DOI:
10.1039/C8CS00768C
(Review Article)
Chem. Soc. Rev., 2019,
48, 5488-5505
The challenges of glycan recognition with natural and artificial receptors
Received
1st July 2019
First published on 25th September 2019
Abstract
Glycans – simple or complex carbohydrates – play key roles as recognition determinants and modulators of numerous physiological and pathological processes. Thus, many biotechnological, diagnostic and therapeutic opportunities abound for molecular recognition entities that can bind glycans with high selectivity and affinity. This review begins with an overview of the current biologically and synthetically derived glycan-binding scaffolds that include antibodies, lectins, aptamers and boronic acid-based entities. It is followed by a more detailed discussion on various aspects of their generation, structure and recognition properties. It serves as the basis for highlighting recent key developments and technical challenges that must be overcome in order to fully deal with the specific recognition of a highly diverse and complex range of glycan structures.
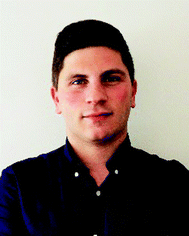
Stefano Tommasone
| Stefano Tommasone is a Research Fellow at the University of Birmingham (UK). He obtained his PhD in Chemistry (2016) at the University of Salerno (Italy) with Prof. Gaeta, in the Neri group, where he worked on the synthesis of calixarenes able to interact with biomolecular targets. He was also a visiting PhD student at the IBMM (Montpellier, France) under the supervision of Prof. Marra, working on a new ligation strategy for the synthesis of glycoconjugates. He then joined the Mendes group (2016), where he investigates glycan recognition with boronic acid derivatives for applications in diagnostics. |
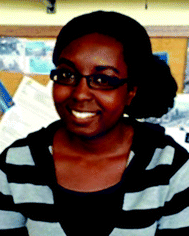
Francia Allabush
| Francia Allabush obtained her MChem in Medicinal Chemistry at the University of Warwick in 2011. After a brief stint in industry as a research chemist, she undertook a PhD in Chemistry at the University of Birmingham where she is now a Research Fellow. Her current research interests lie in DNA nanotechnology, specifically modifying DNA aptamers for sensing applications. |
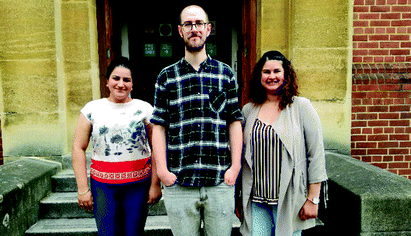
From left to right: Yazmin K. Tagger, Joshua Norman and Monika Köpf
| Yazmin K. Tagger – BSc in Pharmaceutical Science (2012) at the University of Wolverhampton and MRes in Cancer Science (2012) at the University of Birmingham. She is a PhD candidate at the University of Birmingham, working on molecular imprinting on surfaces as a novel diagnostic tool for prostate cancer. Joshua Norman – BSc in Applied Biomedical Science and MRes in Structural Biology – is a PhD candidate at the University of Birmingham. His studies are focused on the development of new point of care diagnostics for prostate cancer biomarkers. Monika Köpf – MRes (2013) in Molecular and Cellular Biology – is a PhD candidate at the University of Birmingham. Her work focused on the development of glycan recognition platforms based on surface molecular imprinting for sensing and diagnostics. |
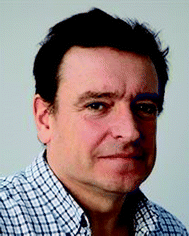
James H. R. Tucker
| Jim Tucker is a supramolecular chemist with research interests in nucleic acid chemistry, bioorganometallic chemistry and functional supramolecular systems. He has held academic positions at the University of Exeter (1995–2005) and at the University of Birmingham (since 2005), where he is currently Professor of Supramolecular Chemistry. |
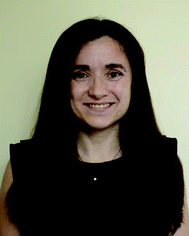
Paula M. Mendes
| Paula Mendes is a Professor of Advanced Materials and Nanotechnology in the School of Chemical Engineering, University of Birmingham, UK. She received her MSc (1997) and PhD (2002) degrees in Chemical Engineering from the University of Porto, Portugal. She undertook post-doctoral research at the University of Birmingham, UK (2002–2004) and at the University of California, Los Angeles, USA (2004–2006). Her research interests lie in the development of novel methods for controlling the structure and functionality of materials at the molecular and nanometre scales and their application in biology and medicine. |
1. Introduction
Glycans occur as free polysaccharides or as mono, oligo or polysaccharides conjugated to a wide variety of biological molecules, which include glycoproteins, glycolipids and peptidoglycans (Fig. 1). In contrast to RNA, DNA and protein synthesis, glycan biosynthesis is not a template-driven process. Instead, glycans are assembled by the expression and activity levels of a series of enzymes present in cells via glycosylation.1 Despite being generated from a limited number of monosaccharides, glycans are characterised by a remarkable structural diversity due to the nature and sequence of the constituent units, the possible branching of the carbohydrate chains and the configuration and position of glycosidic linkages. Monosaccharides have multiple hydroxyl moieties that can serve as linking groups, and the glycosidic bond at the anomeric carbon can have either α or β stereochemistry, leading to a wide range of potential linkages between two monosaccharide units. Furthermore, glycans can be further diversified by a range of modifications, including sulfation, phosphorylation, and acetylation. It is this diversity that makes glycans perform a vast array of biological functions and play important roles in various physiological and pathophysiological events, including cell growth, cell signaling, cell–cell interactions, differentiation and tumour growth.2,3
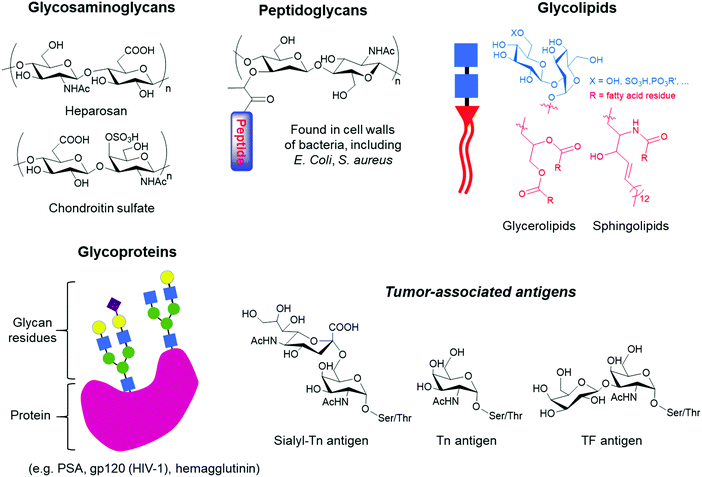 |
| Fig. 1 Examples of glycans that can be found in nature, ranging from glycosaminoglycan polysaccharides to glycoconjugates such as peptidoglycans, glycolipids and glycoproteins, which can bear tumour-associated antigens. | |
Glycans carry information in biological systems that make them an important source of biomarkers for a wide range of diseases, including neurodegenerative diseases, hereditary disorders, immune deficiencies, cardiovascular diseases and many types of cancers.4–6 During the development and progression of these diseases, glycan expression is altered due to misregulation of enzymes such as glycosyltransferases and glycosidases, leading to changes in glycan structures that can potentially be used to accurately identify the disease at an early stage. In addition to their clinical value in disease diagnosis and management, glycans are an emerging class of therapeutics,7 ideal markers for identifying and isolating specific cell types, including stem cell lineages for therapeutic transplantation,8 and among other facets, they are key targets in drug discovery.9 Regarding the latter, glycans are present on the surface of a variety of pathogens and malignant cells, making them ideal targets for vaccines.
The recognition of glycans by other molecules with high affinity and exquisite specificity is at the heart of current and further developments in glycan-related basic research and clinically relevant diagnostics and therapeutic applications. However, there are unique challenges associated with such recognition processes since the glycan-binding entities need to be able to discriminate between a large repertoire of carbohydrate structures, including closely related isomers. Subtle differences, such as the stereochemistry of a single hydroxyl group, make selective recognition hard to achieve. Moreover, strong solvation in water poses another major challenge since receptors must be able to compete with an extensive network of hydrogen bonds surrounding the glycans. Unlike nucleic acids or proteins, it is difficult to recognise glycans using complementary sequences or specific antibodies. Glycans are poorly immunogenic, posing major hurdles in the development of highly selective anti-carbohydrate antibodies.10 While efforts are underway to tackle the low affinities and promiscuous specificity of anti-glycan antibodies, other major strategies involving either lectins,11 aptamers12 and boronic acid derivatives13,14 are concurrently and actively being pursued (Fig. 2).
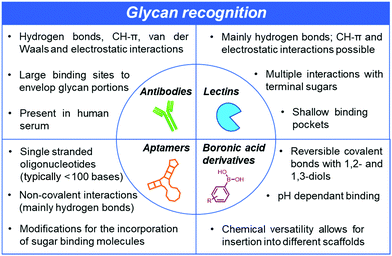 |
| Fig. 2 Glycan recognition can either be achieved using biologically-derived antibodies and lectins or chemically-derived aptamers or other smaller synthetic receptors such as boronic acids. While they all rely on supramolecular interactions, the methods for their generation differ greatly. These differences provide opportunities but also different types of challenges to create glycan-binding scaffolds with high affinity and exquisite specificity. | |
This critical review focuses on the recent progress on all the different strategies to achieve superior glycan recognition, without considering the substantial efforts concentrated on the binding of monosaccharides that are extensively reported elsewhere.15–20 Particular emphasis will be given to the binding characteristics (i.e. affinity and selectivity) of the glycan-binding entities and their potential for further structural glycan differentiation. The purpose of this review is not to provide a comprehensive survey of the literature on antibodies, lectins and synthetic receptors such as boronic acid derivatives and aptamers as entities for the recognition of glycans. Rather examples are provided to highlight main current capabilities and limitations of biologically- and chemically-derived glycan-binding scaffolds. These serve as the framework to bring together as a whole the field of glycan recognition, identify key challenges across the field and potential avenues for further progress on creating glycan-binding scaffolds with high affinity and, more importantly, with great specificity, for a wide, relevant range of glycan structures. The advantages and drawbacks associated with the production and use of glycan-binding scaffolds of biological or synthetic origin are also examined.
2. Anti-glycan antibodies (AGA)
Antibodies are produced by the immune system in response to a foreign molecule, namely antigen, entering the body. A given antibody binds specifically only to a small site on its antigen, called an epitope, which usually consists of a few amino acids or monosaccharide units. An ideal antibody should have high specificity and avidity for its antigen. However, glycans pose a serious challenge to antibody development. While selectivity has been observed in some auto-AGAs, other AGAs have been found to interact with the same antigen, even if with different affinities. Development of highly selective anti-glycan antibodies has been hindered both by the inherently poor immunogenicity of carbohydrates, labour intensive antigenic material production and the similarity between various carbohydrate sequences.21 There are also difficulties in generating anti-glycan antibodies because a wide variety of complex glycans are intrinsically expressed in standard host organisms used to produce antibodies.
A vast collection of AGAs are present in human sera and play critical functions in many immune processes. AGA expression depends on exposure to both foreign carbohydrate sequences, as seen on pathogen surfaces, and to sequences from the “self” of the same species (i.e. blood group antigens A and B). Despite some AGAs exhibiting affinity for naturally occurring glycans, to date they cannot be considered true auto-antibodies due to apparent lack of selectivity for native carbohydrate sequences.22 Prevalent natural AGAs in human sera include those to foreign glycan epitopes such as N-glycolylneuraminic acid (Neu5GC) and galactose-α-1,3-galactose (α-Gal), antigens responsible for the rejection of xenotransplanted organs in humans from pigs.23–25 Other extensively studied AGAs are the anti-blood group antibodies against A, B and rare ‘Bombay’ type O antigens, raised in vivo in the absence of respective erythrocyte surface antigen. They are highly specific and able to differentiate, for instance, between the glycan structures of the A- and B-antigens, wherein an acetamido moiety in A is replaced with a hydroxyl group in B.26
Auto-AGAs found in serum are now useful biomarkers in the diagnosis of inflammatory and autoimmune diseases, including multiple sclerosis and Crohn's disease.27 Cancer patients are also able to produce anti-glycan antibodies, which opens up the possibility of their use for early detection of cancer.28,29 While promising for diagnosis, they are not effective in activating the immune system and eliminating malignant cells, limiting progress in generating new immunotherapies against cancer.30–32
Typically, glycan-binding antibodies exhibit lower affinities (with equilibrium dissociation constant (KD) values in the micromolar range for monovalent interactions) than protein-specific antibodies (with KD values in the nanomolar range).33,34 However, affinities can be enhanced by generating antibodies containing two or more glycan binding sites, or through non-covalent assembly into oligomers with multiple binding sites.35,36 The formation of a multivalent complex can result in higher affinities and enhanced selectivities.37,38 Remarkably, human antibody 2G12 was found to bind strongly to HIV-1 glycoprotein gp120 (KD of 5.6 nM) via formation of a dimer that establishes multiple complex interactions with the oligomannose epitope of the glycoprotein.39,40 Furthermore, phage-display technology has been instrumental in generating AGAs against a broader range of glycans with superior affinity.41–43 Phage display is an in vitro technique developed in the mid-80s that allows the study of protein–ligand interactions between a protein fragment exposed on the surface of a phage particle and a ligand of interest.44–46 T-nouvelle (Tn) antigen (GalNAcα1-Ser/Thr), highly expressed in various tumours, is an interesting target for cancer therapy. However, due to its poor immunogenicity, the development of an anti-Tn antibody is extremely challenging. A novel library (6 × 105 variants) of single-chain antibody fragments (scFv) for the Tn antigen was constructed using phage display technology.41 The best scFv fragments were attached to an antibody constant region (Fc) and converted to scFv-Fc fusion proteins, which showed high specificity against the Tn antigen. Phage display was also utilised to explore the recognition requirements of 2G12 towards gp120.47 A set of amino acid residues on a 2G12 scaffold was varied in a restricted manner in order to identify the minimal physicochemical requirements for oligomannose recognition. Investigation of the generated phage display libraries provided insights into glycan-antibody interactions. In addition, the study revealed that there was a high degree of tolerance within the substitution of five to eight residues. Although in this case the binding affinity was negatively affected, the antibodies retained specificity for the target glycan, leaving positive perspectives for future improvements. Phage-display technology, coupled with new advances in protein engineering, are opening up new opportunities to tailor key antibody structural features to endow AGAs with the ability to form high-affinity interactions with glycan moieties.
A growing understanding of the immunological mechanisms by which the immune system refines its antibodies, together with recent technological advances in carbohydrate chemistry and bioconjugation are also enabling the production of high-affinity anti-glycan antibodies.48,49 By using Qβ virus-like particles (VLPs) conjugated via copper-catalyzed azide–alkyne cycloaddition with short synthetic glycans, anti-glycan IgG antibodies with nanomolar affinity were produced (Fig. 3).48 The controlled glycan density displayed on the virus-like particles facilitated not only B but also T cell recognition, which subsequently provided T cells with help in promoting affinity maturation in B cells.
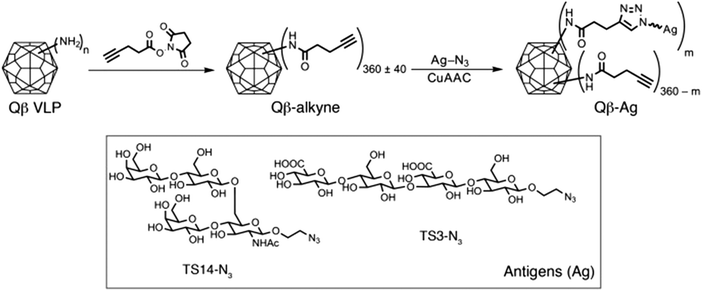 |
| Fig. 3 Synthetic scheme illustrating the functionalization of Qβ VLPs with two oligosaccharide antigens (Ag), TS14 and TS3; m represents the number of antigens per VLP. Reproduced from ref. 48 with permission from the American Society for Clinical Investigation, copyright 2017. | |
Similarly to lectin–carbohydrate interactions, AGA–carbohydrate complexes are held together by hydrogen bonds, CH–π, van der Waals and electrostatic interactions.33,50 However, unlike lectins, antibodies are able to accommodate longer glycan epitopes in their binding sites and can generally interact with all monosaccharide units. Thus, the enveloping capacity of antibody binding sites can allow for very specific recognition.51,52 A range of experimental techniques, including synthetic chemistry, antibody engineering, microarray technology and NMR, alongside computational approaches have been key to elucidate molecular structural features that modulate glycan–antibody recognition.53–57 In this regard, antibody engineering and molecular modelling have been applied to demonstrate that in both pyranoside- and furanoside-antibody systems, the affinity and specificity mostly rely on CH–π interactions.54 While hydrophobic stacking interactions and hydrogen bonds are prevalent in the recognition of neutral glycans by AGAs, ionic interactions have been shown to play a key role in the binding of charged glycans.55 Such interactions result in high glycan-antibody affinities (Kd values in the nanomolar range), which are generally associated with slow dissociation rates. Antibodies bearing positively charged amino acid residues in their binding domain can similarly recognise charged carbohydrate antigens, such as 3-deoxy-D-manno-oct-2-ulosonic acid (or Kdo, a trisaccharide sequence present in Chlamydiae) with KD values <1 μM.58 Modulation of the electrostatic interactions can therefore be used to improve the binding properties of antibodies.59,60 Furthermore, combination of microarray screening and saturation transfer difference NMR provide key information regarding the role of the amino acid linker, such as serine or threonine, in the binding process.56 A related approach has also been used to elucidate the effects of multiple O-glycosylation states on antibody recognition.57 These and future insights on the principles governing glycan-antibody interactions are quite valuable for guiding antibody engineering and creating highly-specific AGAs.
Well-defined validated specificities are now possible due to, recent improvements in glycan microarray-based technology.61,62 Over 1000 AGAs have been reported, although many of them target the same glycan structure, bringing the current total number of glycans being specifically recognised by AGAs to one-quarter of the AGAs available.21 Tumour-associated carbohydrates, such as the Tn (GalNAcα1-Ser/Thr), Sialyl-Tn, (Siaα2-3GalNAcα1-Ser/Thr), and the Thomsen–Friedenreich (TF) antigen (Galβ1-3GalNAcα1-Ser/Thr), have been particularly targeted for AGA development.63,64 There is also a good number of AGAs for ABH blood group antigens, Lewis antigens and glycolipids.65–67 Indeed, these developed antibodies are either currently in clinical trials or already employed in disease treatment. They have also been used for diagnostic applications, as is the case for the antibody that targets Sialyl Lewis A for detecting and monitoring several cancers.68
Despite considerable efforts, many current AGAs display low affinities for their target glycans and a lack of specificity, recognising families of structurally related glycans, rather than a single distinct structure. Furthermore, available AGAs cover a narrow set of glycan families and epitopes and many important O-glycans, N-glycans, glycosaminoglycans and modified glycans (e.g. sulphated glycans) still lack a corresponding antibody.21 AGAs that can discern a specific amino acid residue (e.g. serine versus threonine) involved in the glycopeptide bond are also needed. Therefore, there are still many opportunities for the development of new AGAs for impacting on basic research, as well as for new diagnostic and therapeutic applications. Innovations in and beyond the initial phage display technology and their combination with other emergent technologies, as well as advances in glycan synthesis and glycan arrays for high-throughput AGA analysis, are expected to play a key role in creating a larger repertoire of AGAs with high binding affinities and selectivities.
3. Lectins
Lectins are carbohydrate-binding proteins of non-immune origin that are present in plants, animals and microorganisms. They are involved in many physiological events and currently play a crucial role as tools for glycan probing, purification of glycoproteins, cell labelling, carriers in targeted therapies and detection of cancer and other diseases.69–71 Lectins display relatively weak affinities for monosaccharides, with dissociation constants (KD) in the millimolar range. Affinities are still generally in the micromolar range for oligosaccharides and glycans, despite the opportunity for multiple contacts with the surface of the lectin. This is due to the shallow binding pockets at the lectin surface, which is exposed to competitive solvent interactions. The absence of deeper binding pockets can also explain their poor selectivities for individual sugars. However, in biological settings, some lectins can assemble into homo-oligomeric structures with multiple binding sites in order to achieve superior affinity and selectivity. Thus, an oligomer can interact in an effective manner with different arms of a branched oligosaccharide or to different glycan sites of the same glycoprotein. In such oligomeric arrangements, a high degree of multivalency can be reached, contributing to affinities in the nanomolar range.72–76
Lectins interact with glycans primarily via a network of hydrogen bonds between the ring oxygen atom and multiple hydroxyl groups of the carbohydrate residues and oxygen atoms, amide and hydroxyl groups of the protein, as well as van der Waals contacts.72,77,78 Nevertheless, CH–π interactions between the carbohydrate backbone and aromatic amino acids such as phenylalanine, tyrosine, or tryptophan also make an important contribution to the overall binding.77,79 The Ralstonia solanacearum (RSL) lectin can establish CH–π contacts with the C3, C4, C5 and C6 carbons of α-L-Me-fucoside via the indole ring of a tryptophan residue, with the dispersion interactions contributing to the binding with an energy between 7 and 8 kcal mol−1.80 Electrostatic interactions become relevant for the recognition of charged carbohydrate residues, as in the case for negatively charged sialic acids. In this regard, positively-charged arginine residues are often involved in sialic acid interaction processes.81 Examples of intermolecular contacts between lectins and glycans at the binding site can be found in a study reporting the investigation of the complexation of Siglec-8 lectin and its target, 6′-sulfo sialyl Lewis X (6′S sLex) (Fig. 4).82 Among several other interactions between the lectin and the glycan, the carboxyl group of the sialic acid residue makes a salt bridge with the guanidinium group of Arg109. Moreover, Lys116 and Ser118 engage in a network of hydrogen bonds with the N-acetyl amide and the O8 and O9 hydroxyl groups of the glycerol chain. In addition, the binding is promoted by hydrophobic contacts with the surrounding aromatic rings of other amino acid residues such as Tyr11 and Trp117. The binding of the Gal6S is mediated by the side chains of three residues (Arg56, Tyr58, and Gln59), together with a salt bridge with the guanidinium group of Arg56 and/or a hydrogen bond with the amino group of Gln59.
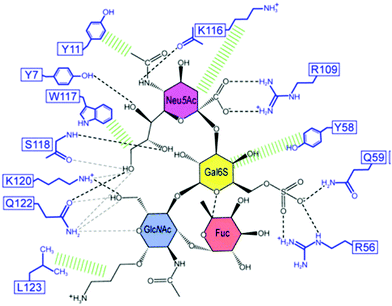 |
| Fig. 4 Schematic illustration of the Siglec-8–6′S sLex interaction network. Black dashed lines indicate hydrogen bonds in the depicted structure; gray dashed lines indicate hydrogen bonds abundantly observed in other structures of the ensemble. Hydrophobic contacts are shown in green. Reproduced from ref. 82 with permission from the National Academy of Sciences, copyright 2016. | |
The carbohydrate binding activity of C-type lectins, which are involved in many cell surface recognition events, is mediated by Ca2+ ions that coordinate carboxylate residues.83,84 Dectin-2, a C-type lectin specific for high-mannose glycans can for instance, trigger a defence response against pathogens in a Ca2+-dependent process.85,86
Currently, several hundred lectins have been identified, with about 100 lectins commercially available. A major breakthrough in the use of lectins for glycan recognition came from the development of the lectin microarray. The technique relies on the immobilization of a panel of lectins on a solid support that allows for high-throughput analysis of complex carbohydrates included in serum glycoproteins, bacteria and whole cells.69,87,88 The advantage of lectin microarrays over other conventional glycan characterization techniques, namely mass spectrometry, NMR spectroscopy and liquid chromatography, is the possibility to rapidly obtain a broad profile or fingerprint of the glycans present in a crude sample, with minute sample consumption and reduced cost. General information about the glycosylation pattern can be determined, such as whether it is N-glycosylated, O-glycosylated, high mannose, core fucosylated, and fully or partially sialylated, with potential applications in the development of disease-related biomarkers.11,87,89–91
Despite the benefits associated with lectin microarrays, the inherent low affinity and specificity of lectins hinder their detection performances. In order to improve their binding properties, researchers have started to focus their efforts on lectin engineering.92–97 Several types of lectins can be used as scaffolds, such as L-type, F-type, Galectins and R-type, with different engineering procedures available (e.g. site-directed mutagenesis, random mutagenesis and DNA shuffle to name a few).97 Recently, the engineering of a bacterial F-type lectin domain (FLD) from Streptosporangium roseum, namely SrFLD, showed significantly improved binding towards multivalent fucosylated glycoconjugates.98 The engineered lectin, SrDupFLD, contained a partial duplication of the original FLD sequence, affording two possible L-fucose binding pockets. Binding studies showed that the engineered lectin had a stronger binding for multivalent fucosylated glycoconjugates, with a dissociation constant 12-fold lower than the wild-type lectin SrFLD. Analogously, lectins in which the L-fucose binding residues of the N-terminal partial FLD region and the complete FLD region were mutated, respectively SrDupTMFLD and SrDupFLDTM, and were expressed and purified. Increased binding avidity was observed for SrDupTMFLD. Against what was its original hypothesis, the study revealed that the increased affinity of the engineered lectins may not be mediated by additional L-fuctose binding sites in the Dup partial FLD region, instead it could be ascribed to an increased tendency of the lectins for oligomerization.
An interesting example of how artificial lectins can be created has been reported by Ribeiro et al.99 They engineered the first chimeric, bispecific lectin, with two rationally oriented and distinct recognition surfaces, which are able to bind both fucosylated and sialylated glycoconjugates (Fig. 5). The chimeric lectin, named FS-Janus lectin, was obtained by fusing sequences from the lectin of Ralstonia solanacearum (RSL), which displays strong affinity for fucose, and a sequence from the NanI sialidase of Clostridium perfringens ATCC13124 (CBM40_NanI), with strong affinity for sialylated oligosaccharides. The binding ability towards fucose, 3′-sialyllactose (3′-SL) and 6′-sialyllactose (6′-SL) functionalised surfaces was evaluated using surface plasmon resonance. The Janus lectin showed nanomolar avidity for fucosylated and sialylated surfaces, with the latter being an important achievement since classic lectins usually display low affinity for sialylated epitopes.
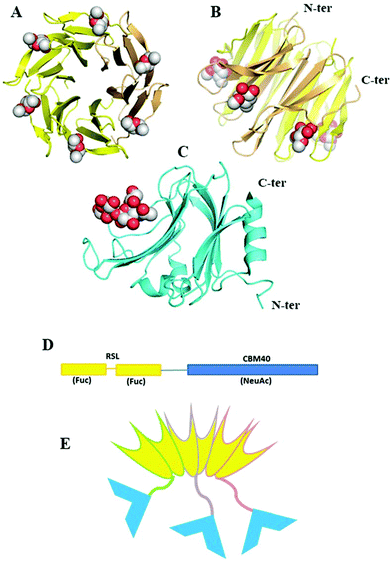 |
| Fig. 5 A Janus lectin with two rationally oriented and distinct recognition surfaces, which are able to bind independently to fucosylated and sialylated glycoconjugates. (A and B) Two views of crystal structure of RSL-trimer complexed with six fucose ligands (pdb code 2BT9). (C) Crystal structure of CBM40_NanI complexed with 30-siallylactose (pdb code 5FRE). (D) Design of the peptide sequence with two blades of RSL connected to CBM40 through a peptide linker. (E) Schematic representation of the expected FSJanus lectin presenting six fucose binding sites on the upper face and three sialic acid binding sites on the bottom face. Reproduced from ref. 99 with permission from The Royal Society of Chemistry, copyright 2018. | |
There are many challenges associated with lectin engineering, including the selection of template lectin, construction of a mutagenesis library and high-throughput screening methods. However, as these challenges are overcome, lectins with superior binding properties will broaden the range of applications.
4. Artificial receptors
For several years, efforts have focused on the design of artificial receptors that can mimic, or even outperform, the role of antibodies and lectins as carbohydrate binding entities. While many studies have targeted monosaccharides, the list of receptors for more complex systems is considerably shorter. One example is a set of ditopic diaminopyrrolic structures designed for the selective recognition of Manα(1–2)Man dimannosides.100 Analogous aminopyrrolic derivatives were able to bind the highly mannosylated HIV virial envelope glycoproteins gp120 and gp41, as evidenced by SPR studies, as well as showing antiviral activity against HIV infection of T-lymphocyte CEM cells.101 Although the aforementioned derivatives showed good binding properties in polar organic media, solubility in water was very poor, limiting their application in aqueous systems. Receptors with impressive binding abilities in water were obtained by developing polycyclic cages where parallel aromatic surfaces were connected via water-solubilising spacers. The contribution of hydrophobic interactions from the aromatic units, together with hydrogen bonding established with the spacers, not only allowed the selective recognition of disaccharides such as cellobiose,102,103 but also more complex targets like maltodextrin104 and polysaccharides.105 Other interesting examples of artificial receptors for disaccharides are cyclic peptides obtained from a dynamic combinatorial approach, which showed selectivity for trehalose.106 Moreover, polyaromatic nanocapsules were reported to exclusively bind sucrose in water.107 Interestingly, selectivity arises from the shape complementarity of the capsules, as well as the establishment of CH–π interactions with the polyaromatic framework.
The above examples provide interesting insights on some of the key features that give rise to the selective recognition of carbohydrates. However, so far, they may lack a direct application to targets of biological relevance. The rest of this review will focus on the examination of boronic acid derivatives and aptamers for the recognition of glycans, since they have been able to provide a more versatile approach for the development of synthetic receptors for applications in biological settings.
4.1 Boronic acids
Boronic acids are known for their ability to covalently bind 1,2- and 1,3-diol groups found in carbohydrates.108 The binding interaction is reversible and pH dependant. Boronic acids can readily interconvert in aqueous media from sp2 to sp3 hybridisation in the presence of a Lewis base, with the resulting tetrahedral species in equilibrium with the neutral trigonal form. The reaction with diols in alkaline aqueous solutions leads to the formation of boronate esters. These features make boronic acids interesting molecules for the realization of synthetic receptors for carbohydrate recognition, also named “boronolectins”.14,19,109–111
The chemical versatility of boronic acids enables their insertion into various scaffolds, with their integration into polymer backbones useful for a variety of biomedical applications.112 Vinylphenylboronic acids can be grafted onto suitably modified nanoparticles,113 while phenylboronic acid–adamantane conjugates have been reported to form self-assembled monolayers on the surface of cyclodextrin vesicles in aqueous solution.114 These vesicles presented multiple boronic acid receptors on their surface, which were found to bind monosaccharides with binding constants in the range of 100–3000 M−1. Moreover, a glucose selective sensor was obtained by the formation of a self-assembled monolayer of bis-boronic acids on gold surfaces.115 The well-defined intermolecular distance between the boronic acid moieties, together with a suitable spatial orientation, resulted in high selectivity for glucose over other saccharides. These, and other approaches, could enable boronic acids to find real life-applications, such as the production of probes for the sensing of sugars in commercial beverages.116,117
Whereas much of the area of sugar binding by boronic acids, in particular monosaccharide recognition and sensing, has already been well documented elsewhere,14,17,19,110,115 glycan and glycoconjugate recognition using such reports is still in its infancy. Promising results have been obtained using boronic acid-functionalized peptidic receptors that act as fluorescent sensors. In particular, diboronic acid compounds of this type could be used to recognise cell surface cancer-associated glycans in situ, such as Sialyl Lewis X (sLex), with high specificity.118,119 The peptide-based linkers connecting two anthracene–phenylboronic acid moieties were employed to improve water solubility and biocompatibility. These receptors show great potential as probes in cancer diagnosis (Fig. 6).
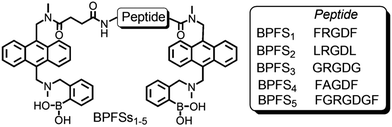 |
| Fig. 6 Molecular structures of BPFSs: peptide sequences are reported with the single-letter amino acid code. Reproduced from ref. 119 with permission from Springer Nature, copyright 2013. | |
Liposomes bearing boronic acids on their outer surface showed cell-binding ability, providing a tool for drug delivery upon binding with the glycans on the cell surface.120,121 Further studies could lead to the development of probes with applications in targeted drug delivery, cell labelling and selective imaging.
An effective way to create synthetic carbohydrate receptors is through Molecularly Imprinted Polymers (MIP) using boronic acids as functional monomers.13,122–127,167 This approach is based on the formation of a polymer network around a template molecule (i.e. a glycoprotein), creating artificial binding sites, which upon removal of the template can be occupied by their target.126 The use of boronic acids in MIPs can allow the realization of lectin-like surfaces for the specific recognition of glycoproteins, where the controlled orientation of the receptors around the glycoprotein enables the creation of epitopes for specific fragments.127–129 The approach is versatile and efficient and provides surfaces with strong affinities and high specificities. For instance, a sensor platform with a 30-fold selectivity for Prostate Specific Antigen (PSA) over other glycoproteins was realised.13 A pre-formed complex of PSA-acrylamido boronic acids was grafted onto a functionalized gold surface, providing the immobilization of the synthetic receptors in spatial arrangements specific for the target glycoprotein. In addition, functionalization of the surface around the template glycoprotein with oligoethylene glycol moieties enabled to create PSA-shaped cavities (Fig. 7). Surface plasmon resonance (SPR) binding studies showed that the nanocavities were specific for PSA, providing high affinity (Kd = 1.8 μM) and detection at nanomolar concentrations.
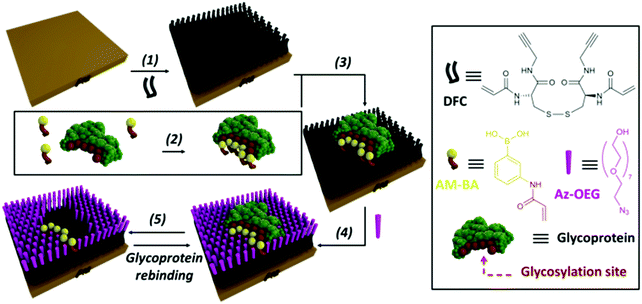 |
| Fig. 7 Strategy for the fabrication of glycoprotein-imprinted surfaces using acrylamido-boronic acids (AM-BAs); (step 1) self-assembled monolayer (SAM) formation on a gold surface with DFC molecule; (step 2) incubation of the AM-BA receptors with the template target glycoprotein; (step 3) grafting of the preformed boronic acid–glycoprotein complex on the SAM via acrylamide co-polymerization; (step 4) azide-terminated oligoethylene glycol (Az-OEG) functionalization to provide glycoprotein-shaped cavities; (step 5) removal of the template protein, affording a nanocavity specific for the target glycoprotein. Reproduced from ref. 13 with permission from The Royal Society of Chemistry, copyright 2015. | |
Recently, a strategy using boronic acid-tosyl functionalized glass slides was introduced for the generation of glycoprotein microarray platforms for glycan profiling.130 The method allows for the immobilization of glycoproteins from complex biological samples, and provides a high throughput alternative for the direct screening of glycan sequences, bypassing the use of multiple techniques and tedious sample preparation. Implementation of this approach could find real-life application in cancer diagnosis.
According to the examples reported above, the use of boronic acids seems very promising for the construction of synthetic receptors for glycan recognition. There is great potential for the development of sensor devices with applications in several areas, such as biomedical diagnostics and pharmaceutical industry. However, there are some limitations. In fact, boronic acids show good binding ability towards sugars in the furanose form,14 while most of the carbohydrates in biologically relevant molecules are in the pyranose form. Besides, the best binding constants are observed at alkaline pH values, which might not be compatible with some biological systems. Further studies should be focused on enhancing binding under physiological conditions, as well as improving glycan recognition with higher selectivities and specificities.
4.2 Benzoboroxoles
Benzoboroxoles are derivatives of phenylboronic acids with an intramolecular five membered-ring containing the boron atom. Although they were first reported in 1957,131 these compounds only started gaining greater attention in the late 2000s as their interesting biological activity and hence medicinal relevance, started to become apparent. Several compounds display antifungal action with some under clinical trials for psoriasis and human African trypanosomiasis.132,133 In addition, benzoboroxoles also show inhibitory activity against several enzymes such as carbonic anhydrases and they are potential anti-tuberculosis agents.134,135 However, this class of compound has recently drawn particular interest from their potential application as carbohydrate receptors, as they can bind sugars under physiological conditions.136 Compared to their boronic acid counterparts, benzoboroxoles are able to bind sugars in the pyranose form, at pH values that are more suitable for biological systems. This makes them attractive as potential candidates for the development of synthetic lectins for the recognition of glycans on cell surfaces and glycoproteins.
Low molecular weight peptidyl bis(benzoboroxole) receptors were reported to be able to target the cancer-related Thomsen–Friedenreich (TF) antigen.137 The receptors consisted of two benzoboroxoles for binding the two diol units of the TF antigen, and also a peptide backbone, with a tuneable length and rigidity, to provide additional hydrogen bonding and hydrophobic/CH–π interactions (Fig. 8). Even small changes in the stereochemical configuration of the peptide spacer considerably affected the binding, highlighting the extent to which molecular recognition is sensitive to subtle variations. Screening for the binding of TF antigen using a competitive ELISA assay showed that the most potent receptor exhibited a IC50 value (the concentration of inhibitor required to produce 50% inhibition of an enzymatic reaction at a specific substrate concentration) of 20 μM. Although only moderate affinities and selectivities were observed (Kd in the low millimolar range) the results are promising and encouraging for future studies.
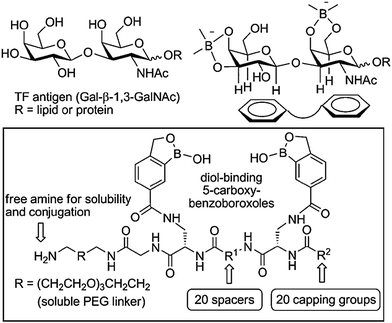 |
| Fig. 8 Design of peptidyl bis(benzoboroxoles) for the recognition of TF antigen; the library was generated by combining different spacers R1, natural and non-natural amino acids, and carboxylic acids as capping groups R2. Reproduced from ref. 137 with permission from Wiley-VCH, copyright 2010. | |
The attractive binding properties of benzoboroxoles have recently led to the development of a carbohydrate-binding ligand for the specific enrichment of glycoproteins.138 A benzoboroxole-modified ligand with a structure that mimics Trp and Phe (two amino acids frequently present in lectins) was immobilised on a Sepharose™ column. This approach allowed the purification of glycoproteins from complex mixtures at neutral pH. When glucose oxidase (GOx) was spiked into an E. coli supernatant, purification with the benzoboroxole-modified column afforded GOx with 98% purity, with the protein retaining its enzymatic activity. Thermo-responsive polymers functionalised with benzoboroxole units have also been reported as promising sensing platforms for the detection of diols and polyols (i.e. catechols, saccharides and glycopolymers) at physiologically relevant pH values.139 Moreover, benzoboroxole-containing nanoparticles have turned out to be promising options for immunological applications such as internalisation of dendritic cells and controlled antigen release.140 These nanoparticles, stable under physiological conditions, were obtained by mixing glycopolymers and a benzoboroxole-containing polymer. Their formation was promoted by the establishment of dynamic covalent bonds between the two complementary polymer chains. The study reported that the nanoparticles can be internalised by dendritic cells which then dissociate when exposed to the acidic environment of the organelles. This feature allowed the controlled release of a preloaded antigen upon internalization, opening up new opportunities for applications in cancer immunotherapy. Modified nanoparticles could also provide a tool for the qualitative screening of glycoproteins, as well as the generation of probes for cancer cell recognition.141,142 Although reaching high affinity and specificity is still a challenge, benzoboroxoles are certainly a very promising class of compounds for the development of synthetic receptors for glycans and their derivatives, which no doubt will lead to continued interest in these compounds.
In order to disclose the full potential of synthetic glycan receptors, future efforts should focus on the fine control of the spatial arrangements of the ligands. Moreover, the promotion of additional secondary interactions, such as hydrogen bonding and CH–π interactions must be considered to achieve selective molecular recognition.
4.3 Aptamers
Aptamers are synthetically derived, single stranded oligonucleotides that bind to non-oligonucleotide based targets. They are typically constructed from less than 100 bases, with sequences determined from the Systematic Evolution of Ligands by EXponential enrichment (SELEX) method, first adopted by Tuerk and Gold.143 Aptamers interact with a variety of targets, ranging from small molecules to whole cells, with similar, if not improved, affinities than antibodies.144 There is limited recent literature on aptamers targeting glycans, with most sources identifying saccharide-binding sequences from over a decade ago.145 The relative lack of new developments in this field may be due to limited options regarding non-covalent binding interactions between sugars and oligonucleotides. For example, the absence of charged groups and aromatic ring structures in simple sugars limits interactions to hydrophobic sites and hydrogen bonding.146 There have been attempts to overcome this particular problem by the use of modified aptamers. Oligonucleotides bearing boronic acid groups have been employed to promote affinities of aptamers to glycan regions of glycoproteins.147 Although there are now a few recent reports on this approach,148–150 there are no other examples of the use of any other modification designed specifically to enhance glycan–aptamer interactions.
Current advances in this area have focused mainly on aptamers that target sugars with charged moieties, such as the sialic acids. These sugars are known to be overexpressed in cancer cells and are thus ideal candidates to probe in cancer diagnostics and therapeutics.151 Kim and co-workers used the SELEX process to identify an RNA aptamer for the sialic acid, N-acetylneuraminic acid (Neu5Ac).152 They reported a high affinity (KD of 1.35 nM) and high selectivity, with very little interaction observed between the aptamer and non-Neu5Ac containing oligosaccharides. They attribute these properties to the directional immobilisation approach undertaken for the selection of the aptamer (Fig. 9). Typically, in SELEX rounds utilising solid supports, target molecules with multiple functional groups are immobilised in random orientations, leading to oligonucleotide pools recognising various epitopes of the same target but with reduced specificity towards a particular epitope. By directing the immobilisation of Neu5Ac to adopt a more natural conformation by exposing the carboxylic acid group of the sugar, the authors were able to identify an aptamer highly selective for both Neu5Ac alone and Neu5Ac modified glycans. The binding of the aptamer to Neu5Ac was also shown to prevent enzymatic hydrolysis of the sugar. In another study, six DNA aptamer sequences which specifically targeted another member of the sialic acid family, N-glycolylneuraminic acid (Neu5Gc), were identified. One of the aptamers produced a high affinity constant (KA) of 6.68 × 109 M−1.153 The technology was later developed into aptamer-nanoparticle biosensor strips for visual detection of Neu5Gc.154 Both of the examples above highlight the latest use of aptamers as novel probes targeting sialic acids, for rapid and effective sensing of this particular type of glycan.
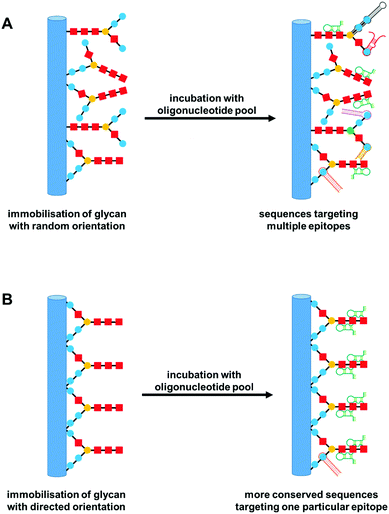 |
| Fig. 9 SELEX process used to enhance selectivity of sequences towards a particular epitope. Target glycan is immobilised to a solid support in either a random orientation (A) resulting in reduced selectivity, or a directed orientation (B) resulting in high selectivity. | |
Specific DNA aptamers have been also generated to recognise cell-surface based glycoconjugates. One such target is the cancer antigen, glycosphingolipid Globo H,155 the binding of which was enhanced by extending the strands of the aptamers, resulting in dissociation constants in the low micromolar range. The specificity of the aptamers towards Globo H was also investigated. However, the probes showed comparable affinities to oligosaccharides similar in structure to Globo H. Another target is membrane-bound N-acetylgalactosamine (GalNAc). A fluorescently tagged aptamer selected for GalNAc was used to image HeLa and Cos-7 cells with precise localization.156
Other recent developments have reported aptamers which target peptidoglycans and glycan regions of glycoproteins. For instance, RNA aptamers have been selected against two classes of glycosaminoglycans, heparosan and chondroitin.157 The non-immunogenic nature of these glycosaminoglycans had previously made their detection in biological samples using popular antibody based technologies difficult. High affinities were observed, with KD values in the range of 0.71–1.0 μM. An RNA aptamer that binds specifically to the glycosylated receptor-binding domain of a hemagglutinin protein on the surface of the influenza virus (HA1, subtype H5) has also been isolated.158 The binding of the aptamer to the glycoprotein blocked attachment of the virus to host cells, indicating a potential use of the aptamer as an antiviral reagent against influenza.
Apart from RNA aptamers, DNA aptamers that selectively bind glycan domains in glycoproteins have also been developed. For instance, DNA aptamers have been shown to target only the glycan moiety of the prostate cancer biomarker, prostate-specific antigen (PSA).159 Counter SELEX rounds were introduced to remove sequences showing affinity for non-glycosylated PSA. The selected sequences were able to discriminate human PSA from the non-glycosylated protein but exhibited comparable affinities to a similarly glycosylated protein (lipocalin-2). To overcome this problem, the authors utilised their glycan targeting PSA aptamer along with the anti-PSA aptamer (shown to bind only to protein regions of PSA) in a sandwich assay for quantification of human PSA (Fig. 10). The assay displayed enhanced selectivity for human PSA over both non-glycosylated PSA and a similarly sized glycoprotein (NGAL), with a limit of detection of 0.66 ng mL−1. While the assay was able to detect human PSA at low amounts, it was difficult to ascertain whether it could discriminate between different glycan forms of PSA, some of which are indicative of more aggressive forms of prostate cancer. Lower readings were obtained for patients with benign prostate hyperplasia compared to the antibody based ELISA, with the authors suggesting that the sensor could detect a fraction of PSA with distinct glycan structures.
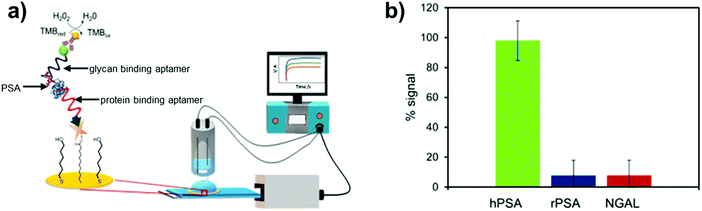 |
| Fig. 10 Prostate specific antigen (PSA) biosensor utilising both glycan and protein binding aptamers in a sandwich based sensing approach: (a) biosensor set up; (b) data showing selectivity of the sensor towards glycosylated PSA (hPSA) over the non-glycosylated version (rPSA) and a similarly sized glycoprotein (NGAL). Reproduced from ref. 159 with permission from Elsevier, copyright 2019. | |
Two peptidoglycan-targeting DNA aptamers have also been identified that displayed high affinities towards Staphylococcus aureus cells.160 The dissociation constants of the two aptamers towards peptidoglycan were 0.415 μM and 1.261 μM. It was observed that the aptamers had similar binding to both Gram negative and Gram positive bacterial cells, despite the latter typically consisting of a higher content of and more exposed peptidoglycan. The probes were selective towards bacterial cells over human fibroblasts, making them promising tools in the diagnosis of bacterial infections. DNA aptamers have also been produced against advanced glycation end (AGE) products to target macromolecules associated with age related disorders.161 Although results were promising, it was stated that further testing of the aptamers needed to be performed for safety and efficacy, before consideration for clinical use.
Although the challenges faced by aptamers mainly stem from the lack of interactions that these oligonucleotides can establish with glycans, the easy adaptation of aptamers to incorporate sugar-binding moieties such as boronic acids, to enhance interactions, is one way to overcome this limitation.147 The biggest challenge for sensing glycoconjugates using aptamers appears to be enhancing specificity towards a particular glycan target. Nevertheless, progress can be achieved by optimising the aptamer selection process (e.g. by utilising directional immobilisation and counter SELEX methods), and/or by employing multiple probes in a sandwich based sensing approach.
5. Advantages and drawbacks of natural and synthetic receptors
Approaches towards the targeting of glycans based on antibodies, lectins, boronic acid derivatives and aptamers have their own advantages and drawbacks. Various factors have to be considered when choosing the appropriate receptor system, according to the glycan being targeted and the application for which it is intended. The extended binding sites of antibodies provide opportunities for enhanced affinities and specificities for glycan targets. However, these improved binding properties are currently underexploited due to major technical challenges associated with the poor immunogenicity of glycans and the lack of high-throughput methods to evaluate the glycan binding properties of newly generated antibodies. Nevertheless, high-throughput analysis methods are available by means of lectin microarrays, yielding information about the glycosylation state of glycoconjugates, with potential applications in the development of biomarkers. Moreover, despite usually showing low binding affinities and poor selectivity for glycans that share the same extremity, lectins can display higher affinities when assembled into oligomeric structures.
Thanks to their chemical versatility that allows for the introduction of various functional groups, boronic acid derivatives can be incorporated into different scaffolds, namely surfaces, nanoparticles or vesicles, unlocking new opportunities for the creation of sensing devices with potential applications in diagnostics. A major drawback arises from the strong pH dependence of the binding, which is favoured at alkaline pH. In this regard, benzoboroxoles can overcome the issue since they are able to bind sugars under physiological conditions, therefore being more compatible with biological targets. Although selectivity can be achieved, binding constants are usually in the low millimolar/micromolar range. Small changes in the structure of the receptor can affect the binding significantly, hence the accurate tuning of the spatial orientation of the binding units is essential.
Aptamers, like boronic acid derivatives, possess heightened stability and amenability to chemical modification compared to antibodies and lectins, whilst displaying similar or even higher affinities for glycan targets. Current research appears to be focused on the detection of specific glycan-containing biomarkers, including the sialic acid family and various glycoproteins such as PSA, for disease diagnostics and therapeutics. Future efforts should be dedicated to tackling the selectivity challenge concerning aptamer technology, improving the so far limited techniques that aim at tweaking the aptamer selection process to focus on particular epitopes and exclude sequences that bind molecules similar in structure to the target glycan.
All the glycan-binding entities described in this review show great potential, although there are still important challenges that must be tackled in order to successfully achieve specific glycan recognition. Research on antibodies is moving towards the rational design of glycan derivatives to improve immunogenicity, and glycan arrays to speed up antibody screening and advances in carbohydrate chemistry to create more complex glycans. However, antibody production still remains a complicated and costly procedure and, thus, in many settings, lectins, boronic acid-based entities and aptamers represent highly suitable and attractive alternatives. Many efforts are currently focussed on lectin engineering and despite the several difficulties associated with the creation of artificial lectins, recent studies have shown that superior affinities for glycans can be achieved. Moreover, for both boronic acid derivatives and aptamers, the identification and introduction of functionalities able to establish additional interactions with the substrate of interest will be crucial for the development of more effective receptors. Due to the vast amount of hydroxyl groups, hydrogen bonding is probably the most sought-after type of interaction,15,20,100,162 followed by CH–π interactions between glycan backbones and aromatic molecules.107,163 Structural features (i.e. the size of the aromatic substituents or the presence of electron-rich groups) can have a significant impact on the stability of the stacking complexes.164 Finally, the establishment of electrostatic contacts in the appropriate positions can afford enhanced binding affinities, particularly with charged molecules such as glycosaminoglycans.165,166 As technology continues to advance, progress is expected in all these areas of research.
6. Summary and outlook
Glycans are of great importance in signifying disease states and in fundamental biological processes. The diversity of possible monosaccharide combinations, linkages and modifications, require a variety of strategies to achieve the selective recognition of biomedically relevant glycans and their derivatives. In this regard, various approaches, based on antibodies, lectins, aptamers and boronic acid derivatives, have been pursued. While these approaches already contribute to cancer biomarker research, diagnostics and therapeutics, the development of new and better glycan binding entities would be invaluable, coupled with better tools to investigate these targets in various biological settings, including in vitro and in vivo conditions. Furthermore, there is the need for more powerful high-throughput screening methods (i.e. fluorescence methods, UV and SPR) and efficient synthetic methodologies for the synthesis of complex glycans in high yield. Finally, a common major challenge across natural and synthetic receptors is enhancing the selectivity of the receptor towards a specific glycan target. Thus, the development of new glycan binding entities will continue to be a high priority for the foreseeable future.
Conflicts of interest
There are no conflicts to declare.
Acknowledgements
The authors acknowledge the financial support of this work by the EPSRC (EP/K027263/1) and ERC (consolidator grant 614787).
References
-
J. Rini, J. Esko and A. Varki, Glycosyltransferases and Glycan-processing Enzymes, in Essentials of Glycobiology, ed. Varki A., Cummings R. D. and Esko J. D, Cold Spring Harbor, New York, 2nd edn, 2009 Search PubMed.
- K. S. Lau, E. A. Partridge, A. Grigorian, C. I. Silvescu, V. N. Reinhold, M. Demetriou and J. W. Dennis, Complex N-glycan number and degree of branching cooperate to regulate cell proliferation and differentiation, Cell, 2007, 129, 123–134 CrossRef CAS.
- Y. Tian and H. Zhang, Glycoproteomics and clinical applications, Proteomics. Clin. Appl., 2010, 4, 124–132 CrossRef CAS PubMed.
- H. Hwang, J. Zhang, K. A. Chung, J. B. Leverenz, C. P. Zabetian, E. R. Peskind, J. Jankovic, Z. Su, A. M. Hancock, C. Pan, T. J. Montine, S. Pan, J. Nutt, R. Albin, M. Gearing, R. P. Beyer, M. Shi and J. Zhang, Glycoproteomics in neurodegenerative diseases, Mass Spectrom. Rev., 2010, 29, 79–125 CAS.
- J. B. Lowe and J. D. Marth, A genetic approach to Mammalian glycan function, Annu. Rev. Biochem., 2003, 72, 643–691 CrossRef CAS.
- B. Adamczyk, T. Tharmalingam and P. M. Rudd, Glycans as cancer biomarkers, Biochim. Biophys. Acta, Gen. Subj., 2012, 1820, 1347–1353 CrossRef CAS.
- J. E. Hudak and C. R. Bertozzi, Glycotherapy: New Advances Inspire a Reemergence of Glycans in Medicine, Chem. Biol., 2014, 21, 16–37 CrossRef CAS.
- P. M. Lanctot, F. H. Gage and A. P. Varki, The glycans of stem cells, Curr. Opin. Chem. Biol., 2007, 11, 373–380 CrossRef CAS PubMed.
- A. Vasconcelos-dos-Santos, I. A. Oliveira, M. C. Lucena, N. R. Mantuano, S. A. Whelan, W. B. Dias and A. R. Todeschini, Biosynthetic machinery involved in aberrant glycosylation: promising targets for developing of drugs against cancer, Front. Oncol., 2015, 5, 138 Search PubMed.
- J. Heimburg-Molinaro, M. Lum, G. Vijay, M. Jain, A. Almogren and K. Rittenhouse-Olson, Cancer vaccines and carbohydrate epitopes, Vaccine, 2011, 29, 8802–8826 CrossRef CAS.
- P. Syed, K. Gidwani, H. Kekki, J. Leivo, K. Pettersson and U. Lamminmaki, Role of lectin microarrays in cancer diagnosis, Proteomics, 2016, 16, 1257–1265 CrossRef CAS.
- W. Sun, L. Du and M. Li, Aptamer-based carbohydrate recognition, Curr. Pharm. Des., 2010, 16, 2269–2278 CrossRef CAS PubMed.
- A. Stephenson-Brown, A. L. Acton, J. A. Preece, J. S. Fossey and P. M. Mendes, Selective glycoprotein detection through covalent templating and allosteric click-imprinting, Chem. Sci., 2015, 6, 5114–5119 RSC.
- X. Wu, Z. Li, X. X. Chen, J. S. Fossey, T. D. James and Y. B. Jiang, Selective sensing of saccharides using simple boronic acids and their aggregates, Chem. Soc. Rev., 2013, 42, 8032–8048 RSC.
- R. A. Tromans, T. S. Carter, L. Chabanne, M. P. Crump, H. Li, J. V. Matlock, M. G. Orchard and A. P. Davis, A biomimetic receptor for glucose, Nat. Chem., 2019, 11, 52–56 CrossRef CAS PubMed.
- O. Francesconi and S. Roelens, Biomimetic Carbohydrate-Binding Agents (CBAs): Binding Affinities and Biological Activities, ChemBioChem, 2019, 20, 1329–1346 CrossRef CAS PubMed.
- L. E. Guo, Y. Hong, S. Y. Zhang, M. Zhang, X. S. Yan, J. L. Cao, Z. Li, T. D. James and Y. B. Jiang, Proline-Based Boronic Acid Receptors for Chiral Recognition of Glucose, J. Org. Chem., 2018, 83, 15128–15135 CrossRef CAS PubMed.
- P. Rios, T. J. Mooibroek, T. S. Carter, C. Williams, M. R. Wilson, M. P. Crump and A. P. Davis, Enantioselective carbohydrate recognition by synthetic lectins in water, Chem. Sci., 2017, 8, 4056–4061 RSC.
- T. D. James, K. R. A. S. Sandanayake and S. Shinkai, Saccharide sensing with molecular receptors based on boronic acid, Angew. Chem., Int. Ed. Engl., 1996, 35, 1910–1922 CrossRef.
- C. Nativi, M. Cacciarini, O. Francesconi, A. Vacca, G. Moneti, A. Ienco and S. Roelens, Pyrrolic tripodal receptors effectively recognizing monosaccharides. Affinity assessment through a generalized binding descriptor, J. Am. Chem. Soc., 2007, 129, 4377–4385 CrossRef CAS PubMed.
- E. Sterner, N. Flanagan and J. C. Gildersleeve, Perspectives on Anti-Glycan Antibodies Gleaned from Development of a Community Resource Database, ACS Chem. Biol., 2016, 11, 1773–1783 CrossRef CAS PubMed.
- N. Bovin, P. Obukhova, N. Shilova, E. Rapoport, I. Popova, M. Navakouski, C. Unverzagt, M. Vuskovic and M. Huflejt, Repertoire of human natural anti-glycan immunoglobulins. Do we have auto-antibodies?, Biochim. Biophys. Acta, 2012, 1820, 1373–1382 CrossRef CAS PubMed.
- R. G. Wang, M. Ruan, R. J. Zhang, L. Chen, X. X. Li, B. Fang, C. Li, X. Y. Ren, J. Y. Liu, Q. Xiong, L. N. Zhang, Y. Jin, L. Li, R. Li, Y. Wang, H. Y. Yang and Y. F. Dai, Antigenicity of tissues and organs from GGTA1/CMAH/beta4GalNT2 triple gene knockout pigs, J. Biomed. Res., 2018 DOI:10.7555/JBR.32.20180018 [Epub ahead of print].
- H. Xu, D. Yin, B. Naziruddin, L. Chen, A. Stark, Y. Wei, Y. Lei, J. Shen, J. S. Logan, G. W. Byrne and A. S. F. Chong, The In Vitro and In Vivo Effects of Anti-Galactose Antibodies on Endothelial Cell Activation and Xenograft Rejection, J. Immunol., 2003, 170, 1531–1539 CrossRef CAS PubMed.
- J. L. Estrada, G. Martens, P. Li, A. Adams, K. A. Newell, M. L. Ford, J. R. Butler, R. Sidner, M. Tector and J. Tector, Evaluation of human and non-human primate antibody binding to pig cells lacking GGTA1/CMAH/beta4GalNT2 genes, Xenotransplantation, 2015, 22, 194–202 CrossRef.
- S. Makeneni, Y. Ji, D. C. Watson, N. M. Young and R. J. Woods, Predicting the Origins of Anti-Blood Group Antibody Specificity: A Case Study of the ABO A- and B-Antigens, Front. Immunol., 2014, 5, 397 Search PubMed.
- N. Dotan, R. Altstock, M. Schwarz and A. Dukler, Anti-Glycan Antibodies as Biomarkers for Diagnosis and Prognosis, Lupus, 2006, 15, 442–450 CrossRef CAS PubMed.
- H. H. Wandall, O. Blixt, M. A. Tarp, J. W. Pedersen, E. P. Bennett, U. Mandel, G. Ragupathi, P. O. Livingston, M. A. Hollingsworth, J. Taylor-Papadimitriou, J. Burchell and H. Clausen, Cancer biomarkers defined by autoantibody signatures to aberrant O-glycopeptide epitopes, Cancer Res., 2010, 70, 1306–1313 CrossRef CAS PubMed.
- D. L. Meany and D. W. Chan, Aberrant glycosylation associated with enzymes as cancer biomarkers, Clin. Proteomics, 2011, 8, 7 CrossRef CAS PubMed.
- C. Pifferi, B. Thomas, D. Goyard, N. Berthet and O. Renaudet, Heterovalent Glycodendrimers as Epitope Carriers for Antitumor Synthetic Vaccines, Chemistry, 2017, 23, 16283–16296 CrossRef CAS PubMed.
- Q. Qin, Z. Yin, X. Wu, K. M. Haas and X. Huang, Valency and density matter: deciphering impacts of immunogen structures on immune responses against a tumor associated carbohydrate antigen using synthetic glycopolymers, Biomaterials, 2016, 101, 189–198 CrossRef CAS PubMed.
- R. D. Astronomo and D. R. Burton, Carbohydrate vaccines: developing sweet solutions to sticky situations?, Nat. Rev. Drug Discovery, 2010, 9, 308–324 CrossRef CAS PubMed.
- O. Haji-Ghassemi, R. J. Blackler, N. Martin Young and S. V. Evans, Antibody recognition of carbohydrate epitopesdagger, Glycobiology, 2015, 25, 920–952 CrossRef CAS PubMed.
- Z. Polonskaya, S. Deng, A. Sarkar, L. Kain, M. Comellas-Aragones, C. S. McKay, K. Kaczanowska, M. Holt, R. McBride, V. Palomo, K. M. Self, S. Taylor, A. Irimia, S. R. Mehta, J. M. Dan, M. Brigger, S. Crotty, S. P. Schoenberger, J. C. Paulson, I. A. Wilson, P. B. Savage, M. G. Finn and L. Teyton, T cells control the generation of nanomolar-affinity anti-glycan antibodies, J. Clin. Invest., 2017, 127, 1491–1504 CrossRef PubMed.
- E. Kaltgrad, S. Sen Gupta, S. Punna, C. Y. Huang, A. Chang, C. H. Wong, M. G. Finn and O. Blixt, Anti-carbohydrate antibodies elicited by polyvalent display on a viral scaffold, ChemBioChem, 2007, 8, 1455–1462 CrossRef CAS.
- C. R. MacKenzie, T. Hirama, S. J. Deng, D. R. Bundle, S. A. Narang and N. M. Young, Analysis by surface plasmon resonance of the influence of valence on the ligand binding affinity and kinetics of an anti-carbohydrate antibody, J. Biol. Chem., 1996, 271, 1527–1533 CrossRef CAS.
- J. J. Lundquist and E. J. Toone, The cluster glycoside effect, Chem. Rev., 2002, 102, 555–578 CrossRef CAS PubMed.
- M. Mammen, S. K. Choi and G. M. Whitesides, Polyvalent Interactions in Biological Systems: Implications for Design and Use of Multivalent Ligands and Inhibitors, Angew. Chem., Int. Ed., 1998, 37, 2754–2794 CrossRef.
- D. A. Calarese, C. N. Scanlan, M. B. Zwick, S. Deechongkit, Y. Mimura, R. Kunert, P. Zhu, M. R. Wormald, R. L. Stanfield, K. H. Roux, J. W. Kelly, P. M. Rudd, R. A. Dwek, H. Katinger, D. R. Burton and I. A. Wilson, Antibody domain exchange is an immunological solution to carbohydrate cluster recognition, Science, 2003, 300, 2065–2071 CrossRef CAS PubMed.
- B. Hoorelbeke, T. van Montfort, J. Xue, P. J. LiWang, H. Tanaka, Y. Igarashi, E. J. Van Damme, R. W. Sanders and J. Balzarini, HIV-1 envelope trimer has similar binding characteristics for carbohydrate-binding agents as monomeric gp120, FEBS Lett., 2013, 587, 860–866 CrossRef CAS.
- T. Kubota, T. Matsushita, R. Niwa, I. Kumagai and K. Nakamura, Novel anti-Tn single-chain Fv-Fc fusion proteins derived from immunized phage library and antibody Fc domain, Anticancer Res., 2010, 30, 3397–3405 CAS.
- N. Yuasa, T. Koyama and Y. Fujita-Yamaguchi, Purification and refolding of anti-T-antigen single chain antibodies (scFvs) expressed in Escherichia coli as inclusion bodies, BioSci. Trends, 2014, 8, 24–31 CrossRef CAS.
- A. Stewart, Y. Y. Liu and J. R. Lai, A strategy for phage display selection of functional domain-exchanged immunoglobulin scaffolds with high affinity for glycan targets, J. Immunol. Methods, 2012, 376, 150–155 CrossRef CAS PubMed.
- G. P. Smith, Filamentous Fusion Phage – Novel Expression Vectors That Display Cloned Antigens on the Virion Surface, Science, 1985, 228, 1315–1317 CrossRef CAS PubMed.
- D. R. Bowley, T. M. Jones, D. R. Burton and R. A. Lerner, Libraries against libraries for combinatorial selection of replicating antigen-antibody pairs, Proc. Natl. Acad. Sci. U. S. A., 2009, 106, 1380–1385 CrossRef CAS.
- J. Bazan, I. Calkosinski and A. Gamian, Phage display--a powerful technique for immunotherapy: 1. Introduction and potential of therapeutic applications, Hum. Vaccines Immunother., 2012, 8, 1817–1828 CrossRef CAS PubMed.
- T. Y. Lin and J. R. Lai, Interrogation of side chain biases for oligomannose recognition by antibody 2G12 via structure-guided phage display libraries, Bioorg. Med. Chem., 2017, 25, 5790–5798 CrossRef CAS.
- Z. Polonskaya, S. Deng, A. Sarkar, L. Kain, M. Comellas-Aragones, C. S. McKay, K. Kaczanowska, M. Holt, R. McBride, V. Palomo, K. M. Self, S. Taylor, A. Irimia, S. R. Mehta, J. M. Dan, M. Brigger, S. Crotty, S. P. Schoenberger, J. C. Paulson, I. A. Wilson, P. B. Savage, M. G. Finn and L. Teyton, T cells control the generation of nanomolar-affinity anti-glycan antibodies, J. Clin. Invest., 2017, 127, 1491–1504 CrossRef PubMed.
- Z. Polonskaya, P. B. Savage, M. G. Finn and L. Teyton, High-affinity anti-glycan antibodies: challenges and strategies, Curr. Opin. Immunol., 2019, 59, 65–71 CrossRef CAS PubMed.
- M. Cygler, Recognition of carbohydrates by antibodies, Res. Immunol., 1994, 145, 36–40 CrossRef CAS PubMed.
- S. Villeneuve, H. Souchon, M. M. Riottot, J. C. Mazie, P. Lei, C. P. Glaudemans, P. Kovac, J. M. Fournier and P. M. Alzari, Crystal structure of an anti-carbohydrate antibody directed against Vibrio cholerae O1 in complex with antigen: molecular basis for serotype specificity, Proc. Natl. Acad. Sci. U. S. A., 2000, 97, 8433–8438 CrossRef CAS PubMed.
- O. Haji-Ghassemi, S. Muller-Loennies, R. Saldova, M. Muniyappa, L. Brade, P. M. Rudd, D. J. Harvey, P. Kosma, H. Brade and S. V. Evans, Groove-type recognition of chlamydiaceae-specific lipopolysaccharide antigen by a family of antibodies possessing an unusual variable heavy chain N-linked glycan, J. Biol. Chem., 2014, 289, 16644–16661 CrossRef CAS PubMed.
- N. Yuasa, T. Koyama, G. P. Subedi, Y. Yamaguchi, M. Matsushita and Y. Fujita-Yamaguchi, Expression and structural characterization of anti-T-antigen single-chain antibodies (scFvs) and analysis of their binding to T-antigen by surface plasmon resonance and NMR spectroscopy, J. Biochem., 2013, 154, 521–529 CrossRef CAS PubMed.
- P. Lak, S. Makeneni, R. J. Woods and T. L. Lowary, Specificity of Furanoside-Protein Recognition through Antibody Engineering and Molecular Modeling, Chem. – Eur. J., 2015, 21, 1138–1148 CrossRef CAS PubMed.
- S. Muller-Loennies, C. R. MacKenzie, S. I. Patenaude, S. V. Evans, P. Kosma, H. Brade, L. Brade and S. Narang, Characterization of high affinity monoclonal antibodies specific for chlamydial lipopolysaccharide, Glycobiology, 2000, 10, 121–130 CrossRef CAS.
- H. Coelho, T. Matsushita, G. Artigas, H. Hinou, F. J. Canada, R. Lo-Man, C. Leclerc, E. J. Cabrita, J. Jimenez-Barbero, S. Nishimura, F. Garcia-Martin and F. Marcelo, The Quest for Anticancer Vaccines: Deciphering the Fine-Epitope Specificity of Cancer-Related Monoclonal Antibodies by Combining Microarray Screening and Saturation Transfer Difference NMR, J. Am. Chem. Soc., 2015, 137, 12438–12441 CrossRef CAS PubMed.
- S. Rangappa, G. Artigas, R. Miyoshi, Y. Yokoi, S. Hayakawa, F. Garcia-Martin, H. Hinou and S. I. Nishimura, Effects of the multiple O-glycosylation states on antibody recognition of the immunodominant motif in MUC1 extracellular tandem repeats, MedChemComm, 2016, 7, 1102–1122 RSC.
- S. Müller-Loennies, C. R. MacKenzie, S. I. Patenaude, S. V. Evans, P. Kosma, H. Brade, L. Brade and S. Narang, Characterization of high affinity monoclonal antibodies specific for chlamydial lipopolysaccharide, Glycobiology, 2000, 10, 121–130 CrossRef PubMed.
- N. Sinha, S. Mohan, C. A. Lipschultz and S. J. Smith-Gill, Differences in Electrostatic Properties at Antibody–Antigen Binding Sites: Implications for Specificity and Cross-Reactivity, Biophys. J., 2002, 83, 2946–2968 CrossRef CAS PubMed.
- K. Yoshida, D. Kuroda, M. Kiyoshi, M. Nakakido, S. Nagatoishi, S. Soga, H. Shirai and K. Tsumoto, Exploring designability of electrostatic complementarity at an antigen-antibody interface directed by mutagenesis, biophysical analysis, and molecular dynamics simulations, Sci. Rep., 2019, 9, 4482 CrossRef PubMed.
- S. Park, J. C. Gildersleeve, O. Blixt and I. Shin, Carbohydrate microarrays, Chem. Soc. Rev., 2013, 42, 4310–4326 RSC.
- J. L. de Paz and P. H. Seeberger, Recent advances and future challenges in glycan microarray technology, Methods Mol. Biol., 2012, 808, 1–12 CrossRef CAS.
- M. S. Pereira, I. Alves, M. Vicente, A. Campar, M. C. Silva, N. A. Padrao, V. Pinto, A. Fernandes, A. M. Dias and S. S. Pinho, Glycans as Key Checkpoints of T Cell Activity and Function, Front. Immunol., 2018, 9, 2754 CrossRef.
- L. G. Karacosta, J. C. Fisk, J. Jessee, S. Tati, B. Turner, D. Ghazal, R. Ludwig, H. Johnson, J. Adams, M. Sajjad, S. Koury, R. Roy, J. R. Olson and K. Rittenhouse-Olson, Preclinical Analysis of JAA-F11, a Specific Anti-Thomsen-Friedenreich Antibody via Immunohistochemistry and In Vivo Imaging, Transl. Oncol., 2018, 11, 450–466 CrossRef.
- M. Jeyakanthan, P. J. Meloncelli, L. Zou, T. L. Lowary, I. Larsen, S. Maier, K. Tao, J. Rusch, R. Chinnock, N. Shaw, M. Burch, K. Beddows, L. Addonizio, W. Zuckerman, E. Pahl, J. Rutledge, K. R. Kanter, C. W. Cairo, J. M. Buriak, D. Ross, I. Rebeyka and L. J. West, ABH-Glycan Microarray Characterizes ABO Subtype Antibodies: Fine Specificity of Immune Tolerance After ABO-Incompatible Transplantation, Am. J. Transplant., 2016, 16, 1548–1558 CrossRef CAS PubMed.
- G. Ragupathi, P. Damani, G. Srivastava, O. Srivastava, S. J. Sucheck, Y. Ichikawa and P. O. Livingston, Synthesis of sialyl Lewis(a) (sLe (a), CA19-9) and construction of an immunogenic sLe(a) vaccine, Cancer Immunol. Immunother., 2009, 58, 1397–1405 CrossRef CAS PubMed.
- R. C. Dolscheid-Pommerich, M. Keyver-Paik, T. Hecking, W. Kuhn, G. Hartmann, B. Stoffel-Wagner and S. Holdenrieder, Clinical performance of LOCI-based tumor marker assays for tumor markers CA 15-3, CA 125, CEA, CA 19-9 and AFP in gynecological cancers, Tumour Biol., 2017, 39 DOI:10.1177/1010428317730246.
- R. Sawada, S. M. Sun, X. H. Wu, F. Hong, G. Ragupathi, P. O. Livingston and W. W. Scholz, Human Monoclonal Antibodies to Sialyl-Lewis(a) (CA19.9) with Potent CDC, ADCC, and Antitumor Activity, Clin. Cancer Res., 2011, 17, 1024–1032 CrossRef CAS PubMed.
- J. Hirabayashi, M. Yamada, A. Kuno and H. Tateno, Lectin microarrays: concept, principle and applications, Chem. Soc. Rev., 2013, 42, 4443–4458 RSC.
- R. Mody, S. H. A. Joshi and W. Chaney, Use of lectins as diagnostic and therapeutic tools for cancer, J. Pharmacol. Toxicol. Methods, 1995, 33, 1–10 CrossRef CAS.
- Y. Feng, Y. Guo, Y. Li, J. Tao, L. Ding, J. Wu and H. Ju, Lectin-mediated in situ rolling circle amplification on exosomes for probing cancer-related glycan pattern, Anal. Chim. Acta, 2018, 1039, 108–115 CrossRef CAS.
- W. I. Weis and K. Drickamer, Structural basis of lectin-carbohydrate recognition, Annu. Rev. Biochem., 1996, 65, 441–473 CrossRef CAS.
- A. Dessen, D. Gupta, S. Sabesan, C. F. Brewer and J. C. Sacchettini, X-ray crystal structure of the soybean agglutinin cross-linked with a biantennary analog of the blood group I carbohydrate antigen, Biochemistry, 1995, 34, 4933–4942 CrossRef CAS PubMed.
- S. R. Stowell, C. M. Arthur, P. Mehta, K. A. Slanina, O. Blixt, H. Leffler, D. F. Smith and R. D. Cummings, Galectin-1, -2, and -3 exhibit differential recognition of sialylated glycans and blood group antigens, J. Biol. Chem., 2008, 283, 10109–10123 CrossRef CAS PubMed.
- C. A. Bewley and S. Otero-Quintero, The Potent Anti-HIV Protein Cyanovirin-N Contains Two Novel Carbohydrate Binding Sites That Selectively Bind to Man8D1D3 and Man9with Nanomolar Affinity: Implications for Binding to the HIV Envelope Protein gp120, J. Am. Chem. Soc., 2001, 123, 3892–3902 CrossRef CAS.
- F. Teillet, B. Dublet, J. P. Andrieu, C. Gaboriaud, G. J. Arlaud and N. M. Thielens, The two major oligomeric forms of human mannan-binding lectin: chemical characterization, carbohydrate-binding properties, and interaction with MBL-associated serine proteases, J. Immunol., 2005, 174, 2870–2877 CrossRef CAS.
- M. Wimmerova, S. Kozmon, I. Necasova, S. K. Mishra, J. Komarek and J. Koca, Stacking interactions between carbohydrate and protein quantified by combination of theoretical and experimental methods, PLoS One, 2012, 7, e46032 CrossRef CAS.
- F. A. Quiocho, Carbohydrate-binding proteins: tertiary structures and protein-sugar interactions, Annu. Rev. Biochem., 1986, 55, 287–315 CrossRef CAS PubMed.
- K. Madhusudan Makwana and R. Mahalakshmi, Implications of aromatic–aromatic interactions: from protein structures to peptide models, Protein Sci., 2015, 24, 1920–1933 CrossRef CAS PubMed.
- N. Srinivasan, M. Wimmerová, S. Kozmon, I. Nečasová, S. K. Mishra, J. Komárek and J. Koča, Stacking Interactions between Carbohydrate and Protein Quantified by Combination of Theoretical and Experimental Methods, PLoS One, 2012, 7, e46032 CrossRef.
- C. E. Faller and O. Guvench, Terminal sialic acids on CD44 N-glycans can block hyaluronan binding by forming competing intramolecular contacts with arginine sidechains, Proteins, 2014, 82, 3079–3089 CrossRef CAS PubMed.
- J. M. Propster, F. Yang, S. Rabbani, B. Ernst, F. H. Allain and M. Schubert, Structural basis for sulfation-dependent self-glycan recognition by the human immune-inhibitory receptor Siglec-8, Proc. Natl. Acad. Sci. U. S. A., 2016, 113, E4170–E4179 CrossRef CAS.
- W. I. Weis, R. Kahn, R. Fourme, K. Drickamer and W. A. Hendrickson, Structure of the calcium-dependent lectin domain from a rat mannose-binding protein determined by MAD phasing, Science, 1991, 254, 1608–1615 CrossRef CAS.
- K. Drickamer, Two distinct classes of carbohydrate-recognition domains in animal lectins, J. Biol. Chem., 1988, 263, 9557–9560 CAS.
- E. P. McGreal, M. Rosas, G. D. Brown, S. Zamze, S. Y. Wong, S. Gordon, L. Martinez-Pomares and P. R. Taylor, The carbohydrate-recognition domain of Dectin-2 is a C-type lectin with specificity for high mannose, Glycobiology, 2006, 16, 422–430 CrossRef CAS PubMed.
- S. Saijo, S. Ikeda, K. Yamabe, S. Kakuta, H. Ishigame, A. Akitsu, N. Fujikado, T. Kusaka, S. Kubo, S. H. Chung, R. Komatsu, N. Miura, Y. Adachi, N. Ohno, K. Shibuya, N. Yamamoto, K. Kawakami, S. Yamasaki, T. Saito, S. Akira and Y. Iwakura, Dectin-2 recognition of alpha-mannans and induction of Th17 cell differentiation is essential for host defense against Candida albicans, Immunity, 2010, 32, 681–691 CrossRef CAS PubMed.
- L. Zhang, S. Luo and B. Zhang, The use of lectin microarray for assessing glycosylation of therapeutic proteins, MAbs, 2016, 8, 524–535 CrossRef CAS PubMed.
- S. Hu and D. T. Wong, Lectin microarray, Proteomics: Clin. Appl., 2009, 3, 148–154 CAS.
- Y. Guo, H. Yu, Y. Zhong, Y. He, X. Qin, Y. Qin, Y. Zhou, P. Zhang, Y. Zhang, Z. Li and Z. Jia, Lectin microarray and mass spectrometric analysis of hepatitis C proteins reveals N-linked glycosylation, Medicine, 2018, 97, e0208 CrossRef CAS PubMed.
- D. Iizuka, S. Izumi, F. Suzuki and K. Kamiya, Analysis of a lectin microarray identifies altered sialylation of mouse serum glycoproteins induced by whole-body radiation exposure, J. Radiat. Res., 2019, 60, 189–196 CrossRef PubMed.
- A. Matsuda, A. Kuno, T. Nakagawa, Y. Ikehara, T. Irimura, M. Yamamoto, Y. Nakanuma, E. Miyoshi, S. Nakamori, H. Nakanishi, C. Viwatthanasittiphong, P. Srivatanakul, M. Miwa, J. Shoda and H. Narimatsu, Lectin Microarray-Based Sero-Biomarker Verification Targeting Aberrant O-Linked Glycosylation on Mucin 1, Anal. Chem., 2015, 87, 7274–7281 CrossRef CAS PubMed.
- J. Hirabayashi and R. Arai, Lectin engineering: the possible and the actual, Interface Focus, 2019, 9, 20180068 CrossRef.
- M. D. Swanson, D. M. Boudreaux, L. Salmon, J. Chugh, H. C. Winter, J. L. Meagher, S. André, P. V. Murphy, S. Oscarson, R. Roy, S. King, M. H. Kaplan, I. J. Goldstein, E. B. Tarbet, B. L. Hurst, D. F. Smee, C. de la Fuente, H.-H. Hoffmann, Y. Xue, C. M. Rice, D. Schols, J. V. Garcia, J. A. Stuckey, H.-J. Gabius, H. M. Al-Hashimi and D. M. Markovitz, Engineering a Therapeutic Lectin by Uncoupling Mitogenicity from Antiviral Activity, Cell, 2015, 163, 746–758 CrossRef CAS PubMed.
- K. Yamamoto, Y. Konami and T. Osawa, A chimeric lectin formed from Bauhinia purpurea lectin and Lens culinaris lectin recognizes a unique carbohydrate structure, J. Biochem., 2000, 127, 129–135 CrossRef CAS PubMed.
- Z. Kriz, J. Adam, J. Mrazkova, P. Zotos, T. Chatzipavlou, M. Wimmerova and J. Koca, Engineering the Pseudomonas aeruginosa II lectin: designing mutants with changed affinity and specificity, J. Comput.-Aided Mol. Des., 2014, 28, 951–960 CrossRef CAS PubMed.
- A. Matsumoto and Y. Miyahara, ‘Borono-lectin’ based engineering as a versatile platform for biomedical applications, Sci. Technol. Adv. Mater., 2018, 19, 18–30 CrossRef CAS PubMed.
- D. Hu, H. Tateno and J. Hirabayashi, Lectin engineering, a molecular evolutionary approach to expanding the lectin utilities, Molecules, 2015, 20, 7637–7656 CrossRef CAS PubMed.
- S. Mahajan and T. N. C. Ramya, Nature-inspired engineering of an F-type lectin for increased binding strength, Glycobiology, 2018, 28, 933–948 CAS.
- J. P. Ribeiro, S. Villringer, D. Goyard, L. Coche-Guerente, M. Höferlin, O. Renaudet, W. Römer and A. Imberty, Tailor-made Janus lectin with dual avidity assembles glycoconjugate multilayers and crosslinks protocells, Chem. Sci., 2018, 9, 7634–7641 RSC.
- O. Francesconi, C. Nativi, G. Gabrielli, M. Gentili, M. Palchetti, B. Bonora and S. Roelens, Pyrrolic Tripodal Receptors for the Molecular Recognition of Carbohydrates: Ditopic Receptors for Dimannosides, Chem. – Eur. J., 2013, 19, 11742–11752 CrossRef CAS PubMed.
- O. Francesconi, C. Nativi, G. Gabrielli, I. De Simone, S. Noppen, J. Balzarini, S. Liekens and S. Roelens, Antiviral Activity of Synthetic Aminopyrrolic Carbohydrate Binding Agents: Targeting the Glycans of Viral gp120 to Inhibit HIV Entry, Chemistry, 2015, 21, 10089–10093 CrossRef CAS PubMed.
- Y. Ferrand, M. P. Crump and A. P. Davis, A synthetic lectin analog for biomimetic disaccharide recognition, Science, 2007, 318, 619–622 CrossRef CAS PubMed.
- B. Sookcharoenpinyo, E. Klein, Y. Ferrand, D. B. Walker, P. R. Brotherhood, C. Ke, M. P. Crump and A. P. Davis, High-Affinity Disaccharide Binding by Tricyclic Synthetic Lectins, Angew. Chem., Int. Ed., 2012, 51, 4586–4590 CrossRef CAS PubMed.
- P. Stewart, C. M. Renney, T. J. Mooibroek, S. Ferheen and A. P. Davis, Maltodextrin recognition by a macrocyclic synthetic lectin, Chem. Commun., 2018, 54, 8649–8652 RSC.
- T. J. Mooibroek, J. M. Casas-Solvas, R. L. Harniman, C. M. Renney, T. S. Carter, M. P. Crump and A. P. Davis, A threading receptor for polysaccharides, Nat. Chem., 2016, 8, 69–74 CrossRef CAS PubMed.
- M. Rauschenberg, S. Bomke, U. Karst and B. J. Ravoo, Dynamic Peptides as Biomimetic Carbohydrate Receptors, Angew. Chem., Int. Ed., 2010, 49, 7340–7345 CrossRef CAS PubMed.
- M. Yamashina, M. Akita, T. Hasegawa, S. Hayashi and M. Yoshizawa, A polyaromatic nanocapsule as a sucrose receptor in water, Sci. Adv., 2017, 3, e1701126 CrossRef PubMed.
- G. Springsteen and B. H. Wang, A detailed examination of boronic acid-diol complexation, Tetrahedron, 2002, 58, 5291–5300 CrossRef CAS.
- X. Wu, Z. Li, X. X. Chen, J. S. Fossey, T. D. James and Y. B. Jiang, Selective sensing of saccharides using simple boronic acids and their aggregates, Chem. Soc. Rev., 2013, 42, 8032–8048 RSC.
- W. Zhai, X. Sun, T. D. James and J. S. Fossey, Boronic Acid-Based Carbohydrate Sensing, Chem. – Asian J., 2015, 10, 1836–1848 CrossRef CAS PubMed.
- T. D. James, K. R. A. S. Sandanayake and S. Shinkai, A Glucose-Selective Molecular Fluorescence Sensor, Angew. Chem., Int. Ed. Engl., 1994, 33, 2207–2209 CrossRef.
- J. N. Cambre and B. S. Sumerlin, Biomedical applications of boronic acid polymers, Polymer, 2011, 52, 4631–4643 CrossRef CAS.
- Y. Qu, J. Liu, K. Yang, Z. Liang, L. Zhang and Y. Zhang, Boronic Acid functionalized core-shell polymer nanoparticles prepared by distillation precipitation polymerization for glycopeptide enrichment, Chemistry, 2012, 18, 9056–9062 CrossRef CAS PubMed.
- S. Himmelein and B. J. Ravoo, A Self-Assembled Sensor for Carbohydrates on the Surface of Cyclodextrin Vesicles, Chemistry, 2017, 23, 6034–6041 CrossRef CAS PubMed.
- A. Stephenson-Brown, H. C. Wang, P. Iqbal, J. A. Preece, Y. Long, J. S. Fossey, T. D. James and P. M. Mendes, Glucose selective surface plasmon resonance-based bis-boronic acid sensor, Analyst, 2013, 138, 7140–7145 RSC.
- P. Ashokkumar, J. Bell, M. Buurman and K. Rurack, Analytical platform for sugar sensing in commercial beverages using a fluorescent BODIPY “light-up” probe, Sens. Actuators, B, 2018, 256, 609–615 CrossRef CAS.
- N. Y. Edwards, T. W. Sager, J. T. McDevitt and E. V. Anslyn, Boronic acid based peptidic receptors for pattern-based saccharide sensing in neutral aqueous media, an application in real-life samples, J. Am. Chem. Soc., 2007, 129, 13575–13583 CrossRef CAS PubMed.
- W. Yang, H. Fan, X. Gao, S. Gao, V. V. Karnati, W. Ni, W. B. Hooks, J. Carson, B. Weston and B. Wang, The first fluorescent diboronic acid sensor specific for hepatocellular carcinoma cells expressing sialyl Lewis X, Chem. Biol., 2004, 11, 439–448 CrossRef CAS PubMed.
- X. D. Xu, H. Cheng, W. H. Chen, S. X. Cheng, R. X. Zhuo and X. Z. Zhang, In situ recognition of cell-surface glycans and targeted imaging of cancer cells, Sci. Rep., 2013, 3, 2679 CrossRef PubMed.
- Y. R. Vandenburg, Z. Y. Zhang, D. J. Fishkind and B. D. Smith, Enhanced cell binding using liposomes containing an artificial carbohydrate-binding receptor, Chem. Commun., 2000, 149–150 RSC.
- X. Zhang, D. S. Alves, J. Lou, S. D. Hill, F. N. Barrera and M. D. Best, Boronic acid liposomes for cellular delivery and content release driven by carbohydrate binding, Chem. Commun., 2018, 54, 6169–6172 RSC.
- G. Wulff and S. Schauhoff, Enzyme-Analog-Built Polymers 27. Racemic-Resolution of Free Sugars with Macroporous Polymers Prepared by Molecular Imprinting – Selectivity Dependence on the Arrangement of Functional-Groups Versus Spatial Requirements, J. Org. Chem., 1991, 56, 395–400 CrossRef CAS.
- F. Bonini, S. Piletsky, A. P. Turner, A. Speghini and A. Bossi, Surface imprinted beads for the recognition of human serum albumin, Biosens. Bioelectron., 2007, 22, 2322–2328 CrossRef CAS PubMed.
- F. Shen and X. Ren, Covalent molecular imprinting made easy: a case study of mannose imprinted polymer, RSC Adv., 2014, 4, 13123–13125 RSC.
- T. Zhao, J. Wang, J. He, Q. Deng and S. Wang, One-step post-imprint modification achieve dual-function of glycoprotein fluorescent sensor by “Click Chemistry”, Biosens. Bioelectron., 2017, 91, 756–761 CrossRef CAS PubMed.
- M. Cieplak and W. Kutner, Artificial Biosensors: How Can Molecular Imprinting Mimic Biorecognition?, Trends Biotechnol., 2016, 34, 922–941 CrossRef CAS PubMed.
- L. Li, Y. Lu, Z. Bie, H. Y. Chen and Z. Liu, Photolithographic boronate affinity molecular imprinting: a general and facile approach for glycoprotein imprinting, Angew. Chem., Int. Ed., 2013, 52, 7451–7454 CrossRef CAS PubMed.
- Z. Bie, Y. Chen, J. Ye, S. Wang and Z. Liu, Boronate-Affinity Glycan-Oriented Surface Imprinting: A New Strategy to Mimic Lectins for the Recognition of an Intact Glycoprotein and Its Characteristic Fragments, Angew. Chem., Int. Ed., 2015, 54, 10211–10215 CrossRef CAS PubMed.
- R. Xing, Y. Ma, Y. Wang, Y. Wen and Z. Liu, Specific recognition of proteins and peptides via controllable oriented surface imprinting of boronate affinity-anchored epitopes, Chem. Sci., 2019, 10, 1831–1835 RSC.
- H.-C. Tu, Y.-P. Lee, X.-Y. Liu, C.-F. Chang and P.-C. Lin, Direct Screening of Glycan Patterns from Human Sera: A Selective Glycoprotein Microarray Strategy, ACS Appl. Bio Mater., 2019, 2, 1286–1297 CrossRef CAS.
- K. Torssell, Arylboronic acids. III. Bromination of tolylboronic acids according to Wohl-Ziegler, Ark. Kemi, 1957, 10, 507–511 CAS.
- Y. R. Freund, T. Akama, M. R. Alley, J. Antunes, C. Dong, K. Jarnagin, R. Kimura, J. A. Nieman, K. R. Maples, J. J. Plattner, F. Rock, R. Sharma, R. Singh, V. Sanders and Y. Zhou, Boron-based phosphodiesterase inhibitors show novel binding of boron to PDE4 bimetal center, FEBS Lett., 2012, 586, 3410–3414 CrossRef CAS PubMed.
- T. J. Robert, J. P. Jacob and D. Robert, Molecule of the Month, Curr. Top.
Med. Chem., 2011, 11, 1301–1303 CrossRef.
- V. Alterio, R. Cadoni, D. Esposito, D. Vullo, A. D. Fiore, S. M. Monti, A. Caporale, M. Ruvo, M. Sechi, P. Dumy, C. T. Supuran, G. Simone and J. Y. Winum, Benzoxaborole as a new chemotype for carbonic anhydrase inhibition, Chem. Commun., 2016, 52, 11983–11986 RSC.
- M. A. Alam, K. Arora, S. Gurrapu, S. K. Jonnalagadda, G. L. Nelson, P. Kiprof, S. C. Jonnalagadda and V. R. Mereddy, Synthesis and evaluation of functionalized benzoboroxoles as potential anti-tuberculosis agents, Tetrahedron, 2016, 72, 3795–3801 CrossRef CAS PubMed.
- M. Berube, M. Dowlut and D. G. Hall, Benzoboroxoles as efficient glycopyranoside-binding agents in physiological conditions: Structure and selectivity of complex formation, J. Org. Chem., 2008, 73, 6471–6479 CrossRef CAS PubMed.
- A. Pal, M. Berube and D. G. Hall, Design, synthesis, and screening of a library of peptidyl bis(boroxoles) as oligosaccharide receptors in water: identification of a receptor for the tumor marker TF-antigen disaccharide, Angew. Chem., Int. Ed., 2010, 49, 1492–1495 CrossRef CAS PubMed.
- C. Chen, G. El Khoury, P. Zhang, P. M. Rudd and C. R. Lowe, A carbohydrate-binding affinity ligand for the specific enrichment of glycoproteins, J. Chromatogr. A, 2016, 1444, 8–20 CrossRef CAS PubMed.
- J. P. Couturier, E. Wischerhoff, R. Bernin, C. Hettrich, J. Koetz, M. Sutterlin, B. Tiersch and A. Laschewsky, Thermoresponsive Polymers and Inverse Opal Hydrogels for the Detection of Diols, Langmuir, 2016, 32, 4333–4345 CrossRef CAS PubMed.
- M. Lin, Y. Zhang, G. Chen and M. Jiang, Supramolecular Glyco-nanoparticles Toward Immunological Applications, Small, 2015, 11, 6065–6070 CrossRef CAS PubMed.
- G. Chen, S. Huang, X. Kou, J. Zhang, F. Wang, F. Zhu and G. Ouyang, Novel Magnetic Microprobe with Benzoboroxole-Modified Flexible Multisite Arm for High-Efficiency cis-Diol Biomolecule Detection, Anal. Chem., 2018, 90, 3387–3394 CrossRef CAS PubMed.
- R. Deng, J. Yue, H. Qu, L. Liang, D. Sun, J. Zhang, C. Liang, W. Xu and S. Xu, Glucose-bridged silver nanoparticle assemblies for highly sensitive molecular recognition of sialic acid on cancer cells via surface-enhanced Raman scattering spectroscopy, Talanta, 2018, 179, 200–206 CrossRef CAS PubMed.
- C. Tuerk and L. Gold, Systematic evolution of ligands by exponential enrichment: RNA ligands to bacteriophage T4 DNA polymerase, Science, 1990, 249, 505–510 CrossRef CAS PubMed.
- A. D. Keefe, S. Pai and A. Ellington, Aptamers as therapeutics, Nat. Rev. Drug Discovery, 2010, 9, 537–550 CrossRef CAS PubMed.
- W. Sun, L. P. Du and M. Y. Li, Aptamer-Based Carbohydrate Recognition, Curr. Pharm. Des., 2010, 16, 2269–2278 CrossRef CAS PubMed.
- Q. Yang, I. J. Goldstein, H. Y. Mei and D. R. Engelke, DNA ligands that bind tightly and selectively to cellobiose, Proc. Natl. Acad. Sci. U. S. A., 1998, 95, 5462–5467 CrossRef CAS PubMed.
- M. Li, N. Lin, Z. Huang, L. Du, C. Altier, H. Fang and B. Wang, Selecting aptamers for a glycoprotein through the incorporation of the boronic acid moiety, J. Am. Chem. Soc., 2008, 130, 12636–12638 CrossRef CAS PubMed.
- A. E. Hargrove, A. D. Ellington, E. V. Anslyn and J. L. Sessler, Chemical Functionalization of Oligodeoxynucleotides with Multiple Boronic Acids for the Polyvalent Binding of Saccharides, Bioconjugate Chem., 2011, 22, 388–396 CrossRef CAS PubMed.
- X. Yang, C. Dai, A. Dayan Calderon Molina and B. Wang, Boronic
acid-modified DNA that changes fluorescent properties upon carbohydrate binding, Chem. Commun., 2010, 46, 1073–1075 RSC.
- J. Steinmeyer and H.-A. Wagenknecht, Synthesis of DNA Modified with Boronic Acid: Compatibility to Copper(I)-Catalyzed Azide–Alkyne Cycloaddition, Bioconjugate Chem., 2018, 29, 431–436 CrossRef CAS.
- M. M. Fuster and J. D. Esko, The sweet and sour of cancer: glycans as novel therapeutic targets, Nat. Rev. Cancer, 2005, 5, 526–542 CrossRef CAS PubMed.
- S. Cho, B.-R. Lee, B.-K. Cho, J.-H. Kim and B.-G. Kim, In vitro selection of sialic acid specific RNA aptamer and its application to the rapid sensing of sialic acid modified sugars, Biotechnol. Bioeng., 2013, 110, 905–913 CrossRef CAS PubMed.
- S. Gong, H.-L. Ren, R.-Y. Tian, C. Lin, P. Hu, Y.-S. Li, Z.-S. Liu, J. Song, F. Tang, Y. Zhou, Z.-H. Li, Y.-Y. Zhang and S.-Y. Lu, A novel analytical probe binding to a potential carcinogenic factor of N-glycolylneuraminic acid by SELEX, Biosens. Bioelectron., 2013, 49, 547–554 CrossRef CAS PubMed.
- S. Gong, H. Ren, C. Lin, P. Hu, R. Tian, Z. Liu, Y. Li, Y. Zhou, Y. Yang and S. Lu, Immunochromatographic strip biosensor for the rapid detection of N-glycolylneuraminic acid based on aptamer-conjugated nanoparticle, Anal. Biochem., 2018, 561–562, 52–58 CrossRef CAS PubMed.
- C.-Y. Wang, C.-Y. Wu, T.-C. Hung, C.-H. Wong and C.-H. Chen, Sequence-constructive SELEX: a new strategy for screening DNA aptamer binding to Globo H, Biochem. Biophys. Res. Commun., 2014, 452, 484–489 CrossRef CAS PubMed.
- Y. Jing, M. Cai, H. Xu, L. Zhou, Q. Yan, J. Gao and H. Wang, Aptamer-recognized carbohydrates on the cell membrane revealed by super-resolution microscopy, Nanoscale, 2018, 10, 7457–7464 RSC.
- M. Kizer, P. Li, B. F. Cress, L. Lin, T. T. Jing, X. Zhang, K. Xia, R. J. Linhardt and X. Wang, RNA Aptamers with Specificity for Heparosan and Chondroitin Glycosaminoglycans, ACS Omega, 2018, 3, 13667–13675 CrossRef CAS PubMed.
- H.-M. Kwon, K. H. Lee, B. W. Han, M. R. Han, D. H. Kim and D.-E. Kim, An RNA Aptamer That Specifically Binds to the Glycosylated Hemagglutinin of Avian Influenza Virus and Suppresses Viral Infection in Cells, PLoS One, 2014, 9, e97574 CrossRef PubMed.
- A. Díaz-Fernández, R. Miranda-Castro, N. de-los-Santos-Álvarez, E. F. Rodríguez and M. J. Lobo-Castañón, Focusing aptamer selection on the glycan structure of Prostate-Specific Antigen: toward more specific detection of prostate cancer, Biosens. Bioelectron., 2019, 128, 83–90 CrossRef PubMed.
- I. M. Ferreira, C. M. de Souza Lacerda, L. S. de Faria, C. R. Corrêa and A. S. R. de Andrade, Selection of Peptidoglycan-Specific Aptamers for Bacterial Cells Identification, Appl. Biochem. Biotechnol., 2014, 174, 2548–2556 CrossRef CAS PubMed.
- S.-I. Yamagishi, K. Taguchi and K. Fukami, DNA-aptamers raised against AGEs as a blocker of various aging-related disorders, Glycoconjugate J., 2016, 33, 683–690 CrossRef CAS.
- S. Saha, B. Kauffmann, Y. Ferrand and I. Huc, Selective Encapsulation of Disaccharide Xylobiose by an Aromatic Foldamer Helical Capsule, Angew. Chem., Int. Ed., 2018, 57, 13542–13546 CrossRef CAS PubMed.
- V. Spiwok, CH/pi Interactions in Carbohydrate Recognition, Molecules, 2017, 22, 1038 CrossRef PubMed.
- E. Jiménez-Moreno, G. Jiménez-Osés, A. M. Gómez, A. G. Santana, F. Corzana, A. Bastida, J. Jiménez-Barbero and J. L. Asensio, A thorough experimental study of CH/π interactions in water: quantitative structure–stability relationships for carbohydrate/aromatic complexes, Chem. Sci., 2015, 6, 6076–6085 RSC.
- B. B. Minsky, P. L. Dubin and I. A. Kaltashov, Electrostatic Forces as Dominant Interactions Between Proteins and Polyanions: an ESI MS Study of Fibroblast Growth Factor Binding to Heparin Oligomers, J. Am. Soc. Mass Spectrom, 2017, 28, 758–767 CrossRef CAS PubMed.
- I. Capila and R. J. Linhardt, Heparin-Protein Interactions, Angew. Chem., Int. Ed., 2002, 41, 390–412 CrossRef CAS.
- P. Mitchell, S. Tommasone, S. Angioletti-Uberti, J. Bowen and P. M. Mendes, ACS Appl. Bio Mater., 2019, 2, 2617–2623 CrossRef PubMed.
|
This journal is © The Royal Society of Chemistry 2019 |