DOI:
10.1039/C9DT03044A
(Paper)
Dalton Trans., 2019,
48, 15127-15135
A new set of metal–organic frameworks synthesised from diisophthalate-based, 2′-phosphorus-substituted m-terphenyl linker molecules†
Received
25th July 2019
, Accepted 23rd September 2019
First published on 23rd September 2019
Abstract
Four metal–organic frameworks employing the m-terphenyl diisophthalate linker molecule with 2′ substitution by P(V)-based functional groups of the central aryl have been synthesised. The dense packing of POMe2/PSMe2 functional groups within UHM-60/UHM-61 (UHM: University of Hamburg Materials) with an underlying net of ucp topology was overcome by increasing the sterical demand of phosphorus substituents. Replacement of the PEMe2 (E = O, S) functional groups by POEt2 or POPh2 gave UHM-62 and UHM-63, respectively, where valid deconstructions of the underlying topology to types 3,3,4,4T199, tim, and tst were found. The potential influence of the now-accessible phosphoryl functional group towards CO2 and CH4 adsorption as well as the selectivity towards CO2/CH4 separation was studied. Based on a comprehensive survey of literature-known Cu(II)-based MOFs with m-terphenyl-based linker molecules, we propose the deconstruction of inter-isophthalate plane angles to angular components of twist and fold allowing for the sophisticated classification of topologies that can be realised in Cu(II)-based MOFs using the m-terphenyl tetracarboxylate linker molecule.
Introduction
We are interested in the synthesis of metal–organic frameworks (MOFs) with organic linker molecules bearing phosphorus-based functional groups with special attention to (a) the relationship between functional groups and the topology of the underlying net of MOFs obtained and (b) chemical utilisation of the phosphorus chemical group by means of post-synthetic metalation and subsequent applications in catalysis. We decided on using the m-terphenyl structural motif as a linker backbone for the phosphorus-based functional groups attached to the C2′ position of the central aryl, see Fig. 1. The connectivity to the respective inorganic secondary building unit was intended to be realised via carboxylates provided by isophthalate functional groups of the parent bent diisophthalate.
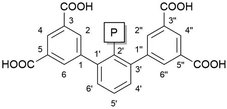 |
| Fig. 1 The diisophthalate-based m-terphenyl linker molecule backbone with a C2′-attached phosphorus functional group. The numbering system of the ligand backbone is given. | |
With special emphasis on P(III)-based phosphanes, phosphorus-based ligands are among the most commonly employed ligands in the fields of organometallic and coordination chemistry. Their coordinating properties towards Lewis acids can be fine-tuned over a broad range by means of well-established methods of synthetical chemistry, influencing both steric and electronic properties.1 Moreover, the transition to phosphane oxides and phosphane sulfides alters the Lewis basicity dramatically, rendering those reagents useful as solvating metal extractants in the area of hydrometallurgy.2 Bulky m-terphenyls form a rigid and concave backbone that has been employed to stabilise both 2′-attached phosphane ligands as well as derived transition metal complexes towards oxidation and dissociation reactions, respectively.3
The m-terphenyltetracarboxylate motif has been employed in the synthesis of a large number of MOFs, the majority of which were Cu(II)-based systems (see Table S4†) like the well-known PCN-305
4/NJU-Bai10
5 or one of the numerous examples belonging to the ZJNU series.6 However, concerning the 2′ position of the linker molecule, only examples exhibiting aza-substitution7–9 or substitution by structurally simple substituents like amino10–13 or methyl groups6,14 have come to our attention.
Some P(V)-based frameworks reported to date show exceptional CO2 uptakes,15 while the variety of linker molecules employed was, apart from only few notable exceptions,16,17 limited to the tri(4-carboxyphenyl)phosphane oxide linker molecule or its elongated analogues.18–20 The P
O functional group was shown to coordinate to the secondary building unit or metal ion via oxygen especially in cases where harder metal ions were employed.21–23 In case of the medium-hard Cu(II) ion, only two MOFs with the P
O oxygen atom being coordinated24 and uncoordinated,25 respectively, were reported.
We have synthesised a series of P(V)-based phosphane oxide and phosphane sulfide linker molecules. We felt that substitution by sterically demanding P-based functional groups at the 2′ position might enable for the synthesis of structurally and topologically novel Cu(II) MOFs and intended to investigate whether the combination of open metal sites26 and polar phosphane oxide functional groups might be beneficial in terms of CO2 adsorption properties. Installing the phosphane oxide functional group within the sterically restricted environment of the m-terphenyl backbone was expected to leave the P
O oxygen atom uncoordinated after the synthesis of the MOF. Fig. 2 gives an overview of the linker molecules and derived MOFs described herein.
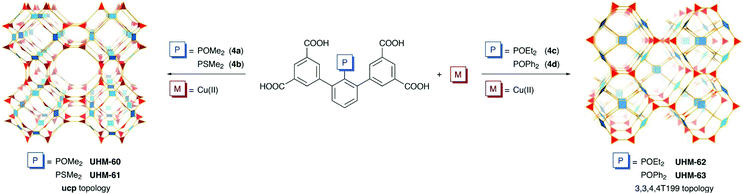 |
| Fig. 2 The 2′-phosphorus substitutional motifs of the parent m-terphenyl tetracarboxylic acid linker molecules along with the metal ions used for the synthesis of the UHM MOFs presented within this work. The MOFs are represented by the respective augmented versions of their underlying nets. Only one possibility of the underlying net of UHM-62 and UHM-63 is shown. | |
Experimental
For synthetical details, analytical methods, and in silico methods, please refer to ESI.† Details on single crystal X-ray structure analyses on MOFs and linker molecule precursor compounds as well as full information on literature surveys regarding MOFs featuring the m-terphenyl structural motif are also given therein.
UHM-60
In a rubber-sealed screw vial, the linker molecule 4a (66 mg, 0.14 mmol) and Cu(NO3)·2.5 H2O (132 mg, 568 μmol) were dissolved in N,N-dimethylacetamide (DMA; 14 mL). An aqueous solution of HBF4 (48 wt%, 0.17 mL, 1.3 mmol) was added and the solution was placed in an oven at 75 °C for 5 d. The solution was filtered using a syringe filter, placed in a Schott bottle, and further reacted at 75 °C. After additional 2 d, a light blue powder had separated from the reaction solution. The liquid was removed via syringe, the solid added with fresh DMA (10 mL) and the mixture left standing for three hours before the liquid was again removed via syringe. After drying at 60 °C for 18 h, 110 mg of the as-synthesised sample were obtained.
UHM-61
In a rubber-sealed screw vial, the linker molecule 4b (192 mg, 386 μmol) was dissolved in N,N-dimethylformamide (DMF; 10.6 mL). A solution of Cu(NO3)2·3 H2O (100 mg mL−1 in demineralised water; 3.88 mL, 388 mg, 1.61 mmol, 4 eq.) and nitric acid (w = 20%; 0.86 mL, 3.0 mmol, 8 eq.) were added. The mixture was heated at 50 °C for 4 d. The mixture was cooled to room temperature, and the light blue solid isolated via filtration. The solid was washed with DMF (5 mL) and dried at 60 °C for 18 h.
UHM-62
In a crimp vial, the linker molecule 4c (10 mg, 20 μmol) and Cu(NO3)2·2.5 H2O (10 mg, 43 μmol, 2.2 eq.) were dissolved in a mixture of DMA (1.1 mL) and water (0.5 mL). 0.02 mL of hydrochloric acid (w = 32%) were added. The vial was sealed, and the mixture reacted at 85 °C for 4 d. Dark blue crystals suitable for X-ray structure determination were obtained. After a single crystal had been picked, the remaining crystals were isolated via filtration, repeatedly washed with small volumes of DMA (totalling 5 mL), and dried at 60 °C for 2 d. 15 mg of blue crystals were obtained. For characterisation purposes, the synthesis was scaled up to 200 mg of linker molecule. In a typical reaction, 200 mg of linker molecule (392 μmol), 191 mg of Cu(NO3)2·2.5 H2O (821 μmol, 2.1 eq.), 13 mL DMA, 8 mL H2O, and 0.40 mL hydrochloric acid (w = 32%) were used. After 3 d, 203 mg of a polycrystalline light blue solid were obtained and washed/dried as described above.
UHM-63
In modification of the procedure described for UHM-62, the linker molecule 4d (10 mg, 16 μmol) and Cu(NO3)2·2.5 H2O (10 mg, 43 μmol, 2.7 eq.) were reacted in a 1
:
1 mixture of DMA and water (total volume of 0.80 mL). 13 mg of single crystals suitable for X-ray structure determination were obtained after 3 d. The synthesis was scaled up to use 201 mg of linker molecule (398 μmol), 166 mg of Cu(NO3)2·2.5 H2O (714 μmol), 13 mL DMA, 8 mL H2O, and 0.40 mL hydrochloric acid (w = 32%). After 4 d, 239 mg of a polycrystalline light blue solid were obtained.
Results and discussion
Characterisation of UHM-60
The structure of UHM-60 with a composition of Cu2L reflecting a neutral framework was elucidated using homology modelling on the UHM-25
27 structure (see ESI† for details). When disregarding the phosphorus functional group, UHM-60 can be described in the cubic space group Pm
m with a = 25.93 Å. The activation procedure of sequential solvent exchange and final thermal activation in vacuo gave a permanently porous material as proven by nitrogen physisorption at 77 K and the retention of characteristic reflections in powder X-ray diffraction (pXRD) at the desolvated state. A type I(b) isotherm indicative of a microporous adsorbent featuring wider micropores was obtained, and a specific BET surface area of SBET = 1939 m2 g−1 as well as a total pore volume of 0.89 cm3 g−1 were derived. Based on a structural model obtained from homology modelling, a calculation using the Poreblazer software28 suggests a void volume of 76% and an accessible specific surface area of 1950 m2 g−1. TG-DTA/MS measurements of as-synthesised and activated materials have proven the near-quantitative removal of solvent guest molecules and a thermal stability of UHM-60 up to approximately 220 °C.
The residue of thermal analysis was characterised as Cu4O(PO4)2 from X-ray powder diffraction. The linker integrity was proven by NMR spectroscopy on the reisolated linker molecule from acidic MOF digestion.
Characterisation of UHM-61
UHM-61 is isostructural to UHM-60 as shown by pXRD (a = 25.96 Å). The cubic symmetry is reflected by the morphology of the material as revealed from scanning electron microscopy showing the presence of cubes with an edge length of approximately 25 μm. The linker identity was examined via1H and 31P{1H} NMR spectroscopy on an acid-digested sample of UHM-61. We found a mixture of phosphane sulfide linker molecule and phosphane oxide linker molecule in a ratio of approximately 2
:
1, proving that some oxidation of the P
S functional group took place, presumably during the synthesis of the MOF. Physisorption experiments employing N2 at 77 K and Ar at 87 K gave type I(b) isotherms and let us derive specific surface areas of SBET = 1930 m2 g−1 and 1772 m2 g−1, respectively. The total pore volume was determined to be 0.78 cm3 g−1 from N2 physisorption. A Poreblazer calculation suggests a void volume of 76% and an accessible specific surface are of 1879 m2 g−1 for UHM-61. TG-DTA/MS in an oxidising atmosphere verified the release of SO2 during the combustion of the material starting from 220 °C, demonstrating the incorporation of the sulfur-containing functional group into the material. To the best of our knowledge, UHM-61 is the first MOF employing a linker molecule featuring the phosphane sulfide functional group.
Structural description of UHM-60/UHM-61
Fig. 3 shows the relevant structural representations of UHM-60 and UHM-61. The structure shown in Fig. 3a is best understood when pore-discriminating polyhedra with respective vertices provided by Cu(II) paddle-wheels are identified. Cuboctahedra (Fig. 3b; orange colour) with an edge length of 9.2 Å are interconnected along the spatial dimensions via four linker molecules, respectively (Fig. 3c; green colour). Those bridges form a square cuboid of dimensions 9.2 Å × 9.2 Å × 12.9 Å. The PEMe2 (E = O, S) functional groups exclusively extending into this pore type show a very dense packing, presumably exhibiting non-classical hydrogen bonding features of type P
E⋯H–CH2–P. Another pore type described by a rhombicuboctahedron (Fig. 3d; red colour) is framed by orange- and green-type pores to construct a cube with cuboctahedra placed at the eight vertices and the bridges as edges of the cube. The faces of the cube represent pore windows (large blue sphere, Fig. 3a) connecting neighbouring red-type pores.
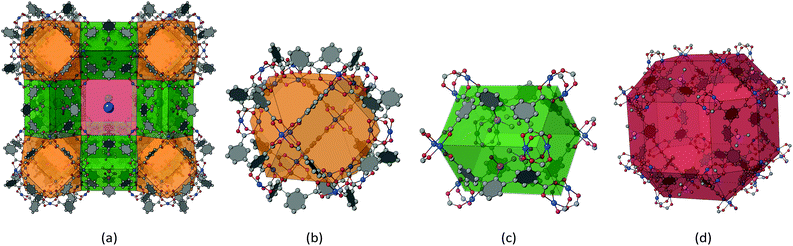 |
| Fig. 3 Structural representations of UHM-60 (UHM-61 is isostructural). (a) View onto the ab plane with pore-discriminating polyhedra using Cu(II) paddle-wheels as vertices. Large blue spheres indicate pore windows between two neighbouring red-type pores. (b) The orange-type pores can be described by a cuboctahedron. (c) Green-type pores are best described by a square cuboid. (d) Red-type pores are represented by a rhombicuboctahedron. Guest molecules, axially coordinated ligands of Cu(II) paddle-wheels, and H atoms omitted for clarity. Fractional coordinates of centroids of polyhedra and the large blue sphere in space group Pm m: orange (0.5,0.5,0.5), green (0.5,0.5,0), red (0,0,0), blue (0.5,0,0). Aryls are shown as face-filled disks. Grey: C, red: O, purple: P, blue: Cu. | |
Within UHM-60/UHM-61 as well as an isoreticular Cu(II)-based MOF by Zaworotko and co-workers29 and the UHM-25 class materials reported by our group,27 the tip of the ‘V’ of the V-shaped linker extends into the red-type pores, thus being averted from the volume of green-type pores. In contrast to UHM-60/UHM-61, where the P-based functional groups extend into the green-type pores, the UHM-25-class MOFs show substitution with chiral pool-derived functional groups expanding into the readily accessible red-type pores.
Topology
The underlying net of UHM-60 and UHM-61 can be described as binodal (3,4)-c ucp with minimal transitivity30pqrs = 2244. If the linker molecule is recognised as a single 4-c node, the edge-transitive and uninodal 4-c basic net rhr with pqrs = 1132 is obtained. The molecular (non-periodic) relative to the cuboctahedron shown in Fig. 3b, MOP-1, which is constructed from isophthalate ligands coordinated to Cu(II) paddle-wheels, was already presented in 2002.31 O'Keeffe and Yaghi proposed different ways to interconnect cuboctahedra, depending on whether neighbouring cuboctahedra point at each other in a face-to-face (A), vertex-to-vertex (B), or vertex-to-face fashion (C).32 Mixed combinations are realised in zmj (A and B) and zhc (A and C) topologies, respectively, while the exclusive presence of the simplest connection of type (A) is described in ucp topology. We discussed the implications of linker conformation on the resulting type of interconnectivity of neighbouring cuboctahedra earlier27 and will expand this approach later herein.
UHM-62 and UHM-63
Characterisation of UHM-62 and UHM-63.
The isostructural MOFs UHM-62 and UHM-63 crystallise in the orthorhombic space group Immm. Reisolation and NMR spectroscopic characterisation of the respective linker molecules after acidic digestion of the MOFs gave no indication of decomposition. TG-DTA/MS measurements show that the materials are stable up to approximately 250 °C and prove the successful removal of guest molecules. N2 physisorption at 77 K gave type I(b) isotherms in both cases, with BET surface areas of 1429 m2 g−1 and 1358 m2 g−1 and total pore volumes of 0.68 cm3 g−1 and 0.62 cm3 g−1 for UHM-62 and UHM-63, respectively. The Ar physisorption isotherm at 87 K for UHM-62 also shows type I(b) behaviour and gave a specific surface area of SBET = 1457 m2 g−1. A void volume of 73% (74%) and a specific surface area of 1337 m2 g−1 (1199 m2 g−1) was calculated using Poreblazer for UHM-62 (UHM-63). For both materials, characteristic reflections are retained at the desolvated state as shown by pXRD, underlining the retention of structural integrity. When disregarding axially coordinated Cu(II) ligands, both materials are electrostatically neutral compounds with a general composition of Cu2L.
Structural description of UHM-62/UHM-63.
The structure shall be described in terms of the different pore types identified and visualised in Fig. 4c–f. With respect to the centroids of limiting Cu(II) paddle-wheels, the principal geometry of pores depicted in Fig. 4c–e can be described by rectangular cuboids of dimensions 16.7 Å × 12.9 Å × 9.2 Å. The longer two of the respective edges represent the interconnection of paddle-wheels across the linker molecule, while the distance of 9.2 Å corresponds to a linkage by an isophthalate entity. The orange-type pore (Fig. 4c) shows additional symmetrical attachment of two edges to opposing faces of 16.7 Å × 12.9 Å with an edge-to-edge distance of 18.3 Å. Pore types depicted in green (Fig. 4d) and yellow colour (Fig. 4e) contain no phosphorus-based functional groups. The yellow-type pore shows additional capping by two vertices attached to opposing faces of 12.9 Å × 9.2 Å with a vertex-to-vertex distance of 26.0 Å. The pore type indicated by a large blue sphere (Fig. 4f) is framed by four linker molecules with phosphane oxide oxygen atoms at distances of 3.7 Å and 7.8 Å, respectively. Remarkably short Cu⋯Cu inter-paddle-wheel distances of 4.8227(13) Å (UHM-62) and 4.789(3) Å (UHM-63), respectively, have been found within the materials. In UHM-62, the interstitial space was found to be occupied by a μ2-Cl− ion. Within UHM-63, a mixed occupation by Cl− and an oxygen-type ligand, presumably water, with an occupancy of 0.53(3) for Cl− was found. In both cases, charge-neutralising dimethylammonium cations were located from the difference Fourier map. To date, no attempts were made to exchange the O/Cl−-type interstitial sites against each other or for related chemical species or to assess the potential removal of the bridging ligands. The highly polar environment characterised by electropositive Cu(II) ions and the negatively polarised phosphane oxide oxygen atoms might render this unique chemical environment interesting in applications such as gas storage or separation or studies concerning the magnetic interaction between inter-paddle-wheel Cu(II) ions.
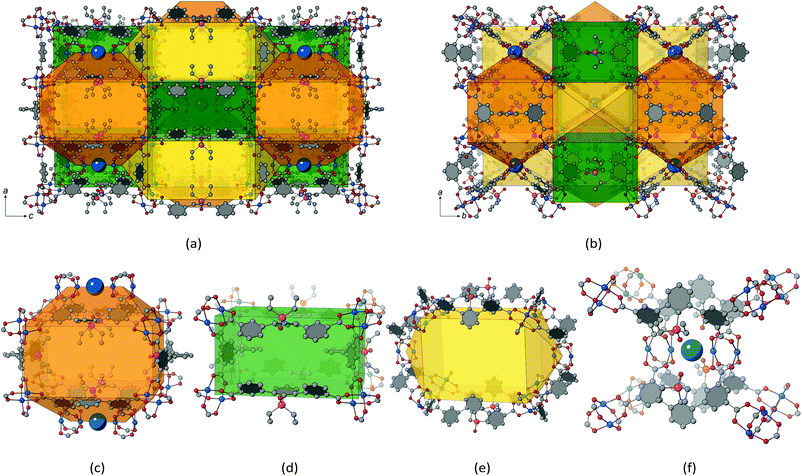 |
| Fig. 4 Structure of UHM-62 (UHM-63 is isostructural) with pore-discriminating coordination polyhedra and large blue spheres with adjacent Cu(II) paddle-wheels as vertices (a) looking onto the ac plane and (b) onto the ab plane. (c) Orange-type pores are described by a rectangular cuboid with two edges added to opposing faces, (d) green-type pores by a rectangular cuboid. (e) Pores indicated by yellow colour can be described by an elongated square bipyramid. (f) A blue sphere indicates interstitial space between two neighbouring Cu(II) paddle-wheels which are interconnected by four linker molecules. An idealised activated state of the material omitting H atoms, guest molecules, and Cu-coordinated moieties is shown. Fractional coordinates of the polyhedral centroids and the blue sphere within the space group Immm: blue (0,0,0), green (0.5,0,0.5), yellow (0,0,0.5), orange (0,0.5,0.5). m-Terphenyl backbone aryls shown as grey disks. Grey: C, red: O, purple: P, blue: Cu. | |
Shortest inter-paddle-wheel distances for MOFs based on the m-terphenyl tetracarboxylate linker molecule have been found for Zn(II)-based (Zn⋯Zn = 3.751(4) Å) and Mn(II)-based (Mn⋯Mn = 3.892(2) Å) materials.33 Herein, a 2′-aza-functionalized m-terphenyl diisophthalate linker molecule was used and the paddle-wheels were bridged by μ2-H2O ligands. In Zhou and co-workers’ PCN-88, which is isoreticular to UHM-62/UHM-63 (see section below) employing the elongated 2,7-bis(3′,5′-dicarboxyphenyl)naphthalene linker molecule, an inter-paddle-wheel Cu⋯Cu distance of 7.3655(12) Å was found.34 This cavity proved to be efficient as a single-molecule trap (SMT) for CO2, rendering the material promising in terms of CO2 capture or activation. Recently, the PCN-88 topology was first found within a Cu(II) m-terphenyl tetracarboxylate-based material BUT-301
35 by employing the 5′-NO2-substituted linker molecule with Cu⋯Cu = 4.7575(13) Å and a μ2-chloride ion. In addition, we are aware of only few examples with axially cross-linked Cu(II) paddle-wheels of 3-periodic MOFs where the link is provided by a chloride anion36 (Cu⋯Cu = 4.550(3) Å) or an oxygen-type ligand. Liu et al. found a water molecule cross-linking neighbouring Cu(II) paddle-wheels at a distance of 4.481(2) Å.37
Carbon dioxide and methane physisorption and selectivities
The chemical nature of the P
O bond is not sufficiently described in terms of a simple Lewis structure, as Gilheany pointed out in an influential review.38 In order to assess the influence of the accessible and polar phosphoryl functional group, we evaluated UHM-62 as a model compound for the study of adsorption and separation of carbon dioxide and methane, with the former having a large quadrupolar moment. The CO2 and CH4 adsorptions at 273 K and 298 K for UHM-62 are shown in Fig. 5a.
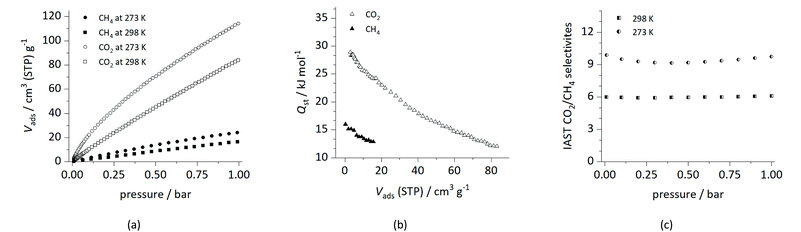 |
| Fig. 5 (a) Adsorption branches of CO2 and CH4 physisorption experiments on UHM-62 at temperatures of 273 K and 298 K, respectively. (b) Isosteric heats of adsorption Qst for CO2 and CH4, respectively. (c) IAST selectivity plot for a binary CO2/CH4 = 50/50 mixture at 273 K and 298 K, respectively. | |
CO2 uptakes of 84.0 cm3 (STP) g−1/3.72 mmol g−1 (298 K) and 114.2 cm3 (STP) g−1/5.06 mmol g−1 (273 K) were determined, whereas 16.4 cm3 g−1/0.722 mmol g−1 and 24.0 cm3 g−1/1.06 mmol g−1 of CH4 are adsorbed at 298 K and 273 K, respectively. Isosteric heats of adsorption (Qst) at low coverage were determined to be 28.9 kJ mol−1 and 16.0 kJ mol−1 for CO2 and CH4, respectively (Fig. 5b).
A comparison with literature-known Cu(II)-based MOFs based on the m-terphenyl linker molecule reveals that CO2 capacities up to 122.6 cm3 g−1 at 298 K (ref. 39) and Qst values up to 39.5 kJ mol−1 (ref. 40) with median values of 103 cm3 g−1 and 24.6 kJ mol−1, respectively, were found. While the Qst value found for UHM-62 lies in the upper quintile, the total CO2 uptake is the second lowest of the values reported in literature. The highest adsorption enthalpies have been reported for materials with 5′-F-2′-NH2 (39.5 kJ mol−1),40 2′-NH2 (37.51 kJ mol−1),11 and 2′-aza-functionalized m-terphenyls (36.9 kJ mol−1),9 indicating a strong interaction between the nitrogen-based functional groups and CO2. A notable exception is found with ZJNU-54 with a 2′-NH2-4′,6′-diaza substitutional pattern, where a moderate value of 24.7 kJ mol−1 was found. However, substitution with nitrogen-based functional groups at positions other than 2′ generally gave rather medium Qst values between 20.7 kJ mol−1 and 26.72 kJ mol−1.4,39,41–44 For electrostatically neutral MOFs featuring unsubstituted and alkyl-substituted m-terphenyl linker molecules, Qst values between 22.5 kJ mol−1 and 24.8 kJ mol−1 were found.4,6,14 For PCN-88, Qst = 27 kJ mol−1 and a capacity of 94 cm3 g−1 (296 K) were reported.34 Taken all together, there is indication for an increased interaction between the P
O functional group and CO2 as indicated by an increased Qst value compared to unfunctionalized materials, but the total CO2 uptake was found to be comparably low. This might be attributed to reduced surface area and pore volume, which are necessary prerequisites for significant CO2 capacities.
The ideal adsorbed solution theory (IAST)45 was used to calculate the CO2/CH4 selectivities of UHM-62 at 298 K and 273 K, respectively (Fig. 5c). Selectivities of 6.1 (298 K) and 9.7 (273 K) at 1 bar were obtained. Within the group of Cu(II)-based m-terphenyl MOFs, best selectivities were determined for anionic JLU-Liu22
46 (9.4 at 298 K) and a cationic MOF9 (30.8 at 298 K). Within the subset of neutral MOFs, selectivities between 4.95 and 7.14 at 298 K were reported; UHM-62 is thus expected to show only modest selectivities in CO2/CH4 separation processes according to the IAST model. For a detailed tabulation of literature-available data regarding CO2/CH4 capacities and selectivities, see Tables S6 and S7.†
Topology of UHM-62 and UHM-63.
UHM-62 and UHM-63 can be deconstructed in several ways shown in Fig. 6a–c in terms of their augmented nets. The Cl− ion (UHM-62) as well as the site of mixed occupancy of Cl− and an oxygen atom species (UHM-63) can (a) be omitted during the deconstruction procedure, (b) be regarded as a link between two 4-c nodes, rendering those to become a set of two interconnected 5-c nodes, or (c) be taken as a chemical conjunction between the paddle-wheels, thus contracting two 4-c nodes to a single 8-c node. The corresponding nets in terms of TOPOS symbols or, if available, RCSR symbols (three-letter codes set in bold lettering), can be identified as:
(a) 3,3,4,4T199: a (3,3,4,4)-c net with pqrs = 4564
(b) tim: a (3,3,4,5)-c net with pqrs = 4653
(c) tst: a (3,3,4,8)-c net with pqrs = 4553
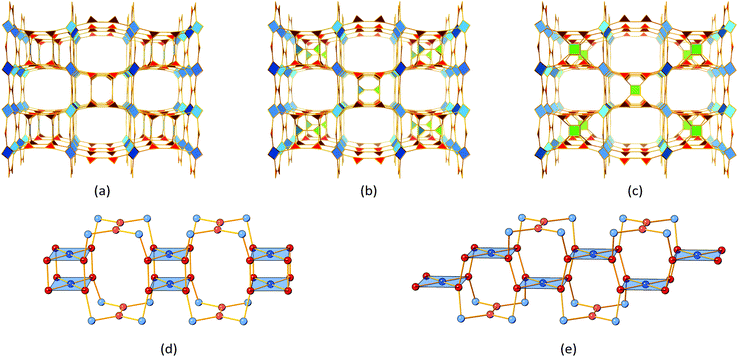 |
| Fig. 6 (a–c) show possible representations of underlying augmented nets of UHM-62 and UHM-63 according to the possibilities proposed in the text where 3-c nodes are shown in red and 4-c nodes are shown in blue colour. Symmetry-inequivalent 3-c and 4-c nodes are not further distinguished by colour. (a) Augmented version of 3,3,4,4T199. (b) tim-a features sets of interconnected 5-c nodes depicted as green-coloured square pyramids in a (3,3,4,5)-c underlying net. (c) In tst-a, 8-c nodes represented by green cubes of a (3,3,4,8)-c net are present. (d) and (e) compare the nets (d) 3,3,4,4T199 and (e) mfj where topologically inequivalent 3-c and 4-c notes are distinguished by different shades of red and blue colour, respectively. Dark blue 4-c nodes have been added with a translucent square with neighbouring 3-c nodes as edges. It becomes obvious that 3,3,4,4T199 shows a stacked fashion of the 4-c nodes in question, while for mfj a stepped sequence is observed. | |
It is noteworthy to mention that the nets of 3,3,4,4T199 and the commonly encountered mfj are closely related to each other as can be seen from the comparison made in Fig. 6d and e. Both nets are (3,3,4,4)-c and derived from two different (4,4,4,4)-c nets, however, the spatial arrangement of the 4-c nodes depicted in dark blue colour in Fig. 6d–e differs significantly. A stacked orientation is observed for the former, while for mfj a parallel-displaced alignment is found.
Conformations of m-terphenyl diisophthalates and topology of derived Cu(II)-based MOFs
An evaluation of literature-known three-periodic MOFs employing the m-terphenyl diisophthalate linker molecule and its substituted derivatives (Table S4†) has shown that there is, apart from only relatively few exceptions that came to our attention,8,9,35,47–49 a strong preference to form structures with underlying mfj or sty topologies, with the first examples reported in literature being the PCN-305 to PCN-308 series4 for mfj and a material that has been prepared by post-synthetic metal ion exchange of Zn(II) for Cu(II) for sty.11 The replacement of the phosphorus-attached methyl groups (UHM-60/UHM-61) for ethyl (UHM-62) and phenyl groups (UHM-63) was expected to inevitably implicate a change in topology. It was our intention to increase the steric demands of the 2′ substituent to render the close packing of the P-attached functional groups within the bridges described in ucp topology (see Fig. 3c) less likely. Nevertheless, the fact that a new topology (ucp) and one that has only been realised once so far (3,3,4,4T199) for MOFs belonging to the well-established class of Cu(II) m-terphenyl diisophthalate MOFs, let us assume that there is an additional structure–topology relationship to be considered.
We tried to assess and classify the conformations of m-terphenyl diisophthalate linker molecules in order to derive implications on resulting topologies. Therefore, we determined the fold and twist angles between the least-squares mean planes (LSMPs) of isophthalates belonging to the same linker molecule. In comparison to an ordinary interplanar angle (plane-to-plane angle), its deconstruction into components of twist and fold is useful for an interpretation in terms of chemical meaningfulness. The twist angle is a measure for the torsion of the isophthalates along the centroid–centroid connecting line, while the fold angle quantifies the opening angle of the isophthalates with respect to their connecting chemical entity, e.g. an aryl for m-terphenyl-type linker molecules. Fig. 7a and b show folded and twisted conformations of a m-terphenyl linker molecule, respectively. For a graphical description of twist and fold angles, see ESI.† LSMPs as well as the relevant angles can conveniently be examined using the OLEX2 software.50
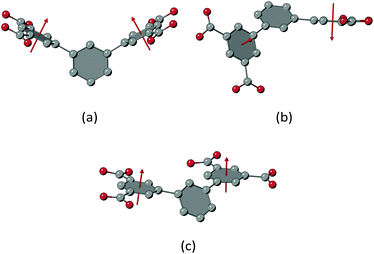 |
| Fig. 7 (a) Folded, (b) twisted, and (c) coplanar conformations of the m-terphenyl diisophthalate linker molecule. Aryls are shown as grey disks, H atoms have been omitted for clarity. The plane normals of the diisophthalates are shown as red arrows. | |
The results of our survey are visualised in Fig. 8. It can be recognised that two linker conformations are present within mfj topology. One conformer shows twist angles between 72°–75° and a fold component of 28°–34° and is thus referred to as twisted conformer. A second, folded conformer shows twist angles of 0° and fold angles of 54°–66°. It is noteworthy that isophthalates related by mirror-symmetry cannot exhibit twist angles other than 0°. Within the MOFs with underlying sty topology, only the folded conformation of the linker molecule with twist angles being close to 0° and a fold component between 60°–71° is observed. For the UHM series materials UHM-60 to UHM-63, twisting of the isophthalates is suppressed by the presence of sterically demanding tetrahedral organophosphorus functional groups attached to C2′. For UHM-60/UHM-61 with underlying ucp topology, a folded conformation of the peripheral aryls with a fold angle of 51° is required. The fold angle for the linker conformation within UHM-60/UHM-61 is slightly lower than that within folded conformations within for mfj (54°–66°) and sty (60°–71°) topologies.
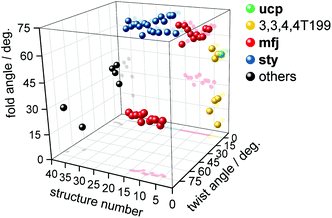 |
| Fig. 8 Evaluation of fold and twist angles of isophthalates belonging to the same linker molecule within Cu(II) MOFs employing the m-terphenyl diisophthalate linker molecule and its substituted derivatives for literature-known examples as well as for UHM-60/UHM-61 (structure number 1), UHM-62 (2), and UHM-63 (3). The different sphere colours represent ucp (green), 3,3,4,4T199 (yellow), mfj (red), sty (blue), or other (black) topologies. MOFs belonging to the structure numbers referred herein and corresponding angles are listed in Table S5.† | |
Also, for UHM-62 and UHM-63 with underlying 3,3,4,4T199 topologies, folded conformations of the linker molecule are observed. The first one shows fold components of 48.0(4)° and 50.8(3)° (for UHM-62 and UHM-63, respectively), thus lying in a range close to that of UHM-60/UHM-61. The second conformer exhibits fold angles of 7.8(16)°/24.1(15)° (two disordered sites within UHM-62) and 22.7(5)° (UHM-63), which results in the isophthalates showing an approximately coplanar orientation. These values are also confirmed by the isoreticular BUT-301, where fold angles of 53.7(2)° for the folded conformer and 16.96(19)° for the coplanar conformer are found. To the best of our knowledge, the coplanar conformation of peripheral aryls is first realised within m-terphenyl diisophthalate MOFs based on the 3,3,4,4T199 topology. Fig. 7c shows the coplanar conformation of the m-terphenyl diisophthalate linker molecule as discussed before. It is noteworthy that the transition from a folded conformation towards a coplanar conformation implies an increase in the metal-to-metal distances across the linker. For UHM-62, a distance between paddle-wheel centroids of 12.9 Å was found for the folded conformation, while the distance is increased to 16.7 Å within the coplanar conformation. It is obvious that the conformation of the m-terphenyl diisophthalates thus exerts direct and significant influence on the pore dimensions expected in a synthesised MOF.
For the examples of MOFs showing underlying nets not belonging to the four topologies discussed before, combinations of twist and fold contributions lying outside the ranges defined for mfj, sty, ucp, and 3,3,4,4T199 are observed, except for one example with an underlying topology characterised by a point symbol {62·82·122}{62·8}4{64·82} showing only a twisted conformation of the linker molecule.8 The relevant angles discussed before are summarised in Table 1.
Table 1 Topologies observed for Cu(II)-based m-terphenyl diisophthalate MOFs. Angular ranges have been rounded. For exact values and corresponding references, see Table S5†
Topology |
Conformer |
Twist angles/deg. |
Fold angles/deg. |
mfj
|
Twisted |
72–75 |
28–34 |
Folded |
0 |
54–66 |
sty
|
Folded |
0 |
57–71 |
ucp
|
Folded |
0 |
51 |
3,3,4,4T199 |
Folded |
0 |
48–54 |
Coplanar |
0–4 |
8–23 |
3,3,3,4,4T31 |
|
62 |
14 |
|
10 |
27 |
{6·8·9}4{6·84·11}{6·84·12} |
|
9 |
39 |
3,3,4T213 |
|
8 |
33 |
3,3,4,4T234 |
|
8 |
36 |
{62·82·122}{62·8}4{64·82} |
Twisted |
70 |
27 |
Conclusions
We successfully synthesised m-terphenyl diisophthalate linker molecules with C2′-organophosphorus substituents. The dimethylphosphoryl and dimethylphosphane sulfide linker molecules 4a and 4b, respectively, gave the isostructural Cu(II)-based UHM-60 and UHM-61 with the relatively rare underlying ucp topology where the latter represents the first example of a MOF incorporating phosphane sulfide functional groups. The non-accessible P
E (E = O, S) functional group was made available to the pore volume by replacement of the P-attached methyl groups by ethyl and phenyl groups to give the Cu(II)-based UHM-62 und UHM-63, respectively. These materials are examples of the rare underlying 3,3,4,4T199 topology and, in comparison to the isoreticular PCN-88, show a significant reduction of inter-paddle-wheel distances to 4.8227(13) Å and 4.789(3) Å, respectively. The chemical interconnection of the paddle-wheels allowed us to derive the topological types tim and tst. A study of CO2 and CH4 adsorption on UHM-62 revealed very low influence of the highly polar P
O functional group on CO2/CH4 selectivities according to the IAST model and adsorption enthalpies. However, a comprehensive study of Cu(II)-based MOFs employing the m-terphenyl diisophthalate linker molecule by deconstruction of interplanar angles to components of twist and fold allowed for the classification of linker conformations and might be useful in the systematic exploration of rare or previously unknown topologies by chemical restriction of conformational space.
Conflicts of interest
There are no conflicts to declare.
Notes and references
- C. A. Tolman, Chem. Rev., 1977, 77, 313–348 CrossRef CAS.
- A. M. Wilson, P. J. Bailey, P. A. Tasker, J. R. Turkington, R. A. Grant and J. B. Love, Chem. Soc. Rev., 2014, 43, 123–134 RSC.
- L. Ortega-Moreno, M. Fernández-Espada, J. J. Moreno, C. Navarro-Gilabert, J. Campos, S. Conejero, J. López-Serrano, C. Maya, R. Peloso and E. Carmona, Polyhedron, 2016, 116, 170–181 CrossRef CAS.
- Y. Liu, J.-R. Li, W. M. Verdegaal, T.-F. Liu and H.-C. Zhou, Chem. – Eur. J., 2013, 19, 5637–5643 CrossRef CAS PubMed.
- Z. Lu, L. Du, K. Tang and J. Bai, Cryst. Growth Des., 2013, 13, 2252–2255 CrossRef CAS.
- D.-L. Chen, Z. Tian, Y. He, M. He, L. Zhu, H. Zhong, X. Zhang, Y. Wang and Y. Zhang, Dalton Trans., 2018, 47, 2444–2452 RSC.
- L. Fan, W. Fan, B. Li, X. Liu, X. Zhao and X. Zhang, CrystEngComm, 2015, 17, 4669–4679 RSC.
- J. Liu, W. Wang, Z. Luo, B. Li and D. Yuan, Inorg. Chem., 2017, 56, 10215–10219 CrossRef CAS PubMed.
- B. Liu, H.-F. Zhou, L. Hou, Z. Zhu and Y.-Y. Wang, Inorg. Chem. Front., 2016, 3, 1326–1331 RSC.
- J. Jiao, L. Dou, H. Liu, F. Chen, D. Bai, Y. Feng, S. Xiong, D.-L. Chen and Y. He, Dalton Trans., 2016, 45, 13373–13382 RSC.
- T. K. Pal, D. De, S. Neogi, P. Pachfule, S. Senthilkumar, Q. Xu and P. K. Bharadwaj, Chem. – Eur. J., 2015, 21, 19064–19070 CrossRef CAS PubMed.
- M. Gupta, D. De, K. Tomar and P. K. Bharadwaj, Inorg. Chim. Acta, 2018, 482, 925–934 CrossRef CAS.
- A. K. Gupta, D. De, K. Tomar and P. K. Bharadwaj, Dalton Trans., 2018, 47, 1624–1634 RSC.
- Y. He, X. Gao, Y. Wang, M. He and S. Li, Dalton Trans., 2018, 47, 8983–8991 RSC.
-
J. E. Reynolds III, S. G. Dunning, C. M. McCulley and S. M. Humphrey, in Elaboration and Applications of Metal-Organic Frameworks, ed. S. Ma and J. A. Perman, World Scientific Publishing Co. Pte. Ltd., Singapore, Shengqian, 2018, pp. 37–142 Search PubMed.
- Q.-D. Shu, S.-N. Kong, Y.-Z. Wei and M.-H. Shu, Polyhedron, 2016, 118, 96–102 CrossRef CAS.
- J. Feng, H. Li, Q. Yang, S.-C. Wei, J. Zhang and C.-Y. Su, Inorg. Chem. Front., 2015, 2, 388–394 RSC.
- Y.-Y. Yang, Z.-J. Lin, J. Liang, Y. Huang and R. Cao, CrystEngComm, 2015, 17, 4547–4553 RSC.
- Y. Sun, X. Chen, F. Wang, R. Ma, X. Guo, S. Sun, H. Guo and E. V. Alexandrov, Dalton Trans., 2019, 48, 5450–5458 RSC.
- H. Guo, Y. Sun, F. Zhang, R. Ma, F. Wang, S. Sun, X. Guo, S. Liu and T. Zhou, Inorg. Chem. Commun., 2019, 107, 107492 CrossRef CAS.
- A. M. Bohnsack, I. A. Ibarra, P. W. Hatfield, W. J. Yoon, Y. K. Hwang, J.-S. Chang and S. M. Humphrey, Chem. Commun., 2011, 47, 4899–4901 RSC.
- X. Du, R. Fan, L. Qiang, K. Xing, H. Ye, X. Ran, Y. Song, P. Wang and Y. Yang, ACS Appl. Mater. Interfaces, 2017, 9, 28939–28948 CrossRef CAS PubMed.
- Y. Li, Z. Weng, Y. Wang, L. Chen, D. Sheng, Y. Liu, J. Diwu, Z. Chai, T. E. Albrecht-Schmitt and S. Wang, Dalton Trans., 2015, 44, 20867–20873 RSC.
- W. R. Lee, D. W. Ryu, W. J. Phang, J. H. Park and C. S. Hong, Chem. Commun., 2012, 48, 10847–10849 RSC.
- L. Huo, J. Zhang, L. Gao, X. Wang, L. Fan, K. Fang and T. Hu, J. Solid State Chem., 2017, 256, 168–175 CrossRef CAS.
- R. Poloni, K. Lee, R. F. Berger, B. Smit and J. B. Neaton, J. Phys. Chem. Lett., 2014, 5, 861–865 CrossRef CAS PubMed.
- M. Sartor, T. Stein, F. Hoffmann and M. Fröba, Chem. Mater., 2016, 28, 519–528 CrossRef CAS.
- L. Sarkisov and A. Harrison, Mol. Simul., 2011, 37, 1248–1257 CrossRef CAS.
- C. Li, W. Qiu, W. Shi, H. Song, G. Bai, H. He, J. Li and M. J. Zaworotko, CrystEngComm, 2012, 14, 1929 RSC.
- M. Li, D. Li, M. O'Keeffe and O. M. Yaghi, Chem. Rev., 2014, 114, 1343–1370 CrossRef CAS PubMed.
- M. Eddaoudi, J. Kim, J. B. Wachter, H. K. Chae, M. O'Keeffe and O. M. Yaghi, J. Am. Chem. Soc., 2001, 123, 4368–4369 CrossRef CAS PubMed.
- M. O'Keeffe and O. M. Yaghi, Chem. Rev., 2012, 112, 675–702 CrossRef PubMed.
- B. Liu, W.-P. Wu, L. Hou, Z.-S. Li and Y.-Y. Wang, Inorg. Chem., 2015, 54, 8937–8942 CrossRef CAS PubMed.
- J.-R. Li, J. Yu, W. Lu, L.-B. Sun, J. Sculley, P. B. Balbuena and H.-C. Zhou, Nat. Commun., 2013, 4, 1538 CrossRef PubMed.
- M.-M. Xu, X.-J. Kong, T. He, X.-Q. Wu, L.-H. Xie and J.-R. Li, Dalton Trans., 2019, 48, 9225–9233 RSC.
- F. Gao, Y. Li, Y. Ye, L. Zhao, L. Chen and H. Lv, Inorg. Chem. Commun., 2017, 80, 72–74 CrossRef CAS.
- J. Liu, G. Liu, C. Gu, W. Liu, J. Xu, B. Li and W. Wang, J. Mater. Chem. A, 2016, 4, 11630–11634 RSC.
- D. G. Gilheany, Chem. Rev., 1994, 94, 1339–1374 CrossRef CAS PubMed.
- D. Bai, X. Gao, M. He, Y. Wang and Y. He, Inorg. Chem. Front., 2018, 5, 1423–1431 RSC.
- T. K. Pal, D. De, S. Senthilkumar, S. Neogi and P. K. Bharadwaj, Inorg. Chem., 2016, 55, 7835–7842 CrossRef CAS PubMed.
- Z. Lu, Y. Xing, L. Du, H. He, J. Zhang and C. Hang, RSC Adv., 2017, 7, 47219–47224 RSC.
- Z. Lu, F. Meng, L. Du, W. Jiang, H. Cao, J. Duan, H. Huang and H. He, Inorg. Chem., 2018, 57, 14018–14022 CrossRef CAS PubMed.
- J. Jiao, D. Jiang, F. Chen, D. Bai and Y. He, Dalton Trans., 2017, 46, 7813–7820 RSC.
- L. Zhang, K. Jiang, Y. Li, D. Zhao, Y. Yang, Y. Cui, B. Chen and G. Qian, Cryst. Growth Des., 2017, 17, 2319–2322 CrossRef CAS.
- A. L. Myers and J. M. Prausnitz, AIChE J., 1965, 11, 121–127 CrossRef CAS.
- D. Wang, B. Liu, S. Yao, T. Wang, G. Li, Q. Huo and Y. Liu, Chem. Commun., 2015, 4, 1166–1169 Search PubMed.
- D. Wang, B. Liu, S. Yao, T. Wang, G. Li, Q. Huo and Y. Liu, Chem. Commun., 2015, 51, 15287–15289 RSC.
- Y. Pan, W. Liu, D. Liu, Q. Ding, J. Liu, H. Xu, M. Trivedi and A. Kumar, Inorg. Chem. Commun., 2019, 100, 92–96 CrossRef CAS.
- J. Jiao, H. Liu, F. Chen, D. Bai, S. Xiong and Y. He, Inorg. Chem. Front., 2016, 1411–1418 RSC.
- O. V. Dolomanov, L. J. Bourhis, R. J. Gildea, J. A. K. Howard and H. Puschmann, J. Appl. Crystallogr., 2009, 42, 339–341 CrossRef CAS.
Footnote |
† Electronic supplementary information (ESI) available: Synthetical details, analytical methods, in silico methods, preparation and characterisation of organic molecules and MOFs, literature survey concerning m-terphenyl diisophthalate coordination polymers and single crystal data. CCDC 1942981–1942987, 1942989 and 1942990. For ESI and crystallographic data in CIF or other electronic format see DOI: 10.1039/c9dt03044a |
|
This journal is © The Royal Society of Chemistry 2019 |