Provenance of drinking water revealed through compliance sampling†
Received
17th September 2018
, Accepted 7th May 2019
First published on 29th May 2019
Abstract
Understanding drinking water hydrochemistry is essential for maintaining safe drinking water supplies. Whilst targeted research surveys have characterised drinking water hydrochemistry, vast compliance datasets are routinely collected but are not interrogated amidst concerns regarding the impact of mixed water sources, treatment, the distribution network and customer pipework. In this paper, we examine whether compliance samples retain hydrochemical signatures of their provenance. We first created and subsequently undertook the first hydrochemical analysis of a novel national database of publically available drinking water compliance analyses (n = 3
873
941) reported for 2015 across England and Wales. k-means cluster analysis revealed three spatially coherent clusters. Cluster 1 is dominated by groundwater sources, with high nitrate concentrations and mineralisation, and lower organic carbon, residual chlorine and THM formation. Cluster 2 was dominated by surface water sources and characterised by low mineralisation (low conductivity and major ion concentrations), low nitrate and high organic carbon concentrations (and hence residual chlorine and THM formation). Cluster 3 shows a mixture of groundwater overlain by confining layers and superficial deposits (resulting in higher trace metal concentrations and mineralisation) and surface water sources. These analyses demonstrate that, despite extensive processing of drinking water, at the national scale signatures of the provenance of drinking water remain. Analysis of compliance samples is therefore likely to be a helpful tool in the characterisation of processes that may affect drinking water chemistry. The methodology used is generic and can be applied in any area where drinking water chemistry samples are taken.
Environmental significance
Understanding drinking water hydrochemistry is essential for maintaining safe water supplies. We examined whether routine drinking water samples retain hydrochemical signatures of their provenance. We undertook the first hydrochemical analysis of a novel national database of routine drinking water samples. Principal component analysis and cluster analysis revealed three spatially coherent clusters reflecting groundwater and surface water sources. Despite treatment of drinking water, at the national scale signatures of the provenance remain. Analysis of routine compliance samples is therefore likely to be a helpful tool in the characterisation of environmental processes occurring that may affect drinking water quality.
|
1 Introduction
Access to safe drinking water is a human right and a requirement for life.1 In the developed world, the quality of water supplies has improved substantially in the past 25 years, largely through the introduction of regulation and advances in treatment.2 In Europe, implementation of the European Union Drinking Water Directive (EUDWD, European Commission3) has resulted in compliance levels of over 99% in 2016.4 Similar directives are also in place internationally (e.g. Australia,5 USA6 and China7).
Against a backdrop of climate change and increased demand,8 water utilities are increasingly considering the use of raw and treated water transfers to supply customers.9 Feasibility studies of local, small scale water transfers in the UK are required to establish the viability of a transfer in terms of environmental water resource availability and both drinking water and environmental water quality.10 However, outside of the UK this is not always the case, as highlighted by the recent Flint Water Crisis.11 In this case, the addition of highly corrosive surface water into a distribution system without corrosion control resulted in a significant public health incident.12 Outside of the UK switching of supply water chemistry may be done without any systematic evaluation,13 and assessing the impacts of drinking water chemistry on potential future large scale raw and potable transfers is considered a significant research need.14
The hydrochemical analyses required in order to support assessment of the water quality implications of transfers are complex. Changes in water quality associated with the mixing of raw water sources, treatment processes, passage through a utilities' distribution system and customer plumbing make unambiguous interpretation of drinking water chemistry data challenging.15 Despite this, numerous studies have characterised drinking water hydrochemistry using specific sampling and laboratory analyses for research purposes.15–24 A number of studies taking this approach have shown a strong link between drinking water hydrochemistry and raw water sources. Dinelli et al.17 and Demetriades25 showed a clear influence of bedrock geology and aquifer composition on major and trace elements in drinking waters in Italy and Greece respectively. Birke et al.23 showed uranium concentrations in drinking water to have a strong geological control. At the European scale, Banks et al.21 and Flem et al.15 showed that drinking water hydrochemistry can be interpreted in terms of source water hydrogeology and land use, as these factors influence raw water chemistry. These authors concluded that drinking water sampling is a highly cost-effective approach to characterise controls on water chemistry at the European scale, with confident interpretation of numerous parameters in terms of hydrogeochemical processes. Stable oxygen and hydrogen isotopes of drinking water have also been shown to be a useful tracer of source waters and hydrological processes both at the national26–28 and city scale29 in the USA and China. In the UK, national scale drinking water trends broadly following the same spatial pattern as unconfined groundwaters.30
There have been substantial reductions in funding for environmental regulators in recent years in some developed countries.31,32 Consequently, environmental monitoring programmes have declined.33 In England and Wales the number of water chemistry measurements taken by the environmental regulator has declined by 40% between 1993 and 2014.34 Environmental water chemistry monitoring is typically devolved to a regional level which results in substantial spatial bias in sampling, as well as both spatial and temporal variability in sampling methodologies, laboratory methods, standards, reporting procedures and data quality assurance.35 With a limited and reducing spatiotemporal extent of environmental water chemistry monitoring, it is essential that other data sources are considered for the characterisation of water chemistry required to assess the viability of raw and treated water transfers. In addition to drinking water datasets collected specifically for research purposes, large drinking water chemistry datasets have been and continue to be collected for regulatory compliance across the developed world (e.g. Europe4 and USA36). Under the EUDWD, around 100
000 water supply zones are routinely sampled for regulatory compliance across Europe.3 The need for data for regulatory compliance results in consistent laboratory standards, extensive data quality assurance and a large spatiotemporal sampling extent.3,37 These datasets have never been analysed in terms of their hydrochemical characteristics and, potentially, represent a vast and powerful dataset that could complement environmental water chemistry datasets and specific national17,25 and continental scale drinking water research surveys.15,21
If water transfers are to be developed to meet future demand, it is essential that the hydrochemistry of current the drinking water distribution is better understood. Moreover, beyond water quality compliance reports, very little public information is available from water utilities on drinking water sources and associated hydrochemistry. To this end, we examined whether drinking water samples for regulatory compliance retain the hydrochemical signatures of their provenance? In this study we present the first national-scale assessment of the hydrochemistry of drinking water based on compliance sampling. Applied to England and Wales, we derived spatially distributed water chemistry datasets based on published water company reports. We then undertook spatial and statistical analyses to determine the likely factors controlling the spatial variation in drinking water chemistry. Finally, we provide an outlook on the use of these datasets for future analysis of drinking water hydrochemistry.
2 Materials and methods
2.1 Study area and regulatory context
The countries of England and Wales were used as a study area for the research reported here (Fig. 1). Drinking water supplies are obtained from both surface water and groundwater sources, approximately in the ratio 60
:
40 overall,38 with raw water characteristics and treatment requirements reflecting these different sources. Most water utilities supply water from both surface water and groundwater sources, although in very different proportions depending on geographical location and underlying geology. The most important aquifers used for water supply in the study area are the Chalk and the Permo–Triassic rocks (referred to as Permo–Triassic or PT herein), are shown in Fig. 1. At one extreme in East Anglia, one utility draws drinking water supplies only from groundwater and predominantly from the Chalk aquifer,39 whereas in Wales over 90% of water supplied is from surface water sources.40
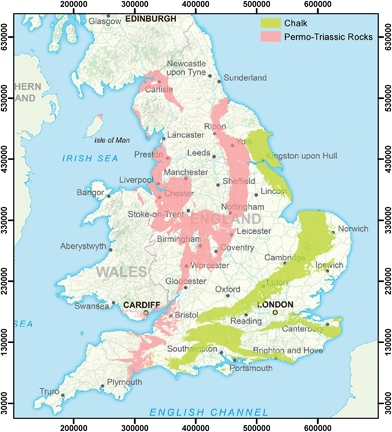 |
| Fig. 1 Location of the study area of England and Wales within the United Kingdom and the outcrop of the Chalk and Permo–Triassic rocks. Contains Ordnance Data© Crown Copyright and database rights 2017. Ordnance Survey Licence no. 100021290. | |
As previously discussed, drinking water quality is regulated under the EUDWD. This is transposed into UK law through primary legislation and regulations as the Water Supply (Water Quality) Regulations.41 Water is deemed to be wholesome if it does not contain substances which contravene the concentrations listed in the Directive or National monitoring categories in ESI Table 1.† A further group of substances (indicator parameters) are also monitored and reported. Non-regulated substances, such as calcium, magnesium and alkalinity, are measured less frequently and reporting of results is not required.
2.2 Water quality sampling
The 27 individual water utilities in England and Wales undertake water quality compliance sampling to meet the requirements of the EUDWD. Measurements are made either at the customer's tap, at a supply point (SP) or at the water treatment works (WTW) exit as set down in the regulations and agreed with the UK Drinking Water Inspectorate (DWI). Monitoring at WTW and service reservoirs (SR) is to quantify levels of residual disinfectant, and control of microbiological parameters and nitrite. Substances can be monitored at designated SPs instead of taps where concentrations are not deemed to change in the distribution network. ESI Table 1† shows both compliance and indicator parameters and location of sampling points. Guidance on the analysis of samples to ensure consistency is provided by the DWI, for a full range of aspects including analyst training, suitable equipment and calibration, method specification, internal and external analytical quality control and record retention.37 Pesticides and microbiological parameters are not considered in this assessment.
2.3 Data extraction, collation and statistical analysis
Under the Water Supply (Water Quality) Regulations,41 the water supply utilities in England and Wales provide the results of the routine water quality sampling detailed above as PDF reports to customers on their websites. Water utility supply areas are divided based on operational factors into designated water supply zones (WSZ), which supply up to 100
000 people, have approximately uniform quality and can comprise a combination of small communities in rural areas. Each water quality report is for a defined WSZ and, under normal conditions, on request all customers within a WSZ receive the same report. We extracted WSZ reports using a similar approach to that reported by Ascott et al.42 Reports can be downloaded using a postcode search. The locations of WSZ boundaries are sometimes available but not consistently across the study area. We downloaded all WSZ water quality reports for water companies in England and Wales for 2015. Where WSZ boundary mapping was not available, we derived WSZ areas based on postcode data. We divided England and Wales into a series of 1 km square grid cells. For each grid cell, the postcode in the centre of the cell was extracted and the name of the corresponding WSZ recorded. We then merged the areas returning the same WSZ report to derive the WSZ area outlines. The downloaded water quality reports for each WSZ were then converted using the tabula software43 and collated in a MS Access database.
A large number of parameters are reported in the WSZ water quality reports as listed in ESI Table 1.† From this list we used the following criteria to exclude parameters which are unlikely to reflect water provenance at the national scale:
• Copper, iron, aluminium, fluoride, lead and manganese, as these are all parameters that may be significantly impacted by water treatment, the distribution network and customer pipework.
• Phosphorus was not considered further due to the widespread practice of phosphate dosing during water treatment.44 Whilst chlorine and THMs are also artefacts of water treatment processes, these parameters were included in the analysis as chlorination (and subsequent THM formation) is more extensive in treatment of surface waters than groundwaters15 and thus may be an indicator of provenance.
• No substantial data gaps at the national scale (<5% of water supply zones with missing data for a certain parameter). As analysis for individual pesticides is assessed on a risk basis, monitoring is not consistent across all WSZs so these were excluded.
• No datasets dominated by zero detects (bacterial counts, specific organic compounds (e.g. benzene), radioactivity, taste/odour, pesticides).
Applying these criteria resulted in 17 parameters that are likely to reflect provenance, as shown in Table 1. We then undertook further statistical analysis of these parameters. Some authors45 have advocated the use of compositional methods46 to analyse water quality samples. These approaches acknowledge that the concentrations of constituents in a sample sum to a whole and thus artefacts can arise in standard analyses because an increase in the concentration of one constituent leads directly to a decrease in the concentrations of the other constituents. Also, the sum of independent predictions of each constituent do not generally sum to the whole. In a compositional approach these artefacts are avoided since the concentrations are transformed to relative ratios of (often log-transformed) constituents or products of constituents. We do not believe that such an approach is required here for a number of reasons. First, quantities such as pH, turbidity and conductivity do not form part of composition and could not be included in a compositional analysis. Also, the magnitude of changes to the concentration of one constituent that result from a change to the concentration of a second constituent are not likely be large because the concentrations of the second constituent will also be small relative to the amount of water in the sample. Furthermore, the primary purpose of compliance monitoring is to determine whether concentrations of individual constituents are above pre-specified thresholds. Breaches of these thresholds will be harder to interpret if the analysis is conducted in a transformed space which focuses on the ratio of concentrations of different constituents of a sample rather than the magnitude of the concentrations.
Table 1 Mean and standard deviation for determinands for drinking water samples classified according to bedrock geology (principal aquifers (Permo–Triassic (PT) and Chalk) and less productive aquifers and non-aquifers). Results of the significance test of Lark and Cullis47 are shown in the last 6 columns. Positive sign indicates that the parameter is greater in the first rock type is greater than the second
Determinand |
Unit |
PCV |
Permo–Triassic rocks |
Chalk |
Other rocks |
PT–Chalk |
PT–other |
Chalk–other |
Mean |
SD |
Mean |
SD |
Mean |
SD |
Sign |
p
|
Sign |
p
|
Sign |
p
|
Ammonium |
mg NH4 L−1 |
0.5 |
0 |
0.01 |
0.01 |
0.03 |
0.03 |
0.06 |
− |
0.029 |
− |
<0.001 |
− |
<0.001 |
Antimony |
μg Sb L−1 |
5 |
0.08 |
0.13 |
0.04 |
0.09 |
0.08 |
0.13 |
+ |
0.096 |
− |
0.011 |
− |
<0.001 |
Arsenic |
μg As L−1 |
10 |
0.57 |
0.95 |
0.27 |
0.44 |
0.4 |
0.48 |
+ |
0.117 |
− |
0.221 |
− |
0.003 |
Boron |
mg B L−1 |
1 |
0.01 |
0.02 |
0.02 |
0.05 |
0.03 |
0.03 |
− |
<0.001 |
− |
<0.001 |
− |
0.011 |
Chloride |
mg Cl L−1 |
250 |
24.59 |
18.63 |
34.24 |
17.08 |
34.75 |
20.73 |
− |
<0.001 |
− |
<0.001 |
+ |
0.062 |
Chlorine |
mg Cl2 L−1 |
![[thin space (1/6-em)]](https://www.rsc.org/images/entities/char_2009.gif) |
0.38 |
0.23 |
0.28 |
0.12 |
0.38 |
0.21 |
+ |
<0.001 |
+ |
0.027 |
− |
<0.001 |
Chromium |
μg Cr L−1 |
50 |
0.15 |
0.28 |
0.2 |
0.45 |
0.14 |
0.29 |
+ |
0.083 |
+ |
0.003 |
+ |
0.298 |
Conductivity |
μS cm−1 @ 20 °C |
2500 |
314.25 |
182.79 |
571.77 |
95.32 |
446.18 |
215.17 |
− |
<0.001 |
− |
<0.001 |
+ |
<0.001 |
pH |
pH units |
6.50–9.50 |
7.5 |
0.26 |
7.41 |
0.16 |
7.55 |
0.25 |
+ |
<0.001 |
− |
<0.001 |
− |
<0.001 |
Nickel |
μg Ni L−1 |
20 |
0.79 |
1.04 |
1.07 |
1.69 |
1.22 |
1.11 |
− |
<0.001 |
− |
<0.001 |
− |
0.01 |
Nitrate |
mg NO3 L−1 |
50 |
10.83 |
10.68 |
25.26 |
11.07 |
13.45 |
10.58 |
− |
<0.001 |
− |
0.251 |
+ |
<0.001 |
Selenium |
μg Se L−1 |
10 |
0.18 |
0.3 |
0.5 |
0.61 |
0.28 |
0.43 |
− |
<0.001 |
− |
0.009 |
+ |
<0.001 |
Sodium |
mg Na L−1 |
200 |
18.3 |
12.65 |
19.2 |
10.98 |
23.12 |
13.06 |
− |
<0.001 |
− |
<0.001 |
− |
0.078 |
Sulphate |
mg SO4 L−1 |
250 |
38.32 |
25.86 |
34.51 |
24.04 |
49.61 |
30.99 |
+ |
0.431 |
− |
<0.001 |
− |
<0.001 |
Total organic carbon |
mg L−1 |
|
1.03 |
0.71 |
0.94 |
0.58 |
1.62 |
0.84 |
+ |
0.014 |
− |
<0.001 |
− |
<0.001 |
Total trihalomethanes |
μg L−1 |
100 |
26.47 |
13.41 |
12.13 |
8.29 |
24.44 |
11.78 |
+ |
<0.001 |
+ |
0.897 |
− |
<0.001 |
Turbidity |
NTU |
4 |
0.03 |
0.06 |
0.04 |
0.06 |
0.06 |
0.06 |
− |
0.006 |
− |
<0.001 |
− |
0.004 |
The statistical analysis required measurements of all parameters in all water supply zones. Of the 17 parameters, data were missing for an average of 2.85% of water supply zones. Where data were missing we infilled using the median value of the same parameter at other sites. The median is a robust measure of the expected value that is not unduly influenced by outliers, and the proportion of data requiring infilling is very small. Thus this infilling is unlikely to introduce artefacts into the eventual clusters. The mean and standard deviation was calculated for each determinand spilt up by aquifer type (Chalk, Permo–Triassic rocks, less productive and non-aquifers). The data were not suitable for a conventional analysis of variance because they were spatially correlated and non-normally distributed. We therefore normalised the data and then followed the approach described by Lark and Cullis47 to test the significance of any differences in the mean values of each variable for each rock type. Briefly, we transformed the observations of each variable to a normal distribution by a non-parametric (normal-scores) approach and then estimated a linear mixed model of the transformed variable. The fixed effects of that linear mixed model were categorical variables corresponding to the three rock types and the random effects were assumed to have an exponential spatial covariance function. A series of Wald tests were then applied to test for significant differences in the mean value of the transformed variable for each pair of rock types. The spatial distribution of each parameter was assessed qualitatively by developing national scale maps of the determinands with the outcrop of the principal aquifers overlain. These maps show the raw data across the areal extent of WSZs, with no interpolation undertaken. The 17 parameters were standardised and we then undertook k-means cluster analysis for k = 2 to k = 5 (ref. 48) using R.49 As the choice of an appropriate number of clusters is somewhat subjective, we developed a parsimonious, rule based approach. We identified the smallest number of clusters which (1) produces spatially coherent cluster membership at the national scale and (2) the spatial patterns of cluster membership correspond to areas of groundwater and surface water supplies. Using this approach, 3 clusters were identified as representing drinking water provenance on the basis of groundwater and surface water at the national scale. Increasing the number of clusters above 3 resulted in incoherent patterns of cluster membership. Such patterns are likely to represent more local scale hydrochemical processes effecting tap water chemistry which are not the focus of this national scale study.
3 Results
3.1 Database statistics and regulatory compliance
The database developed covers 1539 supply zones across England and Wales. Based on the downloaded water quality reports a total of 3
873
941 water chemistry samples were reported in 2015. There are 190 unique determinands within the database. For each determinand within a WSZ, a maximum, minimum and mean concentration is reported, in addition to the number of samples taken in the year and the number that exceeded the drinking water limit. For each water supply zone the number of determinands varies substantially. The maximum and median number of determinands reported for a WSZ was 272 and 75 respectively. This wide range in the number of determinands is the result of different water supply zones having different reporting requirements associated with different population levels. Water companies operating water supply zones which have experienced water quality problems associated with certain parameters may have a regulatory obligation to report these parameters. This is often the case with individual pesticides, which cover 111 of 190 determinands. The sample data, however, show a high level of compliance to DWI and EUDWD standards, with 99.94% of samples compliant. This agrees well with the reported compliance statistics presented by Drinking Water Inspectorate2 for 2014 (99.96% for England).
3.2 Spatial distribution of determinands
In this section, the spatial distribution of concentration data for key parameters within drinking water is presented. Determinands have been grouped based on similarity in their spatial distribution. Table 1 shows the mean and standard deviation of the determinands analysed split by principal aquifers (Chalk and Permo–Triassic Rocks) and less productive aquifers and non-aquifers. Also shown are the results of the significance test of Lark and Cullis.47 Statistically significant differences were observed between the rock types for 10 out of the 17 parameters (p < 0.001, for PT–Chalk, PT–other and Chalk–other).
3.2.1 Nitrate.
Fig. 2 shows the spatial distribution of nitrate concentrations in drinking waters in England and Wales. High nitrate concentrations are present in south and east England corresponding broadly to the outcrop of the Chalk aquifer and some parts of the Permo–Triassic rocks. Analyses of drinking waters from areas of the Chalk show a very different nitrate concentration distribution to those from the Permo–Triassic sandstones, with higher mean values (25.2 mg L−1) and samples most frequently in the 20–40 mg L−1 range for Chalk compared to 10.8 mg L−1 and samples in the 0–10 mg L−1 range for the Permo–Triassic. Low concentrations are present where the Chalk is overlain by low-permeability Palaeogene and superficial deposits (primarily till) in East Anglia. Areas which are shown in white show returned no drinking water quality report. These areas can be considered to be where no mains supply is present and drinking water is obtained from local private supplies.
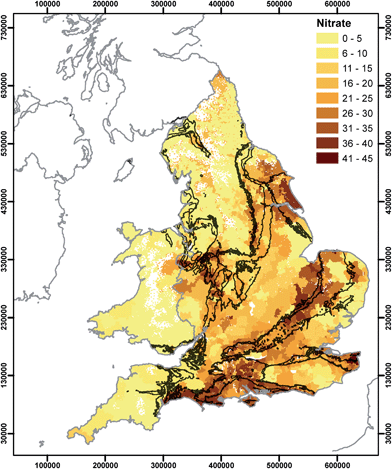 |
| Fig. 2 Nitrate concentrations (mg NO3 L−1) in drinking water in England and Wales in 2015. | |
3.2.2 Nickel and selenium.
Concentrations of trace substances (Ni, Se, As) are low over most of England and Wales. Elevated concentrations of substances such as Ni and Se, are found in areas of East Anglia where the Chalk is not at outcrop (Fig. 3). Mean Ni and Se concentrations are very low from supplies on the Permo–Triassic and approximately double from the Chalk (Table 1).
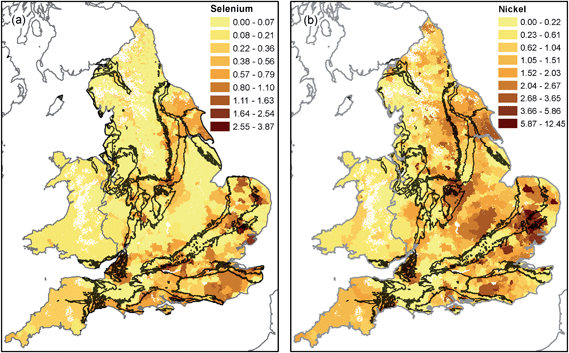 |
| Fig. 3 Selenium (a) and nickel (b) concentrations (μg L−1) in drinking water in England and Wales in 2015. | |
3.2.3 TOC, chlorine, THMs and turbidity.
Fig. 4 shows TOC, chlorine, THMs and turbidity concentrations for drinking water in the study area. Elevated TOC concentrations (of up to 3 mg L−1) are measured in the northeast coast of England, Anglesey, southwest England, Essex, and an area of Central England around Bedford, Northampton and Peterborough (Fig. 4). Average concentrations in supplies located on the aquifers of the Chalk and the Permo–Triassic are similar, about 1 mg L−1, whereas the average for less productive aquifers and non-aquifers is higher (1.62 mg L−1, Table 1).
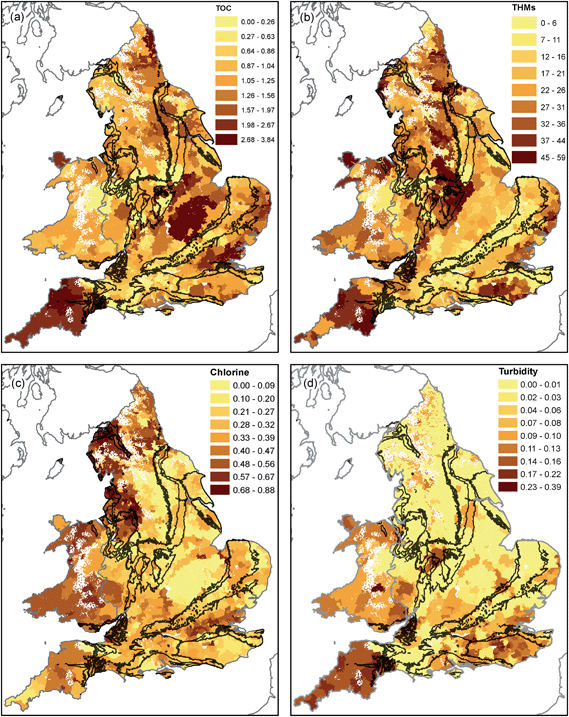 |
| Fig. 4 TOC (mg L−1, (a)), THMs (μg L−1, (b)), chlorine (mg L−1, (c)) and turbidity (NTU, (d)) in drinking water in England and Wales in 2015. | |
The highest residual chlorine concentrations are seen in northwest England (the Lake District Coast and Cheshire) and parts of Wales and southwest England (Fig. 4). Supplies from Chalk areas have the lowest average residual chlorine (0.28 mg L−1), with increasing concentrations on the Permo–Triassic and on less productive aquifers and non-aquifers (0.38 mg L−1, Table 1). Elevated THM concentrations of up to 50 μg L−1 occur in south Wales and southwest England, the Weald, easterly East Anglia and the Pennines (Fig. 4). Average concentrations in supplies on the Chalk are 12.1 μg L−1, whereas on the Permo–Triassic and less productive aquifers and non-aquifers they are in the range 24 to 26 μg L−1 (Table 1). Turbidity values are higher in southwest England and parts of Wales (up to 0.3 NTU) than eastern England (Fig. 4). Average values are similar across the study area with the lowest for the Permo–Triassic (0.03 NTU) and highest on less productive aquifers and non-aquifers (0.06 NTU) (Table 1).
3.2.4 Conductivity, chloride, sodium and sulphate.
Drinking water conductivity is lowest along the west coast and highest in eastern East Anglia where values of up to 900 μS cm−1 are recorded (Fig. 5). Mean conductivity values are considerably higher from areas on the Chalk than on the Permo–Triassic or less productive aquifers and non-aquifers (Table 1). Chloride concentrations follows a similar pattern to conductivity but with additional elevated concentrations in Cheshire and the East Midlands (Fig. 5). Mean chloride concentrations are higher on the Chalk and less productive aquifers and non-aquifers (34–35 mg L−1) than on the Permo–Triassic (24.6 mg L−1) (Table 1). Sodium also follows this pattern although average concentrations do not behave similarly. Mean sodium concentrations are higher on less productive aquifers and non-aquifers (23.1 mg L−1) than on the Permo–Triassic and on the Chalk (18–19 mg L−1). Sulphate is also similar with less obvious elevation of concentration in East Anglia and more in the East Midlands and Yorkshire. Average concentrations are in the range 20–30 mg L−1. Like sodium, mean concentrations are considerably higher on less productive aquifers and non-aquifers (49.6 mg L−1) than on the Permo–Triassic and the Chalk (34–39 mg L−1) (Table 1).
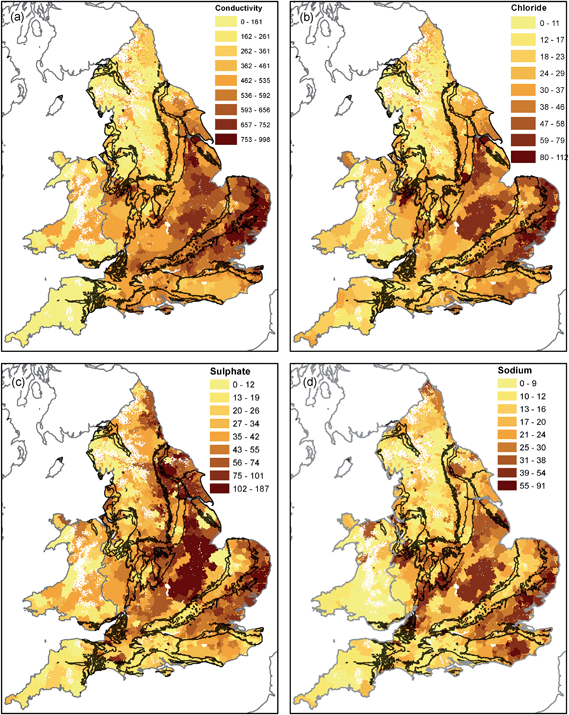 |
| Fig. 5 Conductivity (μS cm−1, (a)), chloride (mg L−1, (b)), sulphate (mg L−1, (c)) and sodium (mg L−1, (d)) in drinking water in England and Wales in 2015. | |
3.2.5 Other factors.
A small group of the 17 parameters only provide limited insight into hydrochemical processes. Ammonium concentrations are slightly elevated in confined areas of the Chalk in the London area and in East Anglia with some concentrations above 0.05 mg L−1. Average concentrations range from 0.03 mg L−1 on less productive aquifers and non-aquifers to <LOD in the Permo–Triassic. Arsenic concentrations are elevated in a few localities, in Cheshire, and the Bristol area. Average values are highest in the Permo–Triassic where it can be naturally occurring and lowest in the Chalk (Table 1). Average antimony concentrations are very low (0.04–0.08 μg L−1) but also exhibit locally higher concentrations in Cheshire. Boron concentrations are also very low (0.01–0.03 mg L−1) with highest concentrations in the Weald and in southern East Anglia.
3.3 Statistical analysis
Fig. 6 shows the results of the cluster analysis; three spatially coherent clusters can be identified. Cluster 1 comprises WSZs in the south east of England and some parts of the Midlands, with significant areas overlapping the outcrop of Chalk and Permo–Triassic aquifers. Cluster 2 WSZs are located in Wales and the southwest and the north of England, where there are limited groundwater resources. Cluster 3 is more spatially variable, covering parts of East Anglia and the southeast, the East Midlands and northeast England. In these areas there is a combination of groundwater resources (including the confined Chalk of East Anglia and Jurassic limestones) and surface water resources. The centroids (Fig. 7) show the differences between clusters for key determinands. Cluster 1 has high nitrate concentrations and conductivity, low organic carbon, chlorine and THM concentrations in comparison to cluster 2. Cluster 2 has low nitrate concentrations, conductivity, sodium and chloride concentrations and higher chlorine and THM concentrations. Cluster 3 has higher conductivity, sodium, chloride and sulphate concentrations in addition to higher boron, antimony, nickel and selenium concentrations. Cluster 3 also has relatively low chlorination and THMs, despite higher TOC concentrations.
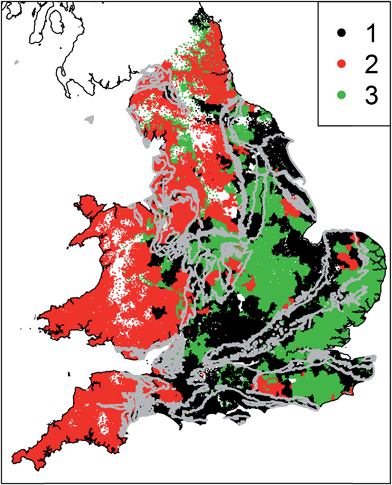 |
| Fig. 6 Location of water supply zone clusters. | |
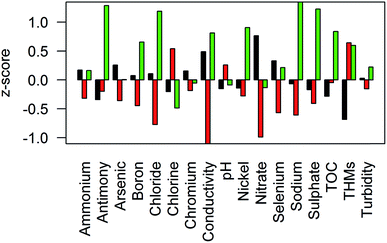 |
| Fig. 7 Z-scores for the cluster centroids. | |
4 Discussion
4.1 Hydrogeochemical controls on drinking water typologies
In this section we relate the spatial distributions presented in Section 3.2 to potential controlling factors in water provenance. It should be noted that water utilities use a number of options for ensuring that drinking water is compliant with the water quality regulations. These can include removal/reduction of determinands by water treatment which can result in regulated substances exhibiting a truncated distribution of concentrations. In this analysis, it is assumed that water comes either from groundwater or surface water. However, in the future drinking water may also be obtained by desalination. In England currently there is only one plant used to desalinate water using the reverse osmosis (RO) process for public supply, on the Thames Estuary, and which has operated since 2010 providing up to 150 ML per day during peak times.50 Drinking water derived from this source will differ significantly in terms of hydrochemistry compared to that from groundwater or surface water sources, because it is derived from the tidal zone of the Thames and has undergone demineralisation.51
The spatial distribution of nitrate concentrations (Fig. 2) shows a clear influence of both underlying hydrogeology and land use, identifiable in cluster 1 (Fig. 6). Large areas of southern and eastern England obtain the majority of their supplies from groundwater.52 The high nitrate concentrations in drinking waters derived from the Chalk may reflect the storage of nitrate in the thick Chalk unsaturated zone and slower flushing of nitrate following changes in agricultural management practices.53–57 This assessment does not include areas of the Chalk where it is not at outcrop, e.g. the eastern part of East Anglia where some elevated values are shown in Fig. 2. Drinking water chemistry demonstrates a residual land use/geology signature despite treatment of water for elevated nitrate.58 This is unsurprising given that nitrate removal by ion exchange is unlikely to be undertaken on raw waters where concentrations are below 50 mg NO3 L−1. It would be anticipated that phosphate would be similarly useful were its distribution not obscured by treatment for plumbosolvency.44,59–61
The spatial distribution of nickel and selenium (Fig. 3) reflects geochemical processes occurring as recharge occurs through overlying superficial deposits. For example, Ander et al.62 showed that oxidation of sulphide minerals (e.g. pyrite) in overlying till deposits in East Anglia is the primary source of high nickel concentrations in Chalk groundwater.
Total organic carbon and other associated parameters (Fig. 4) shows a clear influence of surface water, identifiable in cluster 2 (Fig. 6). Higher concentrations of total organic carbon (TOC) would be expected to occur in areas of hard-fractured rocks or sandstones where superficial deposits may be peaty and/or supplies may be predominantly from surface water.63 These areas correspond to the predominance of surface water supply. Trihalomethanes (THMs) are a long-recognised by-product of water disinfection by chlorine and result from reaction of chlorine with organic carbon.64 The reaction is enhanced in the presence of bromide.65,66 Higher dosing of chlorine is required in water with a higher TOC content to obtain an acceptable residual chlorine concentration. In this dataset, the spatial distribution of THMs shows a qualitative relationship to that of TOC (Fig. 4). Although quantitatively the relationship has substantial scatter (R2 = 0.21), this is broadly in agreement with the findings of Valdivia-Garcia et al.67 which showed dissolved organic carbon to be an important predictor variable in the spatial distribution of THMs. Together these substances (TOC, chlorine and THMs) provide a clear indication where water derived from surface water predominates in drinking water.
Conductivity and associated parameters (Fig. 5) show a strong east-west spatial trend likely to be associated with recharge processes. Rainfall for England and Wales is predominantly from the southwest with highest amounts recorded on upland areas of Wales and the Lake District and low values in Eastern England, including London, East Anglia and Lincolnshire. The distribution of conductivity values appears to be inversely related to recharge68 and therefore predominantly reflects meteorological setting. High chloride concentrations in Cheshire may be associated with halite deposits in the Mercia Mudstone and related salt mining activity.69 Conductivity and the major ions included in regulatory monitoring are likely to be little affected by drinking water treatment70 and therefore retain their hydrological signature of the raw waters. Chloride could be augmented by treatment for nitrate by ion-exchange (see Section 4.2).
4.2 Drinking water compliance sample data for hydrochemical characterisation: an outlook
4.2.1 Benefits and limitations.
As previously discussed, the interpretation of drinking water datasets for hydrochemistry has been shown to be challenging due to mixing of water sources, treatment, the distribution network and sampling point location. Nevertheless, the cluster analysis and the data discussed above clearly shows that compliance samples do reveal drinking water provenance in terms of the raw water sources that dominate water supply in the study area. For a number of these determinands, a relatively confident interpretation of the environmental controls on the spatial trends can be made. Flem et al.15 suggested that sampling and centralised analysis of drinking water may be an effective low cost method for gaining insights into processes effecting drinking water chemistry. Building on this, here we suggest that significant further understanding into these processes can be gained from analysis of compliance samples. Uniform analytical, sampling and reporting standards mean that datasets from different water companies can be compared. The use of compliance water company samples for hydrochemical characterisation over specific centralised sampling15,21 for research has both advantages and disadvantages. Compliance samples cover a much denser sampling network both spatially and temporally than specific samples. However, the parameter range for routine samples is restricted to determinands which are of concern for human health. Consequently, there are a significant number of parameters which are not consistently reported which would be of significant hydrogeochemical interest (e.g. alkalinity, dissolved oxygen, calcium, magnesium, potassium). As a result, it is unlikely that data from compliance sampling could be used in conventional hydrogeochemical analyses and modelling (e.g. development of Piper/Durov diagrams, PHREEQC modelling). For example, Shand et al.71 report on baseline groundwater chemistry for England and Wales focussing on major and minor aquifers and Smedley72 examined UK bottled water chemistry, which tends to reflect the relatively minor aquifers. These studies, to which this work is complementary, discuss primarily major ion chemistry and a range of trace elements not necessarily represented in drinking water regulatory monitoring.
4.2.2 Applications and further work.
Drinking water compliance data have been used extensively in regulatory reporting. Detailed hydrochemical analysis and interpretation of this data has never before been reported. We consider there to be a wide range of potential applications of the dataset used in the research reported here.
The data could be used to support management decisions regarding the potential water chemistry implications of raw and treated water transfers. Fig. 8 shows the location of the clusters identified in this study and suggested raw and treated water transfers.14 Where transfers are between clusters, addition of water of different hydrochemical typologies may have significant implications for both human and environmental health. Without further water treatment, transfers of corrosive surface waters into areas previously supplied by groundwater may result in dissolution of metals from water mains. Where mains water leakage is significant, transfers may result in a flux of water that is hydrochemically different to the water in the environment. Recent work has shown mains water leakage to be a significant source of phosphorus (P) to the environment.60,61,73,74 Transfer of phosphorus dosed mains water into an area without historic P dosing and subsequent leakage into the environment could represent a significant additional source of P. Application of the data presented in this study would be an ideal high-level screening tool to evaluate the water quality implications of water transfers at the national scale. At the level of individual transfers, substantial additional work would be required considering the water quality of both the transferred water and the current water in a supply zone, the distribution network age, material type and location.
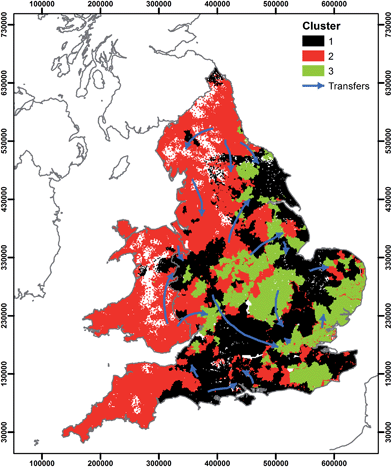 |
| Fig. 8 Location of water supply zone clusters and suggested14 large scale water transfers. | |
The datasets could be reviewed in the context of national scale health datasets. The Environment and Health Atlas75 provides detailed maps of both environmental agents and health conditions in England and Wales. This already includes trihalomethanes but could be extended to consider other potential environmental agents which are reported in the drinking water dataset. Drinking water in England and Wales are compliant with current regulations but such an approach could perhaps provide evidence to be used in future drinking water quality reviews.
The data collated in this study could also be compared against raw untreated water samples. This has been undertaken at a continental scale in Europe by Flem et al.76 but only using a small sample of drinking waters analysed centrally rather than routine compliance samples. This would give an indication of the efficiency of treatment processes. Comparison with groundwater and surface water data would also give an indication of whether water lost through leakage would be significantly different from the water in the environment. In some cases (e.g. phosphorus addition), leakage may be a source of nutrients to the environment. In contrast, in cases where treatment has removed contaminants from the water, leakage may dilute the concentration of pollutants already existing within groundwater or surface water.
In addition to the parameters reported here, there are a large number of other non-standard parameters reported on a case by case basis. The majority of these parameters (58%) are pesticides. Reporting for pesticides is risk-based and thus some determinands may only be reported for a small number of supply zones. The sporadic nature of these reports would make a statistical analysis such as the methodology presented here challenging, but an overall qualitative interpretation would be possible. Other authors advocate the use of compositional statistical methods to water compliance data46 which may yield different insights.
Further work could also explore changes in drinking water chemistry through time. The dataset reported in this study is for 2015. Historically water utilities have reported similar datasets to regulators back to 1993.77 A wide range of factors are likely to be controlling changes in water quality through time such as changes in source water quality, treatment processes and water source blending. Consequently unambiguous interpretation of such time series data is likely to be challenging.
The use of compliance samples to characterise drinking water provenance is likely to be broadly applicable across much of the developed world. In Europe, the EUDWD3 requires member states to report a number of determinands. High level compliance summaries are reported by the European Commission e.g. European Commission.4 In the USA, national databases78,79 are available which report compliance failures. Whilst a few countries hold publically accessible national scale databases for drinking water quality data (e.g. France80), in both the USA and large parts of Europe water quality data are held at the water company level. Given the high level of fragmentation in the water sector in both USA and Europe (>50
000 utilities in USA,81 >6200 in Germany alone82) data collation from individual companies would be an extremely labour intensive task. Given that water utilities already report compliance data to regulators, it would be helpful if regulators consistently provided these reports to the public in addition to high-level compliance summaries.
5 Conclusions
This study has shown that compliance samples reveal the hydrogeochemical provenance of drinking waters for the first time at the national scale. Despite extensive modification of source waters through treatment, blending and pipework, compliance data still show a hydrochemical signature of the source waters. The use of cluster analysis reveals a distinct groundwater–surface water split. The spatial distribution of a number of parameters which control this cluster partition (nitrate, nickel and selenium, TOC, THMs, conductivity) can be interpreted relatively unambiguously in terms of the source water hydrogeology. The approach used in this study is low cost and utilises existing datasets. It is highly generic and can be applied anywhere where compliance drinking water sampling is undertaken. The limited range of determinands measured during compliance sampling make this approach complementary to targeted hydrochemical investigations. The datasets developed have a wide range of applications including high level screening of the hydrochemical impacts of future water transfers, assessment of the impacts of water mains leakage on nutrient fluxes into the environment and comparison with national public health datasets.
Conflicts of interest
There are no conflicts to declare.
Acknowledgements
The authors thank the water companies of England and Wales to use their data for this research published in the public interest. All data are available publically on the websites of the water companies of England and Wales. Any opinions expressed in this paper are those of the authors and do not necessarily reflect those of any of these companies. This research was funded by the UKs Natural Environment Research Council (NERC) National Capability resources devolved to the British Geological Survey. This paper is published with permission of the Executive Director, British Geological Survey (NERC).
References
-
World Health Organisation, Guidelines for Drinking-water Quality, Geneva, Switzerland, 4th edn, 2011 Search PubMed.
-
Drinking Water Inspectorate, Drinking water quality in England: The position after 25 years of regulation, London, 2015 Search PubMed.
- European Commission, Drinking Water Directive (Council Directive 98/83/EC of 3 November 1998 on the quality of water intended for human consumption), Official Journal L, 1998, 330, 32–54 Search PubMed.
-
European Commission, Synthesis Report on the Quality of Drinking Water in the Union examining Member States' reports for the 2011-2013 period, foreseen under Article 13(5) of Directive 98/83/EC. COM/2016/0666 final, Brussels, 2016 Search PubMed.
-
NRMMC, Australian Drinking Water Guidelines Paper 6 National Water Quality Management Strategy, National Health and Medical Research Council, National Resource Management Ministerial Council, Commonwealth of Australia, Canberra, 2011 Search PubMed.
-
United States Environmental Protection Agency, Safe Drinking Water Act (SDWA), https://www.epa.gov/sdwa Search PubMed.
- W. Qu, W. Zheng, S. Wang and Y. Wang, China's new national standard for drinking water takes effect, Lancet, 2012, 380, e8 CrossRef.
- P. Simpson, Water stewardship in the twenty-first century, Nat. Clim. Change, 2014, 4, 311–313 CrossRef.
-
Committee on Climate Change, CCRA2: Updated projections for water availability for the UK. Final Report. MAR5354- RT002-R05-00, Wallingford, 2016 Search PubMed.
-
Environment Agency, Water Resources Planning Guideline: The Technical Methods and Instructions, Environment Agency, Bristol, UK, 2012 Search PubMed.
- L. J. Butler, M. K. Scammell and E. B. Benson, The Flint, Michigan, Water Crisis: A Case Study in Regulatory Failure and Environmental Injustice, Environ. Justice, 2016, 9, 93–97 CrossRef.
-
Flint Water Advisory Task Force, Final Report, Michigan, 2016 Search PubMed.
- G. Liu, Y. Zhang, W.-J. Knibbe, C. Feng, W. Liu, G. Medema and W. van der Meer, Potential impacts of changing supply-water quality on drinking water distribution: A review, Water Res., 2017, 116, 135–148 CrossRef CAS PubMed.
-
Water UK, Water resources long-term planning framework, London, 2016 Search PubMed.
- B. Flem, C. Reimann, M. Birke, D. Banks, P. Filzmoser and B. Frengstad, Inorganic chemical quality of European tap-water: 2. Geographical distribution, Appl. Geochem., 2015, 59, 211–224 CrossRef CAS.
- P. L. Smedley, D. M. Cooper and D. J. Lapworth, Molybdenum distributions and variability in drinking water from England and Wales, Environ. Monit. Assess., 2014, 186, 6403–6416 CrossRef CAS PubMed.
- E. Dinelli, A. Lima, S. Albanese, M. Birke, D. Cicchella, L. Giaccio, P. Valera and B. De Vivo, Major and trace elements in tap water from Italy, J. Geochem. Explor., 2012, 112, 54–75 CrossRef CAS.
- B. Le Bot, J.-P. Lucas, F. Lacroix and P. Glorennec, Exposure of children to metals via tap water ingestion at home: Contamination and exposure data from a nationwide survey in France, Environ. Int., 2016, 94, 500–507 CrossRef CAS PubMed.
- E. I. Brima, Physicochemical properties and the concentration of anions, major and trace elements in groundwater, treated drinking water and bottled drinking water in Najran area, KSA, Appl. Water Sci., 2017, 7, 401–410 CrossRef CAS.
- E. M. Carstea, E. A. Levei, M.-A. Hoaghia and R. Savastru, Quality assessment of Romanian bottled mineral water and tap water, Environ. Monit. Assess., 2016, 188, 521 CrossRef PubMed.
- D. Banks, M. Birke, B. Flem and C. Reimann, Inorganic chemical quality of European tap-water: 1. Distribution of parameters and regulatory compliance, Appl. Geochem., 2015, 59, 200–210 CrossRef CAS.
- K. Y. Patterson, P. R. Pehrsson and C. R. Perry, The mineral content of tap water in United States households, J. Food Compos. Anal., 2013, 31, 46–50 CrossRef CAS.
- M. Birke, U. Rauch, H. Lorenz and R. Kringel, Distribution of uranium in German bottled and tap water, J. Geochem. Explor., 2010, 107, 272–282 CrossRef CAS.
- R. Cidu, F. Frau and P. Tore, Drinking water quality: Comparing inorganic components in bottled water and Italian tap water, J. Food Compos. Anal., 2011, 24, 184–193 CrossRef CAS.
- A. Demetriades, Quality of hellenic bottled mineral and tap water as a proxy for groundwater geochemistry, European Geologist, 2013, 35, 9–16 Search PubMed.
- G. J. Bowen, J. R. Ehleringer, L. A. Chesson, E. Stange and T. E. Cerling, Stable isotope ratios of tap water in the contiguous United States, Water Resour. Res., 2007, 43, W03419 CrossRef.
- J. M. Landwehr, T. B. Coplen and D. W. Stewart, Spatial, seasonal, and source variability in the stable oxygen and hydrogen isotopic composition of tap waters throughout the USA, Hydrol. Processes, 2014, 28, 5382–5422 CrossRef CAS.
- S. Zhao, H. Hu, F. Tian, Q. Tie, L. Wang, Y. Liu and C. Shi, Divergence of stable isotopes in tap water across China, Sci. Rep., 2017, 7, 43653 CrossRef PubMed.
- Y. Jameel, S. Brewer, S. P. Good, B. J. Tipple, J. R. Ehleringer and G. J. Bowen, Tap water isotope ratios reflect urban water system structure and dynamics across a semiarid metropolitan area, Water Resour. Res., 2016, 52, 5891–5910 CrossRef CAS.
- W. Darling, A. Bath and J. Talbot, The O & H stable isotopic composition of fresh waters in the British Isles. 2. Surface waters and groundwater, Hydrol. Earth Syst. Sci., 2003, 7, 183–195 CrossRef CAS.
-
Scientific American, Trump Wants Deep Cuts in Environmental Monitoring, https://www.scientificamerican.com/article/trump-wants-deep-cuts-in-environmental-monitoring/ Search PubMed.
-
The Independent, Massive job cuts at the Environment Agency, http://www.independent.co.uk/news/uk/politics/massive-job-cuts-at-the-environment-agency-8909182.html.
-
M. Acevedo, Real-Time Environmental Monitoring: Sensors and Systems, CRC Press, Florida, USA, 2016 Search PubMed.
-
Environment Agency, Water Quality Archive, 2017 Search PubMed.
- M. Stuart, P. Chilton, D. Kinniburgh and D. Cooper, Screening for long-term trends in groundwater nitrate monitoring data, Q. J. Eng. Geol. Hydrogeol., 2007, 40, 361–376 CrossRef CAS.
-
United States Environmental Protection Agency, Drinking Water Tools, https://www.epa.gov/waterdata/drinking-water-tools, (accessed 24 November 2016) Search PubMed.
-
Drinking Water Inspectorate, Guidance on the implementation of the Water Supply (Water Quality) Regulations (as amended) in England, London, 2012 Search PubMed.
-
Department for Environment Food & Rural Affairs, Water abstraction from non-tidal surface water and groundwater: England and Wales, https://www.gov.uk/government/statistics/water-abstraction-estimates, (accessed 24 November 2016) Search PubMed.
-
Cambridge Water Company, Your Supply, http://www.cambridge-water.co.uk/customers/customer-supply, (accessed 24 November 2016) Search PubMed.
-
Dŵr Cymru, Water resources, http://www.dwrcymru.com/en/Environment/Water-Resources.aspx, (accessed 24 November 2016) Search PubMed.
-
Drinking Water Inspectorate, Water Supply (Water Quality) Regulations, http://www.legislation.gov.uk/uksi/2016/614/pdfs/uksi_20160614_en.pdf, 2016 Search PubMed.
- M. J. Ascott, D. C. Gooddy and B. W. J. Surridge, Public Water Supply Is Responsible for Significant Fluxes of Inorganic Nitrogen in the Environment, Environ. Sci. Technol., 2018, 52(24), 14050–14060 CrossRef CAS PubMed.
-
Tabula, Tabula 1.1.1, http://tabula.technology/ Search PubMed.
-
C. Hayes, Best practice guide on the control of lead in drinking water, IWA Publishing, 2010 Search PubMed.
- A. Buccianti and V. Pawlowsky-Glahn, New Perspectives on Water Chemistry and Compositional Data Analysis, Math. Geol., 2005, 37, 703–727 CrossRef CAS.
- J. Aitchison, The Statistical Analysis of Compositional Data, J. R. Stat. Ser. Soc. B Stat. Methodol., 1982, 44, 139–160 Search PubMed.
- R. Lark and B. Cullis, Model-based analysis using REML for inference from systematically sampled data on soil, Eur. J. Soil Sci., 2004, 55, 799–813 CrossRef.
-
R. Webster and M. A. Oliver, Statistical methods in soil and land resource survey, Oxford University Press (OUP), 1990 Search PubMed.
-
R Core Team, R: A language and environment for statistical computing, R Foundation for Statistical Computing, Vienna, Austria, http://www.R-project.org/, 2013.
-
Thames Water, Thames Gateway Water Treatment Works, http://www.thameswater.co.uk/help-and-advice/18132.htm, accessed 24 November 2016 Search PubMed.
-
Water Projects Online, Thames Gateway Water Treatment Plant, http://www.waterprojectsonline.com/case_studies/2010/Thames_Beckton_2010.pdf Search PubMed.
-
M. J. Ascott, Groundwater resources in the UK, http://www.bgs.ac.uk/research/groundwater/waterResources/GroundwaterInUK/home.html Search PubMed.
- L. Wang, M. E. Stuart, J. P. Bloomfield, A. S. Butcher, D. C. Gooddy, A. A. McKenzie, M. A. Lewis and A. T. Williams, Prediction of the arrival of peak nitrate concentrations at the water table at the regional scale in Great Britain, Hydrol. Processes, 2012, 26, 226–239 CrossRef CAS.
- L. Wang, A. Butcher, M. Stuart, D. Gooddy and J. Bloomfield, The nitrate time bomb: a numerical way to investigate nitrate storage and lag time in the unsaturated zone, Environ. Geochem. Health, 2013, 35, 667–681 CrossRef CAS PubMed.
- L. Wang, M. Stuart, M. Lewis, R. Ward, D. Skirvin, P. Naden, A. Collins and M. Ascott, The changing trend in nitrate concentrations in major aquifers due to historical nitrate loading from agricultural land across England and Wales from 1925 to 2150, Sci. Total Environ., 2016, 542, 694–705 CrossRef CAS PubMed.
- M. J. Ascott, D. C. Gooddy, L. Wang, M. E. Stuart, M. A. Lewis, R. S. Ward and A. M. Binley, Global patterns of nitrate storage in the vadose zone, Nat. Commun., 2017, 8, 1416 CrossRef CAS PubMed.
- M. J. Ascott, L. Wang, M. E. Stuart, R. S. Ward and A. Hart, Quantification of nitrate storage in the vadose (unsaturated) zone: a missing component of terrestrial N budgets, Hydrol. Processes, 2016, 30, 1903–1915 CrossRef CAS.
-
UK Water Industry Research Ltd, Implications of Changing Groundwater Quality for Water Resources and the UK Water Industry-Phase 3: Financial and Water Resources Impact, UKWIR, London, 2004 Search PubMed.
- M. J. Ascott, D. C. Gooddy, D. J. Lapworth, P. Davidson, M. J. Bowes, H. P. Jarvie and B. W. J. Surridge, Phosphorus fluxes to the environment from mains water leakage: Seasonality and future scenarios, Sci. Total Environ., 2018, 636, 1321–1332 CrossRef CAS PubMed.
- D. C. Gooddy, M. J. Ascott, D. J. Lapworth, R. S. Ward, H. P. Jarvie, M. J. Bowes, E. Tipping, R. Dils and B. W. J. Surridge, Mains water leakage: Implications for phosphorus source apportionment and policy responses in catchments, Sci. Total Environ., 2017, 579, 702–708 CrossRef CAS PubMed.
- M. J. Ascott, D. C. Gooddy, D. J. Lapworth and M. E. Stuart, Estimating the leakage contribution of phosphate dosed drinking water to environmental phosphorus pollution at the national-scale, Sci. Total Environ., 2016, 572, 1534–1542 CrossRef CAS PubMed.
-
E. L. Ander, P. Shand, K. J. Griffiths, A. R. Lawrence, P. Hart and J. Pawley, Baseline Report Series: 13. The Great Ouse Chalk aquifer, East Anglia, British Geological Survey Commissioned Report CR/04/236N, Keyworth, Nottingham, 2004 Search PubMed.
- F. Worrall, R. Harriman, C. D. Evans, C. D. Watts, J. Adamson, C. Neal, E. Tipping, T. Burt, I. Grieve, D. Monteith, P. S. Naden, T. Nisbet, B. Reynolds and P. Stevens, Trends in Dissolved Organic Carbon in UK Rivers and Lakes, Biogeochemistry, 2004, 70, 369–402 CrossRef CAS.
- J. A. Cotruvo, Trihalomethanes (THMs) in drinking water, Environ. Sci. Technol., 1981, 15, 268–274 CrossRef CAS PubMed.
- S. Chowdhury, P. Champagne and P. J. McLellan, Investigating effects of bromide ions on trihalomethanes and developing model for predicting bromodichloromethane in drinking water, Water Res., 2010, 44, 2349–2359 CrossRef CAS PubMed.
- W. J. Cooper, R. G. Zika and M. S. Steinhauer, Bromide-oxidant interactions and THM formation: a literature review, J. Am. Water Work. Assoc., 1985, 116–121 CrossRef CAS.
- M. Valdivia-Garcia, P. Weir, Z. Frogbrook, D. W. Graham and D. Werner, Climatic, Geographic and Operational Determinants of Trihalomethanes (THMs) in Drinking Water Systems, Sci. Rep., 2016, 6, 35027 CrossRef CAS PubMed.
- M. M. Mansour, L. Wang, M. Whiteman and A. G. Hughes, Estimation of spatially distributed groundwater potential recharge for the United Kingdom, Q. J. Eng. Geol. Hydrogeol., 2018, 51(2), 247–263 CrossRef.
-
K. J. Griffiths, P. Shand and J. Ingram, Baseline report series. 8, the Permo-Triassic sandstones of Manchester and east Cheshire, Environment Agency, Bristol, UK, 2003 Search PubMed.
- A. Asenbaum, C. Pruner, H. Kabelka, A. Philipp, E. Wilhelm, R. Spendlingwimmer, A. Gebauer and R. Buchner, Influence of various commercial water treatment processes on the electric conductivity of several drinking waters, J. Mol. Liq., 2011, 160, 144–149 CrossRef CAS.
-
P. Shand, W. M. Edmunds, A. R. Lawrence, P. Smedley and S. Burke, The natural (baseline) quality of groundwater in England and Wales, British Geological Survey Research Report RR/07/06 and Environment Agency Technical Report NC/99/74/24, Nottingham, 2007 Search PubMed.
- P. L. Smedley, A survey of the inorganic chemistry of bottled mineral waters from the British Isles, Appl. Geochem., 2010, 25, 1872–1888 CrossRef CAS.
- D. J. Lapworth, D. C. Gooddy, D. Allen and G. H. Old, Understanding groundwater, surface water, and hyporheic zone biogeochemical processes in a Chalk catchment using fluorescence properties of dissolved and colloidal organic matter, J. Geophys. Res.: Biogeosci., 2009, 114, G00F02 Search PubMed.
- D. C. Gooddy, D. J. Lapworth, M. J. Ascott, S. A. Bennett, T. H. Heaton and B. W. Surridge, Isotopic fingerprint for phosphorus in drinking water supplies, Environ. Sci. Technol., 2015, 49, 9020–9028 CrossRef CAS PubMed.
-
UK Small Area Health Statistics Unit, Environment and Health Atlas, http://www.envhealthatlas.co.uk, accessed 24 November 2016 Search PubMed.
- B. Flem, C. Reimann, K. Fabian, M. Birke, P. Filzmoser and D. Banks, Graphical statistics to explore the natural and anthropogenic processes influencing the inorganic quality of drinking water, ground water and surface water, Appl. Geochem., 2018, 88, 133–148 CrossRef CAS.
-
European Commision, Synthesis Report on the Quality of Drinking Water in the Member States of the European Union in the Period 1993–1995, European Commission, Brussels, 1996 Search PubMed.
-
United States Environmental Protection Agency, Enforcement and Compliance History Online (ECHO), https://echo.epa.gov/, (accessed 24 November 2016) Search PubMed.
-
United States Environmental Protection Agency, Analyse Trends: Drinking Water Dashboard, https://echo.epa.gov/trends/comparative-maps-dashboards/drinking-water-dashboard, (accessed 24 November 2016) Search PubMed.
-
Ministry of Social Affairs and Health, Qualité de l’eau potable, http://social-sante.gouv.fr/sante-et-environnement/eaux/article/qualite-de-l-eau-potable Search PubMed.
-
Global Water Intelligence, Water Market, USA, 2016 Search PubMed.
-
Deloitte, Water Country Profiles, Deloitte Touche Tohmatsu Ltd, London, 2014 Search PubMed.
Footnote |
† Electronic supplementary information (ESI) available. See DOI: 10.1039/c8em00437d |
|
This journal is © The Royal Society of Chemistry 2019 |