application to land25,61 however much less is known about the contribution of surface run-off to the contamination of water bodies after treated wastewater irrigation (pathway 3).18 Pharmaceuticals can also migrate from soils and contaminate groundwater supplies via leaching after reclaimed wastewater irrigation, with reported concentrations in groundwater typically being in the range of low ng L−1 (Fig. 1; pathway 2).53,62,63 However our understanding of the potential for the migration of pharmaceuticals to groundwater is primarily limited to a small number of soil column leaching experiments.64 For example, monitoring from the Penn State Living Filter suggests a clear indication of wastewater contribution to groundwater although concentrations were typically one to two orders of magnitude lower in comparison to WWTP effluent.63 This would suggest that soil has a limited capacity to act as a biogeochemical filter before the wastewater recharges underlying aquifers. More research is needed to understand the spatial–temporal factors influencing groundwater contamination including an evaluation of the potential pathway for pharmaceuticals to migrate to surface water from contaminated groundwater. Models have been developed to capture the transport of plant protection chemicals through the soil profile leaching into groundwater (e.g. FOCUS PEARL), but these models were not specifically parameterised for pharmaceuticals. Given the chemical similarity between plant protection products and pharmaceuticals (e.g. molecular weight, pKa within ionisable range) it is expected that these models will provide a good estimation as to the fate and transport of pharmaceuticals in soil. These models may however need to be refined to account for the effect of the reclaimed wastewater matrix on pharmaceutical transport. Studies on veterinary pharmaceuticals in soils have shown that these models under predict exposure, possibly due to colloid-facilitated transport of the pharmaceuticals.65
As well as reclaimed wastewater, surface and ground waters can also be used as a source of irrigation water thereby transferring these contaminants back into the soil environment. Abstraction of contaminated ground and surface water for irrigation is generally well characterised where such practices exist (Fig. 1; pathway 4 and 5), however our understanding of the transfer of pharmaceuticals from surface water to ground water via infiltration is limited to a select number of chemicals (Fig. 1; pathway 6).66 Heberer et al., demonstrated that whilst bank filtration can decrease the concentration of certain pharmaceuticals (dilution and/or removal) pharmaceutically active substances are still present in sampled ground waters at bank filtration sites.67 Similarly, the use of reclaimed wastewater to support aquaculture is known to be practiced68 although we have limited understanding of the specific locations and volumes of water used which presents a challenge with regards to quantifying the presence of pharmaceuticals in these systems (Fig. 1; pathway 7).
Receptors (F–K).
Contamination of soil, surface and ground water presents a risk to receptors which feed on, or inhabit, these compartments. The main receptors considered in this analysis include humans, terrestrial wildlife, livestock, terrestrial plants, aquatic species and the soil microbial community (Fig. 1). The biological potency of pharmaceuticals means that the occurrence of these chemicals in agro-ecosystems has the potential to induce toxic effects in these non-target organisms.69 To meet the growing demand for water and to ensure sustainable use of reclaimed wastewater irrigation it is imperative to understand the pathways by which these receptors are exposed (Fig. 1, Table 1), the propensity for accumulation as well as the levels at which effects are observed. Research efforts to answer these questions are summarised below.
Terrestrial plants.
A range of different crops have been shown to accumulate pharmaceuticals from soil (Fig. 1; pathway 10), including root vegetables (e.g. carrots, radish), fruits (e.g. tomatoes, cucumber), leaves (e.g. lettuce, ryegrass) and grains (e.g. wheat).70–72 However realistic exposure studies, for example field trials using environmentally relevant irrigation regimes are limited in number.72–75 Uptake into a range of plant components has been studied, however we know very little about uptake into crops native to arid and semi-arid countries where reclaimed wastewater irrigation is widely practiced such as the Middle East where olive and fig trees are frequently irrigated with reclaimed wastewater. We also have a limited understanding of the uptake of pharmaceuticals into plants via foliar application of wastewater (Fig. 1; pathway 11) with previous research primarily focusing on quantifying uptake from soil. However, overhead irrigation should not be neglected as this has recently been shown to substantially increase pharmaceutical residues in lettuce leaves compared to surface irrigation of soils for a select number of pharmaceuticals.76
Similarly to the quantification of pharmaceuticals in soils there are several challenges associated with the extraction and detection of pharmaceuticals in plant samples including the identification of metabolites.77 Microbial driven processes can result in the formation of transformation products in soil,78 as well as their presence resulting from the direct application of wastewater containing metabolites formed in the patient or transformation products formed in the treatment process.48 Publications are also beginning to document in-plant metabolism/transformation of pharmaceuticals, particularly for the antiepileptic compound, carbamazepine.6,79–82 Data generated to date show that metabolites can be present in plants at levels similar to or greater than the parent compound.83 It is important to understand the transformation of a wider range of pharmaceuticals in soil-plant systems because the structure and polarity of metabolites can be drastically different from their parent compounds, and therefore it is expected that their fate, uptake and toxicity will be different.77
Models exist to predict transport and whole plant allocation of organic chemicals, including uptake from soil and following foliar application.84–87 However, often these models, which are either simple correlations with compound properties or more complex compartmental models, do often not account for the complexity of the factors and processes determining pharmaceutical uptake, including chemical speciation, in-plant metabolism and differences in plant physiology. Given the widespread use of reclaimed wastewater, containing a range of pharmaceuticals to irrigate a globally diverse set of crops, it is impossible to gather experimental data for all these scenarios. It is therefore essential that new models, that cover a range of plant traits and exposure scenarios, are developed to predict the uptake of pharmaceuticals into plants, so we can adequately assess the risks arising from this accumulation. The development of models for individual compound classes and for separate plant species may therefore represent a promising approach for future model development.88
Due to the biological potency of pharmaceuticals and their metabolites, accumulation of these chemicals in plants presents a risk to the health of the plant directly as well as to the organisms that feed on the plant material, including humans and terrestrial wildlife. For example, exposure of plants to antibiotics has been shown to affect plant biomass.89–91 In addition to visible whole-plant morphological symptoms, pharmaceuticals have also been shown to have the potential to affect in-plant homeostasis, such as changes in phytohormones, cellular metabolism, nutrient uptake and signaling without phenotypic change.92–95 These changes at the subcellular scale and molecular level may be considered as the underlying mechanisms for the long-term visual phytotoxic responses, e.g., plant biomass effects. It is unsurprising that pharmaceutical induced effects have been observed given that these chemicals are designed to interact with specific molecular targets in humans and these targets have orthologs that are conserved in other species e.g. 20–25% the drug targets in humans had predicted orthologs in plants.96 Specifically, common receptors have been identified in plants for a number of antibiotics affecting plant physiological responses (e.g. chloroplast replication).97 We need to understand the mechanisms driving these effect responses, whether they are a direct interaction between the chemical and a receptor or an indirect effect of the chemical affecting soil microbial homeostasis which is in turn affecting plant health (pathway 14; see below for more detail on soil microbial communities).
Soil fauna.
As well as the potential to affect plants, prolonged effluent irrigation has been observed to effect the soil microbial community (Fig. 1; pathway 8).98,99 Research has primarily focussed on the effects of antibiotics, with publications documenting an inhibition of microbial activity,100 and significant dose-related effects on the soil microbial community function.101 More research is needed to understand the effects of a wider suite of pharmaceuticals as well as the effects of mixtures on soil microbial communities and the recovery of soil microbial function in response to pharmaceutical dissipation in soil. It is also known that soil microbes provide an ecosystem service to humans however we know very little about the development, preservation and transfer of antimicrobial resistance via this route (Fig. 1; pathway 19).102
People.
Accumulation of pharmaceuticals in edible crops presents a risk to humans that feed on reclaimed wastewater produce (Fig. 1; pathway 17). Paltiel et al.103 were the first to detect pharmaceutical residues in the urine of individuals consuming crops irrigated with reclaimed wastewater. Whilst this study clearly shows that humans can metabolise pharmaceuticals in plant material, the human health risk from ingesting this contaminated produce is largely debated. A number of studies have concluded that the risk to humans is negligible, with concentrations in edible plant tissue typically below permissible thresholds (e.g. acceptable daily intake (ADI)).70,104,105 However, for certain pharmaceuticals, accumulation in edible plant organs has the potential to reach calculated toxicity thresholds.73,106 Ingestion of even low doses of pharmaceuticals may be a significant issue for sensitive populations (e.g. elderly, children, pregnant women), individuals with allergies to particular medication, as well as increase the potential for contraindications between prescribed treatments and chemicals consumed in crops. With regards to antibiotics in particular, Williams-Nguyen et al.102 highlighted that globally there is a lack of data on human exposure to antimicrobial resistance in agro-environments which may result in a wider health issue. Ultimately, we know very little about the human health risks of consuming wastewater irrigated produce over the long-term. However, pharmaceuticals are arguably one of the most data rich groups of chemicals in terms of mammalian toxicology. There is a wealth of data on pharmaceutical therapeutic effects and occupational exposure limits and we need to exploit this, together with chemical read-across, to derive chemical specific ADIs, to better understand the human health risks of ingesting crops contaminated with pharmaceuticals. In addition to the consumption of contaminated crops, people are at risk of ingesting meat, fish, wildlife and drinking water contaminated with contaminants of emerging concern (Fig. 1; pathways 12, 16 and 24), however human exposure from multiple contaminated sources such as this is rarely considered in risk assessment paradigms. Future research to evaluate the risks of ingesting pharmaceutical contaminated produce can utilise previously published approaches to assess the risks of dietary exposure (e.g. for pesticides) and incorporate dietary information and food sourcing information to generate an accurate assessment of pharmaceutical exposure.
Terrestrial wildlife.
Wildlife species considered in this scenario includes soil invertebrates, birds and small mammals. Studies have so far identified that earthworms can accumulate pharmaceutical residues from soils, however research into other soil invertebrates is lacking.107,108 Accumulation of pharmaceuticals into species at the base of the food chain, such as earthworms, presents a potential risk to top predators, which feed on these organisms (Fig. 1; pathway 13). Our traditional approach to assessing food web transfer of chemical contaminants consists of simplistic exposure scenarios109 where exposure originates from a single contaminated prey source. However, top predators such as birds are exposed to pharmaceuticals from multiple sources including ingestion of contaminated crops (Fig. 1; pathway 18), fish (Fig. 1; pathway 21) and surface water (Fig. 1; pathway 22) and risk assessment needs to account for these more complex exposure scenarios. We also need to further our understanding of the bioaccessibility of pharmaceuticals in wildlife; perhaps building on recent work that developed an in vitro gastrointestinal tract model to compare the bioaccessibility of the antidepressant fluoxetine from invertebrate prey for birds and mammals using.110
Research regarding potential effects in non-target terrestrial wildlife remains scarce,111,112 with minimal data on long term exposure and distribution in higher vertebrates as well as the effects of pharmaceutical mixtures and transformation products.69 Given that the decline of the Asian vulture population has been attributable to exposure of a commonly prescribed non-steroidal anti-inflammatory, diclofenac,113 and the current lack of exposure and effects data in this area, research efforts are needed to evaluate pharmaceutical contaminants in terrestrial wildlife systems. Specifically, we need to advance our understanding of biomagnification and the effects of pharmaceuticals in food webs.
Livestock.
Livestock can be exposed to pharmaceuticals by ingesting reclaimed wastewater irrigated crops as part of their diet (Fig. 1; pathway 15) as well as using contaminated surface water as a source of drinking water (Fig. 1; pathway 23). Even though these pathways have been identified, we have a limited understanding of the bio-accessibility of pharmaceuticals (and their metabolites) in livestock and potential effects of long-term exposure to these chemicals. We have an opportunity to utilise existing modelling approaches developed for assessing livestock exposure to persistent organic pollutants.114 Use of these models in combination with mammalian toxicology data could enable progress to be made in understanding impacts of livestock exposure to pharmaceuticals.
Aquatic species.
As the focus of this work is on terrestrial systems a number of key concepts are summarised briefly below and the authors refer the reader to previously published reviews that comprehensively explore the accumulation of pharmaceuticals in aquatic systems.115–117 Aquatic species can accumulate chemicals present in surface water via aqueous uptake of water-borne chemicals (bioconcentration), and dietary uptake by ingestion of contaminated food particles (biomagnification) (Fig. 1; pathway 9). Whilst experimental protocols exist to evaluate the bioconcentration of chemicals, data only exists for a select number of pharmaceuticals and in a limited number of species, primarily fish.118 Predictive approaches have been proposed to estimate bioconcentration factors (BCFs), however, with a focus on the accumulation of non-ionised pharmaceuticals by fish more work is needed to predict accumulation of contaminants of emerging concern by aquatic invertebrates and to account of the effects of ionisation on chemical uptake.119 Research has also demonstrated that for a number of pharmaceuticals, uptake by aquatic organisms can induce chronic and acute effects, however little is known about the effects of mixtures which would be considered more realistic in terms of a typical environmental exposure.120 To account for recent monitoring outputs, which have demonstrated the spatial and temporal variation of pharmaceuticals in surface waters (see earlier discussion), an evaluation of the effect of pharmaceuticals on aquatic organisms at varying scales, is needed. This adds significant complexity and uncertainty to environmental risk assessment of surface waters as well as other environmental compartments known to be reservoirs of pharmaceuticals (e.g. soil).
Priorities for parameterising the S–P–R framework
As clearly identified, there are numerous major knowledge gaps pertaining to the risks of pharmaceuticals in agricultural systems receiving reclaimed wastewater. Based on our current understanding we are therefore not at a stage where we can fully evaluate the risks of pharmaceuticals in wastewater reuse systems. Table 1 summarises briefly our current understanding and key knowledge gaps related to the sources, pathways and receptors of pharmaceuticals in agro-ecosystems. A synthesis of available data in combination with expert knowledge has enabled the components of the S–P–R diagram to be classified according to ‘Poor’, ‘Moderate’ or ‘High’ with regards to the level of understanding we have in each of these areas.
Of the 34 individual components of the S–P–R diagram we only have high level of understanding of 5 of these (Table 1). We have a greater understanding of the sources of pharmaceuticals in reclaimed wastewater and irrigation practices whereas we only have a low moderate understanding of the processes by which pharmaceuticals move between sources and are taken up by receptors. As the fate and behaviour of most pharmaceuticals entering our agriculture systems remains poorly characterized, our conception and understanding of the risks posed to receptors is equally constrained.
Future research efforts should therefore seek to address five main areas over the next 15–20 years (Fig. 3):
 |
| Fig. 3 Possible time lines and strategy for prioritised research areas, to better understand the fate, uptake and effects of wastewater derived pharmaceuticals in agro-ecosystems. | |
Analytical method development
– Develop methods for a broader suite of pharmaceuticals (and metabolites) using techniques such as high resolution mass spectrometry to enable detection in a variety of complex matrices (e.g. plant tissue) at environmentally relevant concentrations.
– Use non-target screening approaches to explore the formation of biologically active metabolites (and conjugates) to complement targeted analysis based on identified transformation pathways of pharmaceuticals known to be persistent in agro-ecosystems.
Environmental exposure
– Develop experimental datasets on the fate and reactivity of pharmaceuticals (and active metabolites and transformation products) in the environment following long-term and intensive irrigation with reclaimed wastewater.
– Account for the varying performance of different WWTP technologies in different regions and to provide a greater understanding on the use of raw or partially treated wastewater (including water used in aquaculture) for irrigation where sewage connectivity is limited or non-existent.
– Understand how future environmental change (e.g. increased temperature, drought) and agricultural developments (e.g. increased global food demand) will alter the environmental exposure of pharmaceuticals.
Uptake scenarios
– Generation of experimental data sets to evaluate the uptake of pharmaceuticals, including metabolites and transformation products identified in reclaimed wastewater of formed in soils and plants, with particular focus on previously underexplored receptors such as wildlife and the potential for multigenerational exposure (e.g. via accumulation in seeds).
– Investigate the uptake of pharmaceuticals by receptors after low-level chronic exposure and as well in response to mixture exposures.
– Determine the formation of pharmaceutical transformation products in receptors and identify metabolism pathways to help assist with predictive model development (see below).
– Identify key factors which alter the uptake, accumulation and bioaccessibility of pharmaceuticals in receptors accounting for species traits and exposure medium properties.
Uptake model development
– Parameterise and validate a holistic uptake model that accounts for the fate of chemicals in soil, interconnectivity between receptor uptake, bioaccessibility and metabolite formation for a wide suite of pharmaceuticals.
– Account for geographical and species variations in diets, food sourcing and exposure concentrations of pharmaceuticals.
Effects analysis
– Evaluate a wider suite of biological and physiological endpoints to elucidate the impact of biologically active pharmaceutical residues (including metabolites and mixtures) on soil and plant health (including soil fauna) with a view to understand implications for agricultural productivity.
– Investigate the suitability of analogous approaches to those adopted for predicting the effects of pharmaceuticals in the aquatic environment (e.g. chemical read-across).
– Develop thresholds to evaluate the human health risks from consuming produce containing pharmaceutical residues, considering chemical mixtures (i.e. likelihood of contraindications) and populations deemed most at risk (e.g. elderly, children).
Conclusions
The gap between water supply and water needs is growing; thus an integrated water resources management approach is required that utilises new sources of water for agricultural use such as reclaimed wastewater. However, we should ensure that this is done in a safe and sustainable manner and therefore the associated risks associated with such practices must be evaluated.
Use of reclaimed wastewater irrigation results in the contamination of a number of environmental compartments, each of which can act as reservoirs of pharmaceuticals. These chemicals have the potential to accumulate in a variety of receptors including terrestrial wildlife, livestock, terrestrial plants, aquatic species, soil microbial community and humans, posing a range of potential health and environmental challenges. This risk may be greatest in low to middle income countries where wastewater treatment technologies are limited or often non-existent leading to an increased use of semi treated or non-treated wastewater.
A number of broad knowledge gaps were identified, most notably that more research is needed to consider the effect of metabolites on the various pathways and receptors highlighted in Fig. 1. In addition, research pertaining to the effect and fate of pharmaceutical mixtures are lacking as well as data on bio accessibility of pharmaceuticals after ingestion by humans, wildlife and livestock. Ultimately, use of reclaimed wastewater will require a trade-off between the economic benefits and ability to meet growing populations' food demands and the environmental and human health risks associated with using reclaimed wastewater as an irrigation source. More research is needed to fully understand these risks to ensure agricultural sustainability to guide future water reuse policies.
Conflicts of interest
There are no conflicts to declare.
Acknowledgements
We would like to acknowledge the British Council who funded this project through their Institutional Links Science, Technology and Research Exchange Across Mena (STREAM) programme (project number 277947262).
References
-
World Bank, Water Global Practice High and Dry, Washington DC, 2016 Search PubMed.
-
F. Pedrero, I. Kalavrouziotis, J. J. Alarcón, P. Koukoulakis and T. Asano, Use of Treated Municipal Wastewater in Irrigated Agriculture-Review of Some Practices in Spain and Greece, Agricultural Water Management, Agric. Water Manag., 2010 Search PubMed.
-
T. Asano, F. L. Burton, H. Leverenz, R. Tsuchihashi and G. Tchobanoglous, Water Reuse: Issues, Technologies, and Applications, McGraw-Hill Professional, New York, 2007 Search PubMed.
- Y. Zhang and Y. Shen, Wastewater Irrigation: Past, Present, and Future, Wiley Interdiscip. Rev.: Water, 2017, e1234 CrossRef.
-
Y. Inbar, New Standards for Treated Wastewater Reuse in Israel, in Wastewater Reuse–Risk Assessment, Decision-Making and Environmental Security, Springer Netherlands, Dordrecht, 2007, pp. 291–296 Search PubMed.
- M. Goldstein, M. Shenker and B. Chefetz, Insights into the Uptake Processes of Wastewater-Borne Pharmaceuticals by Vegetables, Environ. Sci. Technol., 2014, 48, 5593–5600 CrossRef CAS PubMed.
- Water Authority State of Israel, Long-Term Master Plan for the National Water Sector Part A - Policy Document, Version 4, 2012.
-
P. Anderson, N. Denslow, J. E. Drewes, A. Olivieri, D. Schlenk and S. Snyder, Final Report Monitoring Strategies for Chemicals of Emerging Concern (CECs) in Recycled Water Recommendations of a Science Advisory Panel Panel Members State Water Resources Control Board, 2010 Search PubMed.
- S. Castiglioni, R. Bagnati, R. Fanelli, F. Pomati, D. Calamari and E. Zuccato, Removal of Pharmaceuticals in Sewage Treatment Plants in Italy, Environ. Sci. Technol., 2006, 40, 357–363 CrossRef CAS PubMed.
- E. Gracia-Lor, J. V. Sancho, R. Serrano and F. Hernández, Occurrence and Removal of Pharmaceuticals in Wastewater Treatment Plants at the Spanish Mediterranean Area of Valencia, Chemosphere, 2012, 87, 453–462 CrossRef CAS PubMed.
- J. Radjenovic, M. Petrovic and D. Barceló, Analysis of Pharmaceuticals in Wastewater and Removal Using a Membrane Bioreactor, Anal. Bioanal. Chem., 2007, 387, 1365–1377 CrossRef CAS PubMed.
- N. H. Tran, M. Reinhard and K. Y.-H. Gin, Occurrence and Fate of Emerging Contaminants in Municipal Wastewater Treatment Plants from Different Geographical Regions-a Review, Water Res., 2018, 133, 182–207 CrossRef CAS PubMed.
- H. A. Duong, N. H. Pham, H. T. Nguyen, T. T. Hoang, H. V. Pham, V. C. Pham, M. Berg, W. Giger and A. C. Alder, Occurrence, Fate and Antibiotic Resistance of Fluoroquinolone Antibacterials in Hospital Wastewaters in Hanoi, Vietnam, Chemosphere, 2008, 72, 968–973 CrossRef CAS PubMed.
- N. H. Tran, H. Chen, M. Reinhard, F. Mao and K. Y.-H. Gin, Occurrence and Removal of Multiple Classes of Antibiotics and Antimicrobial Agents in Biological Wastewater Treatment Processes, Water Res., 2016, 104, 461–472 CrossRef CAS PubMed.
- B. Kasprzyk-Hordern, R. M. Dinsdale and A. J. Guwy, The Removal of Pharmaceuticals, Personal Care Products, Endocrine Disruptors and Illicit Drugs during Wastewater Treatment and Its Impact on the Quality of Receiving Waters, Water Res., 2009, 43, 363–380 CrossRef CAS PubMed.
- O. Borgman and B. Chefetz, Combined Effects of Biosolids Application and Irrigation with Reclaimed Wastewater on Transport of Pharmaceutical Compounds in Arable Soils, Water Res., 2013, 47, 3431–3443 CrossRef CAS PubMed.
- A. Grossberger, Y. Hadar, T. Borch and B. Chefetz, Biodegradability of Pharmaceutical Compounds in Agricultural Soils Irrigated with Treated Wastewater, Environ. Pollut., 2014, 185, 168–177 CrossRef CAS PubMed.
- J. A. Pedersen, M. Soliman and I. H. Suffet, Human Pharmaceuticals, Hormones, and Personal Care Product Ingredients in Runoff from Agricultural Fields Irrigated with Treated Wastewater, J. Agric. Food Chem., 2005, 53(5), 1625–1632 CrossRef CAS PubMed.
-
FAO, Coping with water scarcity: an action framework for agriculture and food security, Rome, 2012 Search PubMed.
-
European Commission, Proposal for a Regulation of the European Parliament and of the Council on minimum requirements for water reuse (COM(2018) 337 final 2018/0169 (COD)), Brussels, 2018 Search PubMed.
-
L. Alcalde-Sanz and B. M. Gawlik, Minimum quality requirements for water reuse in agricultural irrigation and aquifer recharge Towards a water reuse regulatory instrument at EU level, Luxembourg Publications Office of the European Union, 2017, pp. 1–69, DOI:10.2760/887727.
-
WHO, Guidelines for the safe use of wastewater, excreta, and greywater, World Health Organization, Geneva, Switzerland, vol. 2, 2006 Search PubMed.
-
NRMMC-EPHC-AHMC, Australian guidelines for water recycling: managing health and environmental risks: Phase 1, Canberra, Australia, 2006 Search PubMed.
-
MRMMC-EPHC-AHMC, Australian guidelines for water recycling: managing health and environmental risks (Phase 2) Augmentation of Drinking Water Supplies, Canberra, Australia, 2008 Search PubMed.
- W. L. Song, Y. J. Ding, C. T. Chiou and H. Li, Selected Veterinary Pharmaceuticals in Agricultural Water and Soil from Land Application of Animal Manure, J. Environ. Qual., 2010, 39, 1211–1217 CrossRef CAS PubMed.
- C. A. Kinney, E. T. Furlong, S. D. Zaugg, M. R. Burkhardt, S. L. Werner, J. D. Cahill and G. R. Jorgensen, Survey of Organic Wastewater Contaminants in Biosolids Destined for Land Application, Environ. Sci. Technol., 2006, 40, 7207–7215 CrossRef CAS PubMed.
-
AQUASTAT, AQUASTAT - FAO's Information System on Water and Agriculture: Municipal Wastewater Database, accessed 29 November 2018, http://www.fao.org/nr/water/aquastat/wastewater/index.stm#db Search PubMed.
-
C. A. Scott, N. I. Faruqui and L. Raschid-Sally, Wastewater Use in Irrigated Agriculture: Management Challenges in Developing Countries, in Wastewater use in irrigated agriculture: confronting the livelihood and environmental realities, CABI, Wallingford, 2004, pp. 1–10 Search PubMed.
- A. Qishlaqi, F. Moore and G. Forghani, Impact of Untreated Wastewater Irrigation on Soils and Crops in Shiraz Suburban Area, SW Iran, Environ. Monit. Assess., 2008, 141, 257–273 CrossRef CAS PubMed.
- P. Verlicchi, M. Al Aukidy and E. Zambello, Occurrence of Pharmaceutical Compounds in Urban Wastewater: Removal, Mass Load and Environmental Risk after a Secondary Treatment—A Review, Sci. Total Environ., 2012, 429, 123–155 CrossRef CAS PubMed.
- E. Gracia-Lor, J. V. Sancho, R. Serrano and F. Hernández, Occurrence and Removal of Pharmaceuticals in Wastewater Treatment Plants at the Spanish Mediterranean Area of Valencia, Chemosphere, 2012, 87, 453–462 CrossRef CAS PubMed.
-
R. Loos, R. Carvalho, S. Comero, D. C. António, M. Ghiani, T. Lettieri, G. Locoro, B. Paracchini, S. Tavazzi, B. M. Gawlik, S. Voorspoels, D. Schwesig and R. Loos, EU Wide Monitoring Survey on Waste Water Treatment Plant Effluents, Publications Office of the European Union, Luxembourg, 2012 Search PubMed.
-
T. aus der Beek, F.-A. Weber, A. Bergmann, G. Gruttner and A. Carius, Pharmaceuticals in the environment: Global occurrence and potential cooperative action under the Strategic Approach to International Chemicals Management, SAICM, 2016 Search PubMed.
- J. H. Guo, C. J. Sinclair, K. Selby and A. B. A. Boxall, Toxicological and Ecotoxicological Risk-Based Prioritization of Pharmaceuticals in the Natural Environment, Environ. Toxicol. Chem., 2016, 35, 1550–1559 CrossRef CAS PubMed.
-
Umwelt Bundesamt (UBA), Database – Pharmaceuticals in the environment, Umweltbundesamt, accessed 29 November 2018, https://www.umweltbundesamt.de/en/database-pharmaceuticals-in-the-environment-0 Search PubMed.
- E. Archer, B. Petrie, B. Kasprzyk-Hordern and G. M. Wolfaardt, The Fate of Pharmaceuticals and Personal Care Products (PPCPs), Endocrine Disrupting Contaminants (EDCs), Metabolites and Illicit Drugs in a WWTW and Environmental Waters, Chemosphere, 2017, 174, 437–446 CrossRef CAS PubMed.
- R. Gurke, M. Rossler, C. Marx, S. Diamond, S. Schubert, R. Oertel and J. Fauler, Occurrence and Removal of Frequently Prescribed Pharmaceuticals and Corresponding Metabolites in Wastewater of a Sewage Treatment Plant, Sci. Total Environ., 2015, 532, 762–770 CrossRef CAS PubMed.
-
J. Struijs, SimpleTreat 4.0, a Model to Predict Fate and Emission of Chemicals in Wastewater Treatment Plants, RIVM (National Institute for Public Health and the Environment), Bilthoven, The Netherlands, 2014 Search PubMed.
- L. S. Lautz, J. Struijs, T. M. Nolte, A. M. Breure, E. van der Grinten, D. van de Meent and R. van Zelm, Evaluation of SimpleTreat 4.0: Simulations of Pharmaceutical Removal in Wastewater Treatment Plant Facilities, Chemosphere, 2017, 168, 870–876 CrossRef CAS PubMed.
- S. T. J. Droge and K.-U. Goss, Development and Evaluation of a New Sorption Model for Organic Cations in Soil: Contributions from Organic Matter and Clay Minerals, Environ. Sci. Technol., 2013, 47, 14233–14241 CrossRef CAS PubMed.
- A. Franco, W. Fu and S. Trapp, Influence of soil ph on the sorption of ionizable chemicals: modeling advances, Environ. Toxicol. Chem., 2009, 28, 458–464 CrossRef CAS PubMed.
- D. E. Helbling, J. Hollender, H.-P. E. Kohler, H. Singer and K. Fenner, Throughput Identification of Microbial Transformation Products of Organic Micropollutants, Environ. Sci. Technol., 2010, 44, 6621–6627 CrossRef CAS PubMed.
- G. E. Cordy, N. L. Duran, H. Bouwer, R. C. Rice, E. T. Furlong, S. D. Zaugg, M. T. Meyer, L. B. Barber and D. W. Kolpin, Do Pharmaceuticals, Pathogens, and Other Organic Waste Water Compounds Persist When Waste Water Is Used for Recharge?, Groundwater Monit. Rem., 2004, 24, 58–69 CrossRef CAS.
- J. E. Drewes, T. Heberer and K. Reddersen, Fate of Pharmaceuticals during Indirect Potable Reuse, Water Sci. Technol., 2002, 46, 73–80 CrossRef CAS PubMed.
- J. Qian, H. Horn, J. Tarchitzky, Y. Chen, S. Katz and M. Wagner, Water Quality and Daily Temperature Cycle Affect Biofilm Formation in Drip Irrigation Devices Revealed by Optical Coherence Tomography, Biofouling, 2017, 33, 211–221 CrossRef CAS PubMed.
- M. Biel-Maeso, C. Corada-Fernández and P. A. Lara-Martín, Monitoring the Occurrence of Pharmaceuticals in Soils Irrigated with Reclaimed Wastewater, Environ. Pollut., 2018, 235, 312–321 CrossRef CAS PubMed.
- P. Dalkmann, M. Broszat, C. Siebe, E. Willaschek, T. Sakinc, J. Huebner, W. Amelung, E. Grohmann and J. Siemens, Accumulation of Pharmaceuticals, Enterococcus, and Resistance Genes in Soils Irrigated with Wastewater for Zero to 100 Years in Central Mexico, PLoS One, 2012, 7, 10 CrossRef.
- C. A. Kinney, E. T. Furlong, S. L. Werner and J. D. Cahill, Presence and Distribution of Wastewater-Derived Pharmaceuticals in Soil Irrigated with Reclaimed Water, Environ. Toxicol. Chem., 2006, 25, 317–326 CrossRef CAS PubMed.
- A. Białk-Bielińska, J. Kumirska, M. Borecka, M. Caban, M. Paszkiewicz, K. Pazdro and P. Stepnowski, Selected Analytical Challenges in the Determination of Pharmaceuticals in Drinking/Marine Waters and Soil/Sediment Samples, J. Pharm. Biomed. Anal., 2016, 121, 271–296 CrossRef PubMed.
- X. García-Santiago, J. M. Garrido, J. M. Lema and A. Franco-Uría, A. Fate of Pharmaceuticals in Soil after Application of STPs Products: Influence of Physicochemical Properties and Modelling Approach, Chemosphere, 2017, 182, 406–415 CrossRef PubMed.
- R. Kodešová, R. Grabic, M. Kočárek, A. Klement, O. Golovko, M. Fér, A. Nikodem and O. Jakšík, Pharmaceuticals' Sorptions Relative to Properties of Thirteen Different Soils, Sci. Total Environ., 2015, 511, 435–443 CrossRef PubMed.
- S. C. Monteiro and A. B. A. Boxall, Factors affecting the degradation of pharmaceuticals in agricultural soils, Environ. Toxicol. Chem., 2009, 28, 2546–2554 CrossRef CAS PubMed.
- S. Bondarenko, J. Gan, F. Ernst, R. Green, J. Baird and M. McCullough, Leaching of Pharmaceuticals and Personal Care Products in Turfgrass Soils during Recycled Water Irrigation, J. Environ. Qual., 2012, 41, 1268–1274 CrossRef CAS PubMed.
- L. K. Dodgen and W. Zheng, Effects of Reclaimed Water Matrix on Fate of Pharmaceuticals and Personal Care Products in Soil, Chemosphere, 2016, 156, 286–293 CrossRef CAS PubMed.
- Y. Wu, M. Williams, L. Smith, D. Chen and R. Kookana, Dissipation of Sulfamethoxazole and Trimethoprim Antibiotics from Manure-Amended Soils, J. Environ. Sci. Health, Part B, 2012, 47, 240–249 CrossRef CAS PubMed.
-
N. H. Batjes, A Global Data Set of Soil pH Properties, Technical Paper 27, 1995 Search PubMed.
- S. T. J. Droge and K. Goss, Sorption of Organic Cations to Phyllosilicate Clay Minerals: CEC- Normalization, Salt Dependency, and the Role of Electrostatic and Hydrophobic Effects, Environ. Sci. Technol., 2013, 47, 14224–14232 CrossRef CAS PubMed.
- S. T. J. Droge and K. U. Goss, Ion-Exchange Affinity of Organic Cations to Natural Organic Matter: Influence of Amine Type and Nonionic Interactions at Two Different PHs, Environ. Sci. Technol., 2013, 47, 798–806 CrossRef CAS PubMed.
- A. J. Ebele, M. Abou-Elwafa Abdallah and S. Harrad, Pharmaceuticals and Personal Care Products (PPCPs) in the Freshwater Aquatic Environment, Emerging Contaminants, 2017, 3, 1–16 CrossRef.
- A. Nikolaou, S. Meric and D. Fatta, Occurrence Patterns of Pharmaceuticals in Water and Wastewater Environments, Anal. Bioanal. Chem., 2007, 387, 1225–1234 CrossRef CAS PubMed.
- E. Topp, S. C. Monteiro, A. Beck, B. B. Coelho, A. B. A. Boxall, P. W. Duenk, S. Kleywegt, D. R. Lapen, M. Payne, L. Sabourin, H. Li and C. D. Metcalfe, Runoff of Pharmaceuticals and Personal Care Products Following Application of Biosolids to an Agricultural Field, Sci. Total Environ., 2008, 396, 52–59 CrossRef CAS PubMed.
- R. Gibson, J. C. Durán-Álvarez, K. L. Estrada, A. Chávez and B. Jiménez Cisneros, Accumulation and Leaching Potential of Some Pharmaceuticals and Potential Endocrine Disruptors in Soils Irrigated with Wastewater in the Tula Valley, Mexico, Chemosphere, 2010, 81, 1437–1445 CrossRef CAS PubMed.
- F. A. Kibuye, H. E. Gall, K. R. Elkin, B. Ayers, T. L. Veith, M. Miller, S. Jacob, K. R. Hayden, J. E. Watson and H. A. Elliott, Fate of Pharmaceuticals in a Spray-Irrigation System: From Wastewater to Groundwater, Sci. Total Environ., 2019, 654, 197–208 CrossRef CAS PubMed.
- J. Oppel, G. Broll, D. Loffler, M. Meller, J. Rombke and T. Ternes, Leaching Behaviour of Pharmaceuticals in Soil-Testing-Systems: A Part of an Environmental Risk Assessment for Groundwater Protection, Sci. Total Environ., 2004, 328, 265–273 CrossRef CAS PubMed.
- P. A. Blackwell, P. Kay, R. Ashauer and A. B. A. Boxall, Effects of Agricultural Conditions on the Leaching Behaviour of Veterinary Antibiotics in Soils, Chemosphere, 2009, 75, 13–19 CrossRef CAS PubMed.
- D. J. Lapworth, N. Baran, M. E. Stuart and R. S. Ward, Emerging Organic Contaminants in Groundwater: A Review of Sources, Fate and Occurrence, Environ. Pollut., 2012, 163, 287–303 CrossRef CAS PubMed.
- T. Heberer, A. Mechlinski, B. Fanck, A. Knappe, G. Massmann, A. Pekdeger and B. Fritz, Field Studies on the Fate and Transport of Pharmaceutical Residues in Bank Filtration, Groundwater Monit. Rem., 2004, 24, 70–77 CrossRef CAS.
- D. Kumar, M. K. M. Chaturvedi, S. K. Sharma and S. R. Asolekar, Sewage-Fed Aquaculture: A Sustainable Approach for Wastewater Treatment and Reuse, Environ. Monit. Assess., 2015, 187, 656 CrossRef PubMed.
- K. E. Arnold, A. R. Brown, G. T. Ankley and J. P. Sumpter, Medicating the Environment: Assessing Risks of Pharmaceuticals to Wildlife and Ecosystems, Philos. Trans. R. Soc., B, 2014, 369(1656), 20130569 CrossRef PubMed.
- L. J. Carter, E. Harris, M. Williams, J. J. Ryan, R. S. Kookana and A. B. A. Boxall, Fate and Uptake of Pharmaceuticals in Soil-Plant Systems, J. Agric. Food Chem., 2014, 62, 816–825 CrossRef CAS PubMed.
- R. Tanoue, Y. Sato, M. Motoyama, S. Nakagawa, R. Shinohara and K. Nomiyama, Plant Uptake of Pharmaceutical Chemicals Detected in Recycled
Organic Manure and Reclaimed Wastewater, J. Agric. Food Chem., 2012, 60, 10203–10211 CrossRef CAS PubMed.
- A. M. Franklin, C. F. Williams, D. M. Andrews, E. E. Woodward and J. E. Watson, Uptake of Three Antibiotics and an Antiepileptic Drug by Wheat Crops Spray Irrigated with Wastewater Treatment Plant Effluent, J. Environ. Qual., 2016, 45(2), 546–554 CrossRef CAS PubMed.
- T. Malchi, Y. Maor, G. Tadmor, M. Shenker and B. Chefetz, Irrigation of Root Vegetables with Treated Wastewater: Evaluating Uptake of Pharmaceuticals and the Associated Human Health Risks, Environ. Sci. Technol., 2014, 48, 9325–9333 CrossRef CAS PubMed.
- X. Q. Wu, J. L. Conkle, F. Ernst and J. Gan, Treated Wastewater Irrigation: Uptake of Pharmaceutical and Personal Care Products by Common Vegetables under Field Conditions, Environ. Sci. Technol., 2014, 48, 11286–11293 CrossRef CAS PubMed.
- E. Ben Mordechay, J. Tarchitzky, Y. Chen, M. Shenker and B. Chefetz, Composted Biosolids and Treated Wastewater as Sources of Pharmaceuticals and Personal Care Products for Plant Uptake: A Case Study with Carbamazepine, Environ. Pollut., 2018, 232, 164–172 CrossRef CAS PubMed.
- G. D. Bhalsod, Y.-H. Chuang, S. Jeon, W. Gui, H. Li, E. T. Ryser, A. K. Guber and W. Zhang, Uptake and Accumulation of Pharmaceuticals in Overhead- and Surface-Irrigated Greenhouse Lettuce, J. Agric. Food Chem., 2018, 66, 822–830 CrossRef CAS PubMed.
- M. D. Celiz, J. Tso and D. S. Aga, Pharmaceutical Metabolites in the Environment: Analytical Challenges and Ecological Risks, Environ. Toxicol. Chem., 2009, 28, 2473–2484 CrossRef CAS PubMed.
- L. K. Dodgen, J. Li, X. Wu, Z. Lu and J. J. Gan, Transformation and Removal Pathways of Four Common PPCP/EDCs in Soil, Environ. Pollut., 2014, 193, 29–36 CrossRef CAS PubMed.
- B. Bartha, C. Huber and P. Schroder, Uptake and Metabolism of Diclofenac in Typha Latifolia--How Plants Cope with Human Pharmaceutical Pollution, Plant Sci., 2014, 227, 12–20 CrossRef CAS PubMed.
- L. J. Carter, M. Williams, S. Martin, S. P. B. Kamaludeen and R. S. Kookana, Sorption, Plant Uptake and Metabolism of Benzodiazepines, Sci. Total Environ., 2018, 628–629, 18–25 CrossRef CAS PubMed.
- C. Riemenschneider, B. Seiwert, M. Moeder, D. Schwarz and T. Reemtsma, Extensive Transformation of the Pharmaceutical Carbamazepine Following Uptake into Intact Tomato Plants, Environ. Sci. Technol., 2017, 51, 6100–6109 CrossRef CAS PubMed.
- M. Goldstein, T. Malchi, M. Shenker and B. Chefetz, Pharmacokinetics in Plants: Carbamazepine and Its Interactions with Lamotrigine, Environ. Sci. Technol., 2018, 52, 6957–6964 CrossRef CAS PubMed.
- X. S. Miao, J. J. Yang and C. D. Metcalfe, Carbamazepine and Its Metabolites in Wastewater and in Biosolids in a Municipal Wastewater Treatment Plant, Environ. Sci. Technol., 2005, 39, 7469–7475 CrossRef CAS PubMed.
- N. M. Satchivi, E. W. Stoller, L. M. Wax and D. P. Briskin, Simulation Model for Xenobiotic Transport and Whole Plant Allocation Following Foliar Application. I. Conceptual Foundation for Model Development, Pestic. Biochem. Physiol., 2000, 68, 67–84 CrossRef CAS.
- S. Trapp and M. Matthies, Generic One-Compartment Model for Uptake of Organic Chemicals by Foliar Vegetation, Environ. Sci. Technol., 1995, 29, 2333–2338 CrossRef CAS PubMed.
- N. M. Satchivi, E. W. Stoller, L. M. Wax and D. P. Briskin, Nonlinear Dynamic Simulation Model for Xenobiotic Transport and Whole Plant Allocation Following Foliar Application. II. Model Validation, Pestic. Biochem. Physiol., 2000, 68, 85–95 CrossRef CAS.
- G. G. Briggs, R. H. Bromilow and A. A. Evans, Relationships between Lipophilicity and Root Uptake and Translocation of Non-Ionized Chemicals by Barley, Pestic. Sci., 1982, 13, 495–504 CrossRef CAS.
- E. L. Miller, S. L. Nason, K. G. Karthikeyan and J. A. Pedersen, Root Uptake of Pharmaceuticals and Personal Care Product Ingredients, Environ. Sci. Technol., 2016, 50, 525–541 CrossRef CAS PubMed.
- D. G. Hillis, J. Fletcher, K. R. Solomon and P. K. Sibley, Effects of Ten Antibiotics on Seed Germination and Root Elongation in Three Plant Species, Arch. Environ. Contam. Toxicol., 2011, 60, 220–232 CrossRef CAS PubMed.
- F. Liu, G. G. Ying, R. Tao, Z. Jian-Liang, J. F. Yang and L. F. Zhao, Selected Antibiotics on Plant Growth and Soil Microbial and Enzymatic Activities, Environ. Pollut., 2009, 157, 1636–1642 CrossRef CAS PubMed.
- J. B. Sallach, S. L. Bartelt-Hunt, D. D. Snow, X. Li and L. Hodges, Uptake of Antibiotics and Their Toxicity to Lettuce Following Routine Irrigation with Contaminated Water in Different Soil Types, Environ. Eng. Sci., 2018, 35(8), 887–896 CrossRef CAS.
- A. Christou, C. Antoniou, C. Christodoulou, E. Hapeshi, I. Stavrou, C. Michael, D. Fatta-Kassinos and V. Fotopoulos, Detoxification Mechanisms Induced by Common Pharmaceuticals in Alfalfa (Medicago Sativa L.), Sci. Total Environ., 2016, 557–558, 652–664 CrossRef CAS PubMed.
- C. Sun, S. Dudley, J. Trumble and J. Gan, Pharmaceutical and Personal Care Products-Induced Stress Symptoms and Detoxification Mechanisms in Cucumber Plants, Environ. Pollut., 2018, 234, 39–47 CrossRef CAS PubMed.
- C. Hurtado, Y. N. Montano-Chávez, C. Domínguez and J. M. Bayona, Degradation of Emerging Organic Contaminants in an Agricultural Soil: Decoupling Biotic and Abiotic Processes, Water, Air, Soil Pollut., 2017, 228, 243 CrossRef.
- L. J. Carter, M. Williams, C. Böttcher and R. S. Kookana, Uptake of Pharmaceuticals Influences Plant Development and Affects Nutrient and Hormone Homeostases, Environ. Sci. Technol., 2015, 49, 12509–12518 CrossRef CAS PubMed.
- B. Verbruggen, L. Gunnarsson, E. Kristiansson, T. Österlund, S. F. Owen, J. R. Snape and C. R. Tyler, A Database Connecting Drugs and Conservation of Their Targets across Species, Nucleic Acids Res., 2018, 46, D930–D936 CrossRef CAS PubMed.
- R. A. Brain, M. L. Hanson, K. R. Solomon and B. W. Brooks, Aquatic Plants Exposed to Pharmaceuticals: Effects and Risks, Rev. Environ. Contam. Toxicol., 2008, 192, 67–115 CrossRef CAS.
- G. J. Gielen, P. W. Clinton, M. R. van den Heuvel, M. O. Kimberley and L. G. Greenfield, Influence of Sewage and Pharmaceuticals on Soil Microbial Function, Environ. Toxicol. Chem., 2011, 30, 1086–1095 CrossRef CAS PubMed.
- A. M. Ibekwe, A. Gonzalez-Rubio and D. L. Suarez, Impact of Treated Wastewater for Irrigation on Soil Microbial Communities, Sci. Total Environ., 2018, 622–623, 1603–1610 CrossRef CAS PubMed.
- S. Thiele-Bruhn and I.-C. Beck, Effects of Sulfonamide and Tetracycline Antibiotics on Soil Microbial Activity and Microbial Biomass, Chemosphere, 2005, 59, 457–465 CrossRef CAS PubMed.
- F. Liu, J. Wu, G.-G. Ying, Z. Luo and H. Feng, Changes in Functional Diversity of Soil Microbial Community with Addition of Antibiotics Sulfamethoxazole and Chlortetracycline, Appl. Microbiol. Biotechnol., 2012, 95, 1615–1623 CrossRef CAS PubMed.
- J. Williams-Nguyen, J. B. Sallach, S. Bartelt-Hunt, A. B. Boxall, L. M. Durso, J. E. McLain, R. S. Singer, D. D. Snow and J. L. Zilles, Antibiotics and Antibiotic Resistance in Agroecosystems: State of the Science, J. Environ. Qual., 2016, 45, 394–406 CrossRef CAS PubMed.
- O. Paltiel, G. Fedorova, G. Tadmor, G. Kleinstern, Y. Maor and B. Chefetz, Human Exposure to Wastewater-Derived Pharmaceuticals in Fresh Produce: A Randomized Controlled Trial Focusing on Carbamazepine, Environ. Sci. Technol., 2016, 50(8), 4476–4482 CrossRef CAS PubMed.
- X. Q. Wu, F. Ernst, J. L. Conkle and J. Gan, Comparative Uptake and Translocation of Pharmaceutical and Personal Care Products (PPCPs) by Common Vegetables, Environ. Int., 2013, 60, 15–22 CrossRef CAS PubMed.
- L. K. Dodgen, J. Li, D. Parker and J. J. Gan, Uptake and Accumulation of Four PPCP/EDCs in Two Leafy Vegetables, Environ. Pollut., 2013, 182, 150–156 CrossRef CAS PubMed.
- C. Riemenschneider, M. Al-Raggad, M. Moeder, B. Seiwert, E. Salameh and T. Reemtsma, Pharmaceuticals, Their Metabolites, and Other Polar Pollutants in Field-Grown Vegetables Irrigated with Treated Municipal Wastewater, J. Agric. Food Chem., 2016, 64, 5784–5792 CrossRef CAS PubMed.
- L. J. Carter, C. D. Garman, J. Ryan, A. Dowle, E. Bergstrom, J. Thomas-Oates and A. B. A. Boxall, Fate and Uptake of Pharmaceuticals in Soil-Earthworm Systems, Environ. Sci. Technol., 2014, 48, 5955–5963 CrossRef CAS PubMed.
- C. A. Kinney, E. T. Furlong, D. W. Kolpin, M. R. Burkhardt, S. D. Zaugg, S. L. Werner, J. P. Bossio and M. J. Benotti, Bioaccumulation of Pharmaceuticals and Other Anthropogenic Waste Indicators in Earthworms from Agricultural Soil Amended with Biosolid or Swine Manure, Environ. Sci. Technol., 2008, 42, 1863–1870 CrossRef CAS.
-
European Commission, Technical Guidance Document on Risk Assessment Part II, Institute for Health and Consumer Protection - European Chemicals Bureau, Luxembourg, 2003, vol. EUR 20418 Search PubMed.
- T. G. Bean, K. E. Arnold, J. Lane, S. Pietravalle and A. B. A. Boxall, Method for Determining the Bioaccessibility of Pharmaceuticals in Wildlife, Environ. Toxicol. Chem., 2016, 35, 2349–2357 CrossRef CAS PubMed.
- S. Markman, S. Leitner, C. Catchpole, S. Barnsley, C. T. Muller, D. Pascoe and K. L. Buchanan, Pollutants Increase Song Complexity and the Volume of the Brain Area HVC in a Songbird, PLoS One, 2008, 3(2), e1674 CrossRef PubMed.
- R. F. Shore, M. A. Taggart, J. Smits, R. Mateo, N. L. Richards and S. Fryday, Detection and Drivers of Exposure and Effects of Pharmaceuticals in Higher Vertebrates, Philos. Trans. R. Soc., B, 2014, 369, 20130570 CrossRef PubMed.
- J. L. Oaks, M. Gilbert, M. Z. Virani, R. T. Watson, C. U. Meteyer, B. A. Rideout, H. L. Shivaprasad, S. Ahmed, M. J. I. Chaudhry, M. Arshad, S. Mahmood, A. Ali and A. A. Khan, Diclofenac Residues as the Cause of Vulture Population Decline in Pakistan, Nature, 2004, 427, 630–633 CrossRef CAS PubMed.
- G. O. Thomas, A. J. Sweetman and K. C. Jones, Polychlorinated Biphenyls in a Long-Term Study of Lactating Dairy Cows, Environ. Sci. Technol., 1999, 33, 104–112 CrossRef CAS.
- T. H. Miller, N. R. Bury, S. F. Owen, J. I. MacRae and L. P. Barron, A Review of the Pharmaceutical Exposome in Aquatic Fauna, Environ. Pollut., 2018, 239, 129–146 CrossRef CAS PubMed.
- J. Corcoran, M. J. Winter and C. R. Tyler, Pharmaceuticals in the Aquatic Environment: A Critical Review of the Evidence for Health Effects in Fish, Crit. Rev. Toxicol., 2010, 40, 287–304 CrossRef CAS PubMed.
- O. A. H. Jones, N. Voulvoulis and J. N. Lester, Human Pharmaceuticals in the Aquatic Environment a Review, Environ. Technol., 2001, 22, 1383–1394 CrossRef CAS PubMed.
- A. Zenker, M. R. Cicero, F. Prestinaci, P. Bottoni and M. Carere, Bioaccumulation and Biomagnification Potential of Pharmaceuticals with a Focus to the Aquatic Environment, J. Environ. Manage., 2014, 133, 378–387 CrossRef CAS PubMed.
- A. B. A. Boxall, M. A. Rudd, B. W. Brooks, D. J. Caldwell, K. Choi, S. Hickmann, E. Innes, K. Ostapyk, J. P. Staveley, T. Verslycke, G. T. Ankley, K. F. Beazley, S. E. Belanger, J. P. Berninger, P. Carriquiriborde, A. Coors, P. C. DeLeo, S. D. Dyer, J. F. Ericson, F. Gagne, J. P. Giesy, T. Gouin, L. Hallstrom, M. V. Karlsson, D. G. J. Larsson, J. M. Lazorchak, F. Mastrocco, A. McLaughlin, M. E. McMaster, R. D. Meyerhoff, R. Moore, J. L. Parrott, J. R. Snape, R. Murray-Smith, M. R. Servos, P. K. Sibley, J. O. Straub, N. D. Szabo, E. Topp, G. R. Tetreault, V. L. Trudeau and G. Van Der Kraak, Pharmaceuticals and Personal Care Products in the Environment: What Are the Big Questions?, Environ. Health Perspect., 2012, 120, 1221–1229 CrossRef PubMed.
- R. P. Schwarzenbach, B. I. Escher, K. Fenner, T. B. Hofstetter, C. A. Johnson, U. von Gunten and B. Wehrli, The Challenge of Micropollutants in Aquatic Systems, Science, 2006, 313, 1072–1077 CrossRef CAS PubMed.
-
FAO, Water withdrawal by sector, around 2007, AQUASTAT database, 2014 Search PubMed.
-
OECD, Test No. 106: Adsorption – Desorption Using a Batch Equilibrium Method, OECD Publishing, 2000 Search PubMed.
- J. Simunek, M. T. van Genuchten and M. Sejna, HYDRUS: Model Use, Calibration, and Validation, Trans. ASABE, 2012, 55, 1263–1276 Search PubMed.
- W. A. Jury and H. Flühler, Transport of Chemicals Through Soil: Mechanisms, Models, and Field Applications, Adv. Agron., 1992, 47, 141–201 CAS.
- L. M. Abriola, Modeling Multiphase Migration of Organic Chemicals in Groundwater Systems, Environ. Health Perspect., 1989, 83, 117 CAS.
- P. Panagos, M. Van Liedekerke, A. Jones and L. Montanarella, European Soil Data Centre: Response to European Policy Support and Public Data Requirements, Land Use Policy, 2012, 29, 329–338 CrossRef.
-
USGS, USGS (United States Geological Survey), Groundwater Watch, accessed 11 March 2019, https://groundwaterwatch.usgs.gov/ Search PubMed.
- M. S. Fram and K. Belitz, Occurrence and Concentrations of Pharmaceutical Compounds in Groundwater Used for Public Drinking-Water Supply in California, Sci. Total Environ., 2011, 409, 3409–3417 CrossRef CAS PubMed.
- G. K. Devia, B. P. Ganasri and G. S. Dwarakish, A Review on Hydrological Models, Aquat. Procedia, 2015, 4, 1001–1007 CrossRef.
- J. A. Pedersen, M. A. Yeager and I. H. Suffet, Xenobiotic Organic Compounds in Runoff from Fields Irrigated with Treated Wastewater, J. Agric. Food Chem., 2003, 51, 1360–1372 CrossRef CAS PubMed.
- R. Wallach and R. Shabtai, Modelling Surface Runoff Contamination by Soil Chemicals under Transient Water Infiltration, J. Hydrol., 1992, 132, 263–281 CrossRef CAS.
- P. I. Adriaanse, R. C. Van Leerdam and J. J. T. I. Boesten, The Effect of the Runoff Size on the Pesticide Concentration in Runoff Water and in FOCUS Streams Simulated by PRZM and TOXSWA, Sci. Total Environ., 2017, 584–585, 268–281 CrossRef CAS PubMed.
- M. L. Richardson and J. M. Bowron, The Fate of Pharmaceutical Chemicals in the Aquatic Environment, J. Pharm. Pharmacol., 1985, 37, 1–12 CrossRef CAS PubMed.
- A. L. Spongberg and J. D. Witter, Pharmaceutical Compounds in the Wastewater Process Stream in Northwest Ohio, Sci. Total Environ., 2008, 397, 148–157 CrossRef CAS PubMed.
-
European Environment Angency, Water use and environmental pressures, accessed 9 March 2019, https://www.eea.europa.eu/themes/water/european-waters/water-use-and-environmental-pressures/water-use-and-environmental-pressures Search PubMed.
- D. Molden, T. Oweis, P. Steduto, P. Bindraban, M. A. Hanjra and J. Kijne, Improving Agricultural Water Productivity: Between Optimism and Caution, Agric. Water Manag., 2010, 97(4), 528–535 CrossRef.
- A. Kotzerke, S. Sharma, K. Schauss, H. Heuer, S. Thiele-Bruhn, K. Smalla, B.-M. Wilke and M. Schloter, Alterations in Soil Microbial Activity and N-Transformation Processes Due to Sulfadiazine Loads
in Pig-Manure, Environ. Pollut., 2008, 153, 315–322 CrossRef CAS PubMed.
- C. Girardi, J. Greve, M. Lamshöft, I. Fetzer, A. Miltner, A. Schäffer and M. Kästner, Biodegradation of Ciprofloxacin in Water and Soil and Its Effects on the Microbial Communities, J. Hazard. Mater., 2011, 198, 22–30 CrossRef CAS PubMed.
- C. Ding and J. He, Antibiotics in the Environment on Microbial Populations, Appl. Microbiol. Biotechnol., 2010, 87, 925–941 CrossRef CAS PubMed.
- M. V. Karlsson, L. J. Carter, A. Agatz and A. B. A. Boxall, Novel Approach for Characterizing PH-Dependent Uptake of Ionizable Chemicals in Aquatic Organisms, Environ. Sci. Technol., 2017, 51, 6965–6971 CrossRef CAS PubMed.
- J. A. Arnot and F. A. P. C. Gobas, A Generic QSAR for Assessing the Bioaccumulation Potential of Organic Chemicals in Aquatic Food Webs, QSAR Comb. Sci., 2003, 22, 337–345 CrossRef CAS.
- J. M. Armitage, J. A. Arnot, F. Wania and D. Mackay, Development and Evaluation of a Mechanistic Bioconcentration Model for Ionogenic Organic Chemicals in Fish, Environ. Toxicol. Chem., 2013, 32, 115–128 CrossRef CAS PubMed.
-
OECD, Test No. 305: Bioaccumulation in Fish: Aqueous and Dietary Exposure, OECD, 2012 Search PubMed.
- M. D. Overturf, J. C. Anderson, Z. Pandelides, L. Beyger and D. A. Holdway, Pharmaceuticals and Personal Care Products: A Critical Review of the Impacts on Fish Reproduction, Crit. Rev. Toxicol., 2015, 45, 469–491 CrossRef CAS PubMed.
- T. Brodin, S. Piovano, J. Fick, J. Klaminder, M. Heynen and M. Jonsson, Ecological Effects of Pharmaceuticals in Aquatic Systems–Impacts through Behavioural Alterations, Philos. Trans. R. Soc., B, 2014, 369(1656), 1–10 CrossRef PubMed.
- J. P. Berninger and B. W. Brooks, Leveraging Mammalian Pharmaceutical Toxicology and Pharmacology Data to Predict Chronic Fish Responses to Pharmaceuticals, Toxicol. Lett., 2010, 193, 69–78 CrossRef CAS PubMed.
- F. Melnikov, J. Kostal, A. Voutchkova-Kostal, J. B. Zimmerman and P. T. Anastas, Assessment of Predictive Models for Estimating the Acute Aquatic Toxicity of Organic Chemicals, Green Chem., 2016, 18, 4432–4445 RSC.
- B. I. Escher, N. Bramaz, R. I. L. Eggen and M. Richter, In Vitro Assessment of Modes of Toxic Action of Pharmaceuticals in Aquatic Life, Environ. Sci. Technol., 2005, 39, 3090–3100 CrossRef CAS.
- R. S. Prosser, S. Trapp and P. K. Sibley, Modeling Uptake of Selected Pharmaceuticals and Personal Care Products into Food Crops from Biosolids-Amended Soil, Environ. Sci. Technol., 2014, 48, 11397–11404 CrossRef CAS PubMed.
- S. Trapp, Plant Uptake and Transport Models for Neutral and Ionic Chemicals, Environ. Sci. Pollut. Res., 2004, 11, 33–39 CrossRef CAS PubMed.
- M. Lamshoeft, Z. Gao, H. Resseler, C. Schriever, R. Sur, P. Sweeney, S. Webb, B. Zillgens and M. U. Reitz, Evaluation of a Novel Test Design to Determine Uptake of Chemicals by Plant Roots, Sci. Total Environ., 2018, 613–614, 10–19 CrossRef CAS PubMed.
-
DWI, Drinking Water Safety, London, 2009 Search PubMed.
-
E. and R. A. Department of Agriculture, Water advice for livestock farmers, accessed 11 March 2019, https://www.daera-ni.gov.uk/articles/water-advice-livestock-farmers Search PubMed.
- M. Schulz and A. Schmoldt, Therapeutic and Toxic Blood Concentrations of More than 800 Drugs and Other Xenobiotics, Pharmazie, 2003, 58, 447–474 CAS.
- B. Meibohm and H. Derendorf, Basic Concepts of Pharmacokinetic/Pharmacodynamic (PK/PD) Modelling, Int. J. Clin. Pharmacol. Ther., 1997, 35, 401–413 CAS.
-
P. Lee, H. Aizawa, L. Gan, C. Prakash and D. Zhong, Handbook of metabolic pathways of xenobiotics, John Wiley & Sons, 1st edn, 2014 Search PubMed.
- R. J. L. Heron and F. C. Pickering, Health Effects of Exposure to Active Pharmaceutical Ingredients (APIs), Occup. Med., 2003, 53, 357–362 CrossRef CAS PubMed.
- T. G. Bean, K. E. Arnold, J. M. Lane, E. Bergström, J. Thomas-Oates, B. A. Rattner and A. B. A. Boxall, Predictive Framework for Estimating Exposure of Birds to Pharmaceuticals, Environ. Toxicol. Chem., 2017, 36, 2335–2344 CrossRef CAS PubMed.
- J. S. Freshman and C. A. Menzie, Two Wildlife Exposure Models to Assess Impacts at the Individual and Population Levels and the Efficacy of Remedial Actions, Hum. Ecol. Risk Assess. Int. J., 1996, 2, 481–498 CrossRef.
- M. R. Pino, J. Val, A. M. Mainar, E. E. Zuriaga, C. Español, E. Langa, C. Espanol and E. Langa, Acute Toxicological Effects on the Earthworm Eisenia Fetida of 18 Common Pharmaceuticals in Artificial Soil, Sci. Total Environ., 2015, 518, 225–237 CrossRef PubMed.
-
European Medicines Agency, Guideline on environmental impact assessment for veterinary medicinal products in support of the VICH guidelines GL6 and GL38, London, 2016 Search PubMed.
- R. Ortíz-Castro, H. A. Contreras-Cornejo, L. Macías-Rodríguez and J. López-Bucio, The Role of Microbial Signals in Plant Growth and Development, Plant Signaling Behav., 2009, 4, 701–712 CrossRef.
- P. K. Jjemba, The Effect of Chloroquine, Quinacrine, and Metronidazole on Both Soybean Plants and Soil Microbiota, Chemosphere, 2002, 46, 1019–1025 CrossRef CAS PubMed.
- T. Eggen, E. S. Heimstad, A. O. Stuanes and H. R. Norli, Uptake and Translocation of Organophosphates and Other Emerging Contaminants in Food and Forage Crops, Environ. Sci. Pollut. Res., 2013, 20, 4520–4531 CrossRef CAS PubMed.
- A. W. Speedy, Global Production and Consumption of Animal Source Foods, J. Nutr., 2003, 133, 4048S–4053S CrossRef CAS PubMed.
-
Defra, Food Statistics Pocketbook 2016, Dep. Environment Food Rural Aff., 2016, p. 15 Search PubMed.
-
B. E. Sample, M. S. Aplin, R. A. Efroymson, G. W. Suter II and C. J. Welsh, Methods and Tools for Estimation of the Exposure of Terrestrial Wildlife to Contaminants, Tennessee, 1997 Search PubMed.
-
United States Environmental Protection Agency, Wildlife Exposure Factors Handbook, Washington, DC, 1993, vol. I Search PubMed.
- M. Delgado-Baquerizo, F. T. Maestre, P. B. Reich, T. C. Jeffries, J. J. Gaitan, D. Encinar, M. Berdugo, C. D. Campbell and B. K. Singh, Microbial Diversity Drives Multifunctionality in Terrestrial Ecosystems, Nat. Commun., 2016, 7, 10541 CrossRef CAS PubMed.
- D. C. Love, S. Rodman, R. A. Neff and K. E. Nachman, Veterinary Drug Residues in Seafood Inspected by the European Union, United States, Canada, and Japan from 2000 to 2009, Environ. Sci. Technol., 2011, 45, 7232–7240 CrossRef CAS PubMed.
- X. He, Z. Wang, X. Nie, Y. Yang, D. Pan, A. O. W. Leung, Z. Cheng, Y. Yang, K. Li and K. Chen, Residues of Fluoroquinolones in Marine Aquaculture Environment of the Pearl River Delta, South China, Environ. Geochem. Health, 2012, 34, 323–335 CrossRef CAS PubMed.
- P. W. Gerbens-Leenes and M. M. Mekonnen, The Water Footprint of Poultry, Pork and Beef: A Comparative Study in Different Countries and Production Systems, Water Resources and Industry, 2013, 1–2, 25–36 CrossRef.
- P. Verlicchi, M. Al Aukidy and E. Zambello, Occurrence of Pharmaceutical Compounds in Urban Wastewater: Removal, Mass Load and Environmental Risk after a Secondary Treatment—A Review, Sci. Total Environ., 2012, 429, 123–155 CrossRef CAS PubMed.
- S. Bondarenko, J. Gan, F. Ernst, R. Green, J. Baird and M. McCullough, Leaching of Pharmaceuticals and Personal Care Products in Turfgrass Soils during Recycled Water Irrigation, J. Environ. Qual., 2012, 41, 1268 CrossRef CAS PubMed.
|
This journal is © The Royal Society of Chemistry 2019 |
Click here to see how this site uses Cookies. View our privacy policy here.