DOI:
10.1039/C8DT03776K
(Communication)
Dalton Trans., 2019,
48, 1941-1946
Energy storage inspired by nature – ionic liquid iron–sulfur clusters as electrolytes for redox flow batteries †
Received
18th September 2018
, Accepted 20th December 2018
First published on 20th December 2018
Abstract
The redox flow battery (RFB) is a promising technology for the storage of electric energy. Many commercial RFBs are often based on acidic vanadium electrolyte solutions that have limitations regarding stability and energy density. Here, a new approach is presented that is inspired by nature's electron storage, i.e. iron–sulfur clusters [Fe4S4(SR)4]2−. In combination with imidazolium cations, new ionic liquid electrolyte materials were obtained and characterized with regard to their physico- and electrochemical properties. For flow battery tests, the bromide/bromine redox-couple was used in the second half cell in an ionic liquid solution. In these measurements, liquid iron–sulfur clusters show high coulombic (>95%) and energy (69%) efficiencies combined with a high theoretical energy density (88 W h L−1).
Introduction
The increasing share of renewable energies leads to an intermittent generation of electric energy. Therefore, it is necessary to provide ways for efficient energy storage, which requires high energy and power densities. Furthermore, a high cycling stability and a low price are targets. Here, the redox flow battery (RFB) is a promising technology. The separated electrolyte tanks ensure a low self-discharge and independent scaling of power and energy outputs up to multi-MW/MWh systems.1 Today's most commonly applied all-vanadium RFBs, however, suffer from a low energy density of max. 40 W h L−1 (ref. 2) compared to Li ion batteries that reach up to ∼700 W h L−1.3,4 Recently, new RFB systems have been described using metal complexes or organic substances in aqueous or non-aqueous solution.5–18 The focus of many studies is on energy density but costs, stability, membrane selectivity and current density are also investigated. The maximum theoretical energy density is proportional to the number of transferred electrons n, the concentrations of the electrolytes c and the potential difference of the two half cells ΔE. This provides three possibilities to improve the energy density:
(i) To increase ΔE, the electrochemical window of the solvent must be wide; therefore water is a limiting factor. Here, ionic liquids (ILs) show great potential due to their wide electrochemical window.19,20
(ii) High concentrations can be achieved if the redox active component is part of the IL as one of its ions. Only two such systems have been described in the literature so far, i.e. a semi-flow battery that deposits copper21 and two systems using liquid metal salts22 or organic anions.23 However, the energy density is not discussed in the latter publication. Therefore, we targeted new redox active ionic liquids with high potentials.
(iii) Finally, the number of transferred electrons per molecule can be increased. This was utilized by the application of different polyoxometalates (POMs)24–29 and organic molecules5,15,30–32 but often due to low concentrations, only low energy densities were achieved.
With respect to these considerations, liquid iron–sulfur cluster salts are proposed as a high energy density electrolyte. These clusters [Fe4S4(SR)4]n− (Fig. 1) are well-known from ferredoxin-proteins and serve as electron reservoirs in nature for numerous purposes.33 They are composed of four iron atoms that are bridged by four μ3-sulfido-ligands and coordinated by a variable thiolato ligand (RS−). They are known to exist in four different oxidation states, making them interesting for energy storage (cf. iii)). Additionally, the high abundance of iron and sulfur makes them attractive, inexpensive and environment friendly energy storage materials. By the combination of the anionic clusters with IL cations (Fig. 1), liquids with promising electrochemical properties are anticipated.
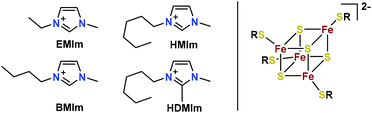 |
| Fig. 1 Ionic liquid cations (left) and the general structure of cubane-type iron–sulfur clusters (right). | |
The use of ILs is emphasized by their beneficial properties like low volatility, non-flammability, good thermal and chemical stability and intrinsic conductivity.34–40 The large amount of different ILs offers numerous possibilities for the synthesis of ILs with specific properties. Therefore, ILs are highly interesting for RFB applications;41 however only a few IL-based systems have been reported so far.23,42,43 Additionally, in comparison with acidic and aqueous electrolytes, like the classic vanadium system, ionic liquids are not corrosive and thus allow the use of less robust materials (e.g. pump, tank, and tubing). Also they are inflammable and non-volatile in contrast to the recently applied organic solvents resulting in a saver application.
Already known ILs with multi-electron transfers are POM-based.44,45 Such ILs were also previously investigated by our group;46 however, due to their low intrinsic energy density, they were not further considered for RFB applications. Other multimetallic systems besides POMs are rare, but a few with oligomeric structures30,31 were reported that aim for an increase in the number of electrons or larger structures to prevent crossover.
Results and discussion
Synthesis
The preparation of iron–sulfur clusters is well established.47 A sodium thiolate is reacted with FeCl3 and sulfur forming [Fe4S4(SR)4]2− in solution, which can be isolated by the addition of an appropriate organic cation. To obtain ionic liquids, we chose suitable cations, e.g. imidazolium salts. In a synthetically more simple procedure, the tetramethylammonium (TMA) salt of the cluster with R = Me was prepared at first and characterized by NMR and X-ray crystal structure analysis (cf. the ESI†). The cation can be exchanged afterwards by a typical IL cation (eqn (1)). With imidazolium cations, cluster salts were obtained as room temperature ILs and characterized (Scheme 1). However, apparent high viscosities make them unsuitable for RFBs.
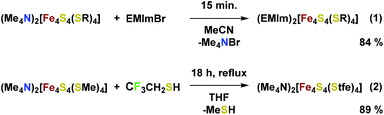 |
| Scheme 1 Synthesis of iron–sulfur cluster based ionic liquids. | |
It is known that ILs with low viscosity often contain fluorinated anions, e.g. BF4− and PF6−.48 Therefore, a new cluster with a fluorinated thiolato ligand was sought. In this case, 2,2,2-trifluoroethanethiol was used to prepare the cluster [Fe4S4(Stfe)4]2− (Stfe = trifluoroethanethiolate) by refluxing a solution of [Fe4S4(SMe)4]2−, while removing gaseous methanethiol from the reaction mixture (eqn (2)). The X-ray crystal structure49 of the TMA salt of [Fe4S4(Stfe)4]2− (Fig. 2) shows the typical structural characteristics of cubane iron–sulfur clusters.50
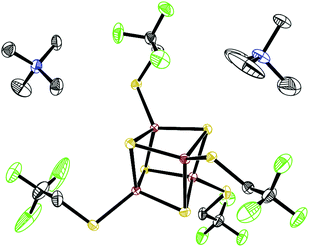 |
| Fig. 2 ORTEP-plot (50%) of (Me4N)2[Fe4S4(SCH2CF3)4]. A co-crystallized THF molecule and the hydrogen atoms are omitted for clarity ( Fe, S, F, N, ● C). | |
Thermo- and physicochemical properties
After cation exchange, several liquids could be obtained. Their melting points were determined by differential scanning calorimetry (Table 1). These salts undergo glass transitions at temperatures between −30 °C and −50 °C. The Stfe-cluster salts show glass transition temperatures Tg lower than those of SMe-cluster salts. As it is known for imidazolium ILs, Tg increases with increasing alkyl chain-length and decreases with methyl substitution in the 2-position.34 The first trend is not observed in this case; however, such a behavior has been reported for other imidazolium ILs.51
Table 1 Glass transition temperatures of IL iron–sulfur clusters [°C]
Thiolate |
Cation |
EMIm |
BMIm |
HMIm |
HDMIm |
SMe |
−42 |
−39 |
−41 |
−32 |
Stfe |
−45 |
−50 |
−50 |
−49 |
The thermal stability of the EMIm- and TMA-salts of [Fe4S4(Stfe)4]2− was investigated by thermogravimetric analysis (TGA), which proved that the salts are stable up to 150 °C. For the application of an ionic liquid in an RFB, its viscosity is also an important parameter. At room temperature, (EMIm)2-[Fe4S4(Stfe)4] has a viscosity of 1800 mPa s, which decreases significantly to 366 mPa s at 50 °C and further to 117 mPa s at 80 °C. The electric conductivity of neat (EMIm)2[Fe4S4(Stfe)4] was determined at different temperatures reaching from 0.16 mS cm−1 at 18 °C to 5.81 mS cm−1 at 101 °C. These values are typical of ionic liquids,39,52,53 but are below those of aqueous systems.54 This can lead to a higher cell resistance in the RFB.
The electrochemistry of [Fe4S4(Stfe)4]2− was investigated by cyclic voltammetry (Fig. 3). In THF, two quasi-reversible reductions (ΔEp1 = 97 mV, ΔEp2 = 190 mV at 100 mV s−1) can be observed at −1.55 V and −2.23 V vs. Fc/Fc+. The measurement of multiple cycles did not alter the shape of the CV-curve, confirming reversibility (s. ESI, Fig. S4†). In ILs, the redox-potentials obtained from the CV are shifted towards more positive potentials (s. ESI, Fig. S3†).
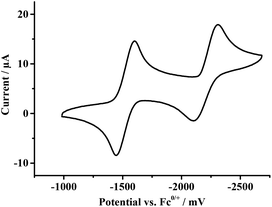 |
| Fig. 3 Cyclic voltammogram of [Fe4S4(Stfe)4]2− (vs. Fc/Fc+), THF (0.1 M nBu4NPF6), 100 mV s−1. | |
The rate constants of the reduction processes were determined according to the Nicholson method55 from the peak separation in the CV. In EMImNTf2, the first reduction has a rate constant of 5.1 × 10−3 cm s−1, while the second reduction shows a rate constant of 4.1 × 10−3 cm s−1. In acetonitrile, the rate constants are 0.91 × 10−2 cm s−1 and 0.78 × 10−3 cm s−1 for the first and second reduction, respectively. This fast electron transfer is common in non-aqueous media in contrast to many aqueous systems, e.g. vanadium electrolytes, where slower kinetics are frequently observed.8,12,56
This reversibility in ILs and the strongly negative redox potentials support the application of iron–sulfur clusters as a redox-active ionic liquid RFB electrolyte.
Flow battery measurements
To investigate the iron sulfur cluster in an RFB, the bromide/bromine redox couple was used as the positive electrolyte in ionic liquid solution (Scheme 2). The Br−/Br2 couple – or in excess of Br−, the Br−/Br3− couple – is well-known from the aqueous Zn/Br-RFB; however, it was not applied in an IL-medium previously. This results in a cell voltage of 1.4 V. For initial tests, a 0.1 M solution of (EMIm)2[Fe4S4(Stfe)4] and a 0.15 M solution of EMImBr in EMImNTf2 were used in the respective half-cells. Both half-cells were separated using an F-14100 (FuMATech) cation exchange membrane. This cell was charged with 1 mA cm−2 until the first reduction step of the cluster was completed using cut-offs at 1.8 V and 0.5 V as limits. This results in a relatively high energy efficiency (EE) of 69% (Fig. 4b), which is comparable with that of other RFB systems.5–12,21,57 It must be emphasized that the applied membrane is optimized for proton transport and not for the much larger imidazolium cations, which impedes the application of higher current densities in combination with the moderate conductivity of ILs. The coulombic efficiency (CE) is at a high level at about 95% considering the early stage of development. With the Br−/Br3− half-cell, a maximum theoretical energy density of approximately 70 W h L−1 (for calculations see ESI, eqn (4)†) can be reached considering that the neat ionic liquid (EMIm)2[Fe4S4(Stfe)4] has a 1.5 M concentration and a 3 M concentration of EMImBr in EMImNTf2 is possible. With the room temperature IL HMImBr which is 5 M, the energy density can be increased to 88 W h L−1. This value could certainly be even further improved by using a catholyte with a more positive potential.
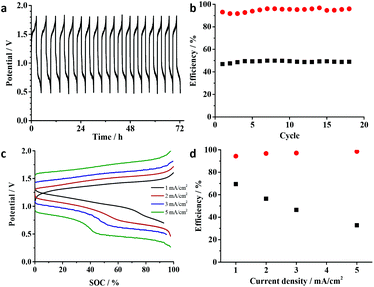 |
| Fig. 4 (a) Charge/discharge voltage-profile at 1 mA cm−2 with 0.1 M (EMIm)2[Fe4S4(Stfe)4] and (b) the corresponding coulombic ( ) and energy (■) efficiencies. (c) Charge/discharge voltage-profiles at different current densities (SOC: state of charge) and (d) the corresponding coulombic ( ) and energy (■) efficiencies. | |
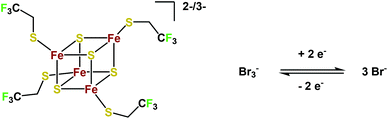 |
| Scheme 2 Redox-reactions of the iron–sulfur cluster ionic liquid RFB. | |
The charge/discharge behavior was investigated at different current densities in order to improve the performance of the battery. By increasing the current density up to 5 mA cm−2, the charge and discharge potentials are shifted towards higher and lower potentials, respectively (Fig. 4c).
Due to this, the energy efficiency is decreased to 56% at 2 mA cm−2 and further to 33% at 5 mA cm−2 (Fig. 4d). The coulombic efficiency is not affected by current density though.
In order to demonstrate that ionic liquid electrolytes can be applied for RFBs with high energy density, (EMIm)2-[Fe4S4(Stfe)4] was used in 1.0 M concentration. Note that this is close to the concentration of the neat redox-active IL of 1.5 M. The measurements were performed at 50 °C to decrease the viscosity of the ionic liquid and at the same time increase its conductivity. The obtained charge/discharge profile (Fig. 5) shows a similar behavior to that of the diluted sample. However, the CE is decreased to approximately 80% in the first cycles, but increases afterwards and reaches 95% after 15 cycles. As a result of the lower CE, a decrease in EE to 60% is observed. In contrast to the increase in CE during the measurement, the EE decreases over time. This effect is most likely caused by the degradation of the membrane causing a higher cell resistance and therefore energy losses. This increased cell resistance could be observed by impedance spectroscopy (see ESI, Fig. S7†).
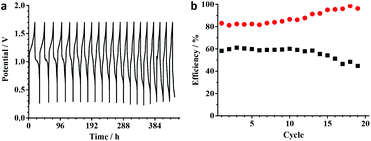 |
| Fig. 5 (a) Charge/discharge voltage-profile at 1 mA cm−2 with 1.0 M (EMIm)2[Fe4S4(Stfe)4] and (b) the corresponding coulombic ( ) and energy (■) efficiencies. | |
The behavior of the 0.1 M electrolyte was investigated by 19F NMR spectroscopy. The paramagnetic shift of the 19F NMR signal of the trifluoroethyl group provides insight into the species present during cycling. While charging the battery, new signals at −2.4 ppm and −55.3 ppm were observed and assigned to [Fe(Stfe)4]2− and [Fe3S4(Stfe)2]2− corresponding to the dismutation of the reduced cluster. This is in contrast to the expected formation of [Fe4S4(Stfe)4]3−, nevertheless upon discharging, [Fe4S4(Stfe)4]2− was restored. During the second cycle, the aforementioned complexes were again formed and in addition [Fe6S9(Stfe)2]2− was generated, as well as non-coordinated tfeS−. These processes explain the non-ideal shape of the charge/discharge profile. This behavior is in contrast to the CV data as well as the bulk electrolysis experiments in acetonitrile, which led to the isolation of (Bu4N)3[Fe4S4(Stfe)4] (s. ESI†). This species was characterized and found to be stable for weeks. So far this differing behavior in the flow cell remains unaccounted, but is further investigated. This flexibility of iron–sulfur cluster structures prevents actual decomposition especially since [Fe4S4(Stfe)4]2− is known to be a thermodynamic sink in iron–sulfur–thiolate chemistry50,58,59 and thus can be reformed.
Another issue that may lead to a capacity decrease is the crossover of bromide or tribromide ions through the membrane. For hydrogen–bromine cells, crossover rates in the range of 10 mg h−1 cm−2 were reported60,61 that were confirmed for quinone–bromine RFBs.62 These values may be in the same range for ILs as well and therefore could contribute to the capacity decay. Thus, this issue will receive further attention in our research concerning the improvement of cell performance.
A comparison with other multimetallic electrolytes is given in Table 2. This shows that the presented ionic liquid electrolyte possesses a higher theoretical energy density than most systems as well as one of the highest demonstrated energy densities. The achieved current density is relatively low; however, this is not uncommon for non-aqueous systems in the early stage of development. The energy efficiency is also sufficiently high and in the same range of other systems. Only the remarkable system of Cronin et al.28 which was only recently published shows a significantly higher energy density than every other RFBs.
Table 2 Multimetallic RFB electrolyte systems and their energy density, current density and energy efficiency
System |
Theor. EDa [W h L−1] |
Appl. EDb [W h L−1] |
J [mA cm−2] |
EE [%] |
Ref. |
Maximum possible energy density calculated with the maximum solubility and redox-potentials.
Applied energy density in a test cell calculated with the utilized concentrations.
|
[SiV3W9O40]10− |
≈73 |
1.6 |
2.0 |
≈30 |
24
|
[SiV3W9O40]10− |
[Co6S8(PTA)6] |
1.5 |
0.023 |
≈0.1 |
≈50 |
63
|
Methylviologene |
[CoW12O40]6− |
15.4 |
15.4 |
25–100 |
86 |
25
|
[CoW12O40]6− |
[SiW12O40]4− |
? |
≈7 |
30–60 |
70 |
26
|
[PV14O42]9− |
[TiV5O6(OMe)13]− |
15.8 |
0.16 |
0.015 |
≈50 |
27
|
[TiV5O6(OMe)13]− |
[P2W18O62]6− |
1000 |
225 |
50 |
76 |
28
|
Br− |
[V6O7(OR)9(OCH2)3R’] |
14.6 |
8.6 |
0.4 |
45 |
29
|
[V6O7(OR)9(OCH2)3R’] |
Perylene diimide |
20.0 |
4.6 |
1.16 |
68 |
30
|
C(O(CH2)6Fc)4 |
EMIm2[Fe4S4Stfe)4]2− |
87.7 |
43.6 |
1.0 |
69 |
Herein |
Br− |
In comparison with aqueous all-vanadium RFBs, a significant increase in energy density is achieved. However, the accessible current density is two orders of magnitude lower than that for vanadium systems.
Conclusions
In summary, for the first time, nature's electron storage iron–sulfur clusters were adopted for energy storage in RFBs. For this purpose, new room temperature ionic liquids were prepared and characterized. Their electro- and physicochemical properties were investigated, showing two redox waves, a sufficient viscosity and conductivity. Together with their high thermal stability, these redox-active ionic liquids are promising electrolytes for one of the first IL-based RFBs. In combination with a bromide/tribromide based IL, the energy storage capability was demonstrated with a high energy density. Further adjustments to improve the performance of this system especially concerning the use of other membrane materials are currently ongoing.
Conflicts of interest
There are no conflicts to declare.
Acknowledgements
Funding of this project (tubulAir±, FKZ 03SF0436C) by the Bundesministerium für Bildung und Forschung is gratefully acknowledged. The authors thank Uta Sazama for TGA-MS and DSC measurements, Dr Daniel Szopinski for rheological experiments and Dr Bernhard Bugenhagen for solving the crystal structure.
Notes and references
- Z. Yang, J. Zhang, M. C. W. Kintner-Meyer, X. Lu, D. Choi, J. P. Lemmon and J. Liu, Chem. Rev., 2011, 111, 3577 CrossRef CAS PubMed.
- L. Li, S. Kim, W. Wang, M. Vijayakumar, Z. Nie, B. Chen, J. Zhang, G. Xia, J. Hu, G. Graff, J. Liu and Z. Yang, Adv. Energy Mater., 2011, 1, 394 CrossRef CAS.
- K. M. Abraham, J. Phys. Chem. Lett., 2015, 6, 830 CrossRef CAS PubMed.
- J. W. Choi and D. Aurbach, Nat. Rev. Mater., 2016, 1, 16013 CrossRef CAS.
- B. Huskinson, M. P. Marshak, C. Suh, S. Er, M. R. Gerhardt, C. J. Galvin, X. Chen, A. Aspuru-Guzik, R. G. Gordon and M. J. Aziz, Nature, 2014, 505, 195 CrossRef CAS PubMed.
- T. Janoschka, N. Martin, U. Martin, C. Friebe, S. Morgenstern, H. Hiller, M. D. Hager and U. S. Schubert, Nature, 2015, 527, 78 CrossRef CAS PubMed.
- B. Hu, C. DeBruler, Z. Rhodes and T. L. Liu, J. Am. Chem. Soc., 2017, 139, 1207 CrossRef CAS PubMed.
- K. Gong, Q. Fang, S. Gu, S. F. Y. Li and Y. Yan, Energy Environ. Sci., 2015, 8, 3515 RSC.
- X. Wei, W. Duan, J. Huang, L. Zhang, B. Li, D. Reed, W. Xu, V. Sprenkle and W. Wang, ACS Energy Lett., 2016, 1, 705 CrossRef CAS.
- J. Luo, B. Hu, C. DeBruler and T. L. Liu, Angew. Chem., Int. Ed., 2018, 57, 231 CrossRef CAS.
- A. M. Kosswattaarachchi and T. R. Cook, Electrochim. Acta, 2018, 261, 296 CrossRef CAS.
- J. Winsberg, T. Hagemann, T. Janoschka, M. D. Hager and U. S. Schubert, Angew. Chem., Int. Ed., 2017, 56, 686 CrossRef CAS PubMed.
- L. E. VanGelder, A. M. Kosswattaarachchi, P. L. Forrestel, T. R. Cook and E. M. Matson, Chem. Sci., 2018, 9, 1692 RSC.
- J. M. Stauber, S. Zhang, N. Gvozdik, Y. Jiang, L. Avena, K. J. Stevenson and C. C. Cummins, J. Am. Chem. Soc., 2018, 140, 538 CrossRef CAS.
- C. S. Sevov, K. H. Hendriks and M. S. Sanford, J. Phys. Chem. C, 2017, 121, 24376 CrossRef CAS.
- C. DeBruler, B. Hu, J. Moss, J. Luo and T. L. Liu, ACS Energy Lett., 2018, 3, 663 CrossRef CAS.
- A. Mukhopadhyay, J. Hamel, R. Katahira and H. Zhu, ACS Sustainable Chem. Eng., 2018, 6, 5394 CrossRef CAS.
- W. Duan and X. Wei,
et al.
, ACS Energy Lett., 2017, 2, 1156 CrossRef.
- M. Hayyan, F. S. Mjalli, M. A. Hashim, I. M. AlNashef and T. X. Mei, J. Ind. Eng. Chem., 2013, 19, 106 CrossRef CAS.
-
A. A. J. Torriero, Electrochemistry in Ionic Liquids, Springer International Publishing, Cham, 2015 Search PubMed.
- S. Schaltin, Y. Li, N. R. Brooks, J. Sniekers, I. F. J. Vankelecom, K. Binnemans and J. Fransaer, Chem. Commun., 2016, 52, 414 RSC.
-
T. M. Anderson, D. Ingersoll, C. Staiger and H. Pratt, Sandia Corporation, US9123943B1, 2015 Search PubMed.
-
T. Nokami, T. Ito, H. Sakaguchi and H. Usui, Tottori Univ., JP2016033117A, 2016 Search PubMed.
- H. D. Pratt III, N. S. Hudak, X. Fang and T. M. Anderson, J. Power Sources, 2013, 236, 259 CrossRef.
- Y. Liu, S. Lu, H. Wang, C. Yang, X. Su and Y. Xiang, Adv. Energy Mater., 2017, 7, 1601224 CrossRef.
- J. Friedl, M. V. Holland-Cunz, F. Cording, F. L. Pfanschilling, C. Wills, W. McFarlane, B. Schricker, R. Fleck, H. Wolfschmidt and U. Stimming, Energy Environ. Sci., 2018, 11, 3010 RSC.
- L. E. VanGelder and E. M. Matson, J. Mater. Chem. A, 2018, 6, 13874 RSC.
- J.-J. Chen, M. D. Symes and L. Cronin, Nat. Chem., 2018, 10, 1042 CrossRef CAS PubMed.
- L. E. VanGelder, B. E. Petel, O. Nachtigall, G. Martinez, W. W. Brennessel and E. M. Matson, ChemSusChem, 2018, 11, 4139 CrossRef CAS PubMed.
- M. Milton, Q. Cheng, Y. Yang, C. Nuckolls, R. H. Sánchez and T. J. Sisto, Nano Lett., 2017, 17, 7859 CrossRef CAS PubMed.
- J. Friedl, M. A. Lebedeva, K. Porfyrakis, U. Stimming and T. W. Chamberlain, J. Am. Chem. Soc., 2018, 140, 401 CrossRef CAS PubMed.
- C. S. Sevov, S. L. Fisher, L. T. Thompson and M. S. Sanford, J. Am. Chem. Soc., 2016, 138, 15378 CrossRef CAS PubMed.
- H. Beinert, Science, 1997, 277, 653 CrossRef CAS.
-
P. Wasserscheid and T. Welton, Ionic Liquids in Synthesis, Wiley-VCH Verlag GmbH & Co. KGaA, Weinheim, 2007 Search PubMed.
- M. Watanabe, M. L. Thomas, S. Zhang, K. Ueno, T. Yasuda and K. Dokko, Chem. Rev., 2017, 117, 7190 CrossRef CAS PubMed.
- S. Sowmiah, V. Srinivasadesikan, M.-C. Tseng and Y.-H. Chu, Molecules, 2009, 14, 3780 CrossRef CAS PubMed.
- C. Maton, N. de Vos and C. V. Stevens, Chem. Soc. Rev., 2013, 42, 5963 RSC.
- O. Zech, A. Stoppa, R. Buchner and W. Kunz, J. Chem. Eng. Data, 2010, 55, 1774 CrossRef CAS.
- A. Stoppa, O. Zech, W. Kunz and R. Buchner, J. Chem. Eng. Data, 2010, 55, 1768 CrossRef CAS.
-
B. Kirchner, Ionic Liquids, Springer, Berlin, Heidelberg, 2010 Search PubMed.
- M. V. Fedorov and A. A. Kornyshev, Chem. Rev., 2014, 114, 2978 CrossRef CAS PubMed.
- A. Ejigu, P. A. Greatorex-Davies and D. A. Walsh, Electrochem. Commun., 2015, 54, 55 CrossRef CAS.
-
J. Noack, J. Tübke and K. Pinkwart, Fraunhofer Gesellschaft, EP2399317B1, 2010 Search PubMed.
- P. G. Rickert, M. R. Antonio, M. A. Firestone, K.-A. Kubatko, T. Szreder, J. F. Wishart and M. L. Dietz, J. Phys. Chem. B, 2007, 111, 4685 CrossRef CAS PubMed.
- A. B. Bourlinos, K. Raman, R. Herrera, Q. Zhang, L. A. Archer and E. P. Giannelis, J. Am. Chem. Soc., 2004, 126, 15358 CrossRef CAS PubMed.
- S. S. Mal, O. Tröppner, I. Ivanović-Burmazović and P. Burger, Eur. J. Inorg. Chem., 2013, 2013, 1960 CrossRef CAS.
- G. Christou and C. D. Garner, J. Chem. Soc., Dalton Trans., 1979, 1093 RSC.
- G. Yu, D. Zhao, L. Wen, S. Yang and X. Chen, AIChE J., 2012, 58, 2885 CrossRef CAS.
- CCDC 1573435† contains the supplementary crystallographic data for this paper.
- V. R. Pallem and R. H. Holm, Chem. Rev., 2004, 104, 527 CrossRef PubMed.
- M. Blesic, M. Swadźba-Kwaśny, T. Belhocine, H. Q. N. Gunaratne, J. N. C. Lopes, M. F. C. Gomes, A. A. H. Pádua, K. R. Seddon and L. P. N. Rebelo, Phys. Chem. Chem. Phys., 2009, 11, 8939 RSC.
- K. J. Fraser, E. I. Izgorodina, M. Forsyth, J. L. Scott and D. R. MacFarlane, Chem. Commun., 2007, 39, 3817 RSC.
- D. R. MacFarlane, M. Forsyth, E. I. Izgorodina, A. P. Abbott, G. Annat and K. Fraser, Phys. Chem. Chem. Phys., 2009, 11, 4962 RSC.
- F. Rahman and M. Skyllas-Kazacos, J. Power Sources, 2009, 189, 1212 CrossRef CAS.
- R. S. Nicholson, Anal. Chem., 1965, 37, 1351 CrossRef CAS.
- C. Choi, S. Kim, R. Kim, Y. Choi, S. Kim, H.-y. Jung, J. H. Yang and H.-T. Kim, Renewable Sustainable Energy Rev., 2017, 69, 263 CrossRef CAS.
- I. L. Escalante-Garcia, J. S. Wainright, L. T. Thompson and R. F. Savinell, J. Electrochem. Soc., 2014, 162, A363–A372 CrossRef.
- R. H. Holm and W. Lo, Chem. Rev., 2016, 116, 13685 CrossRef CAS.
- K. S. Hagen, J. G. Reynolds and R. H. Holm, J. Am. Chem. Soc., 1981, 103, 4054 CrossRef CAS.
- G. Lin, P. Y. Chong, V. Yarlagadda, T. V. Nguyen, R. J. Wycisk, P. N. Pintauro, M. Bates, S. Mukerjee, M. C. Tucker and A. Z. Weber, J. Electrochem. Soc., 2015, 163, A5049–A5056 CrossRef.
- M. C. Tucker, K. T. Cho, F. B. Spingler, A. Z. Weber and G. Lin, J. Power Sources, 2015, 284, 212 CrossRef CAS.
- Q. Chen, L. Eisenach and M. J. Aziz, J. Electrochem. Soc., 2015, 163, A5057–A5063 CrossRef.
- M. B. Freeman, Le Wang, D. S. Jones and C. M. Bejger, J. Mater. Chem. A, 2018, 6, 21927 RSC.
Footnote |
† Electronic supplementary information (ESI) available: Experimental details, syntheses, scan-rate-dependent CVs of [Fe4S4(Stfe)4]2−, Randles–Ševčík-plots, TGA-plots, conductivity- and viscosity-data, NMR-spectra and X-ray-data. CCDC 1573434 and 1573435. For ESI and crystallographic data in CIF or other electronic format see DOI: 10.1039/c8dt03776k |
|
This journal is © The Royal Society of Chemistry 2019 |