Multi-electron reduction of Wells–Dawson polyoxometalate films onto metallic, semiconducting and dielectric substrates†
Received
16th November 2018
, Accepted 2nd December 2018
First published on 3rd December 2018
Abstract
The investigation of conditions allowing multi-electron reduction and reoxidation of polyoxometalate (POM) films onto solid substrates is considered an issue of critical importance for their successful incorporation in electronic devices, different types of sensors and catalytic systems. In the present paper, the rich multi-electron redox chemistry of films of Wells–Dawson ammonium salts, namely (NH4)6P2Mo18O62 and (NH4)6P2W18O62, on top of metallic (Al), semiconducting (ITO) and dielectric (SiO2) substrates under ambient conditions is investigated. The respective Keggin heteropolyacids, H3PMo12O40 and H3PW12O40, are also investigated for comparison. On Al substrates, the Wells–Dawson ammonium salts are found to be significantly more reduced (4–6e−) compared to the respective Keggin heteropolyacids (∼2e−), in accordance with their deeper lying lowest unoccupied molecular orbital (LUMO) level. Subsequent thermal treatment in air results in reoxidation of the initially highly reduced POM films. Similar behavior is found on ITO substrates, but in initially less reduced (2–4e−) Wells–Dawson POM films. On the other hand, on SiO2 substrates, the thermal reduction of (NH4)6P2Mo18O62 film is observed and attributed to the thermal oxidation of ammonium counterions by [P2Mo18O62]6− anions. Overall, the multi-electron reduction of Wells–Dawson ammonium salts onto metallic and semiconducting substrates (Al, ITO) is determined by the relative position of the LUMO level of POMs in relation to the Fermi level of the substrate (i.e. substrate work function) and affected in a synergistic way by the presence of ammonium counterions. In contrast, on dielectric substrates (SiO2) the reduction of Wells–Dawson POMs ((NH4)6P2Mo18O62) is attributed only to the oxidation of ammonium counterions.
1. Introduction
Polyoxometalates (POMs) are polynuclear metal-oxygen anionic clusters with well-defined molecular structure and interesting redox properties that are investigated for applications in many scientific fields such as catalysis, materials science, and medicine.1–4 Especially in photocatalysis, POMs are widely used as photocatalysts mainly owing to their capability to undergo reversible multi-electron photoredox reactions without decomposition. The multi-electron transfer capability of POMs stems primarily from the high number of metal centers present in their structure. On the other hand, the reversibility results mostly from the capacity of POMs to retain their structure during photochemical (or electrochemical) electron transfer reactions and to reoxidize efficiently.5–7 Thus, initially, POMs had been used mainly as homogeneous photocatalysts for the photooxidation or mineralization of a wide range of organic compounds (such as alcohols, alkenes, alkanes, and chlorinated hydrocarbons).8 More recently, POMs have been investigated primarily as potential photocatalysts in solar light active systems for energy conversion and storage, especially in the photooxidation of water and the photoreductive evolution of hydrogen, owing to their multi-electron photoredox capability.9–15
Multi-electron redox reactions (primarily reductions) of POMs are favored in a number of cases in POM-mediated catalysis. Especially in electrocatalysis, POMs are extensively used as electrocatalysts (mediators) in homogeneous electrochemical reactions, where they catalyze the electron transfer between the electrode and the electrolyte solution.16–18 Thus, a vast range of POMs has been investigated as electrocatalysts, with the majority of them being the Keggin- and Wells–Dawson-structure heteropolyanions ([XM12O40]p− and [X2M18O62]q− respectively; mainly M = W, Mo, and X = P, Si), mixed-addenda heteropolyanions, and transition metal-substituted heteropolyanions.16,19–21 Therefore, it is apparent that the multi-electron reduction of POMs and their capacity to act as electron reservoirs without significant structural changes (the specific number of electrons accepted depends on the POM structure and pH of solution) is an issue of highest importance, especially for the closely related scientific fields of photocatalysis, electrocatalysis and electronics.22,23
In a similar manner with electrocatalysis, recently POMs (and their analogue metal oxides) have been proposed and used as interfacial charge transporting layers (i.e. mediators) in applications such as organic optoelectronics, photocatalytic devices and fuel cells, whereas special interest has been also devoted in their use as key components in molecular electronics.24,25 Especially in organic optoelectronics (i.e. organic light emitting diodes, OLEDs, and organic photovoltaics, OPVs) POMs are mostly used as interfacial layers to promote charge (electrons/holes) injection or extraction respectively, between the active polymeric layer (i.e. organic semiconducting layer) and the metal-based electrodes (cathode/anode). In most of the cases, interfacial redox reactions take place between POM layers and metal-based electrodes or support substrates of interest in photocatalysis that are either reported in an insufficient manner or even completely ignored. In recent publications of our group, POMs have been shown to act as efficient electron injection/extraction layers between Al-cathode and the active polymeric layer in OLEDs/OPVs applications respectively.26–28 Multi-electron reduction of POMs was observed onto Al-cathode forming occupying LUMO levels of POMs that align with the high work function (EF = −4.3 eV) of Al, creating thus an efficient electron conducting path and favoring the overall energy level alignment between Al and the active polymeric layer at the POM-modified cathode interface. In addition, POMs have been reported (by our and other groups) as efficient hole injection/extraction layers positioned between ITO (or Au) anode and the active polymeric layer in optoelectronic devices.29–33 In this case, effective energy level alignment between POM films and ITO (or Au) anode in addition to interfacial oxidation (or p-doping) of the polymer donor by POMs was reported. Possible POM reduction by the anode electrode was not investigated in that case.
Motivated by the above results, in the current paper we investigate in depth the redox behavior of ammonium salts of Wells–Dawson POMs, (NH4)6P2Mo18O62 and (NH4)6P2W18O62, which are among the best performing POMs in both optoelectronic devices and hydrogen production, on top of different solid substrates of interest to organic optoelectronics, molecular electronics, and photocatalysis. Our goal is to investigate the interfacial electron exchange between films of ammonium salts of Wells–Dawson POMs and metallic, semiconducting and dielectric substrates, under ambient conditions. Therefore, we selected Al and ITO substrates, because they are used as typical electrode materials in organic optoelectronic and molecular electronic devices. In addition, we selected SiO2 as a typical dielectric substrate of interest in both electronics and catalytic applications. For comparison with the Wells–Dawson ammonium salts, the redox chemistry of the respective Keggin heteropolyacids (H3PMo12O40, H3PW12O40) was investigated. The present investigation on the redox chemistry of Wells–Dawson ammonium salts onto various solid substrates aims to pave the way for new applications of POM-based charge transport layers not only in the broad field of electronic devices, but also in the area of energy harvesting and storage devices such as fuel cells, solar cells, batteries, and heterogeneous catalysis.2–4,9
2. Experimental section
2.1. Materials
The ammonium 18-molybdodiphosphate hydrate, (NH4)6P2Mo18O62·xH2O, was synthesized according to the modified procedure reported by Tsigdinos,34,35 and characterized with FT-IR (1% KBr pellet; Nicolet-Avatar 370) and 31P NMR spectroscopy (400 MHz JEOL, resonance frequency for 31P was 161.6 MHz) (Fig. S1a and S2a, ESI†).36–44 The ammonium 18-tungstodiphosphate hydrate, (NH4)6P2W18O62·xH2O, was synthesized according to well-established methods,34 and characterized with FT-IR (1% KBr pellet; Nicolet-Avatar 370) and 31P NMR spectroscopy (JEOL 400 MHz) (Fig. S1b and S2b, ESI†).38,40,42,44–48 The heteropolyacids hydrates, H3PW12O40·xH2O, H3PMo12O40·xH2O, and H4SiW12O40·xH2O (Aldrich) were of analytical grade and used without further purification. All solvents (purchased from usual commercial sources) were of analytical grade and used as received. Deionized water (with a resistivity of 15 MΩ cm−1) prepared from a Millipore, Milli-RO plus 90 apparatus was used where necessary.
2.2. Preparation of POM films onto solid substrates
POM films were coated on top of three different solid substrates/underlayers: (1) Al-films with a thickness range of 5–10 nm (in order to be semitransparent) evaporated onto Si-wafers and fused quartz slides in a dedicated vacuum chamber. (2) Indium tin oxide (ITO)-coated glass slides (Aldrich) with ITO thickness ∼100 nm. (3) Bare Si-wafers (with a native ∼9 nm thick SiO2 film) and fused quartz slides cleaned with piranha solution (H2O2
:
H2SO4, 1
:
1 v/v). For the formation of POM films onto those solid substrates, POM solutions in a concentration range of 5–10 mg ml−1 in MeOH were used. POM films were coated with two different techniques: (1) spin-coating at a spinning velocity range of 600–700 rpm, for 30–40 s, forming ∼2 nm-thick POM films (called henceforward as thin POM films). (2) Drop casting (solvent evaporation) at room temperature (RT) forming ∼10 nm-thick POM films (called henceforth as thick POM films). Drop casting was used, despite its disadvantage of forming less homogeneous films, because it is a simple method for the high increase of POM film thickness without the need of sharp increase in the POM concentration in solution, and also because a significant number of initial measurements (i.e. UV-Vis, FT-IR, XPS, UPS) were made with this coating method. After coating of POM films, a thermal treatment step (in ambient air) from RT to 190 °C, for 5 min, was performed in some cases.
2.3. Physicochemical characterization of POM films
UV-Vis absorption spectra of POM films onto Al-coated quartz slides, ITO-coated glass slides, and bare quartz slides, were recorded on a Perkin-Elmer Lambda 40 spectrophotometer. (A typical UV-Vis spectrum of an Al-coated quartz slide is given in Fig. S5c, ESI†.) In the UV-Vis spectra of POM films, the spectrum of the solid substrate was not subtracted, because POMs react with the substrate (to a different degree depending on the nature of the substrate/underlayer) through interfacial redox reactions. (Those reactions may have impact on the nature and thickness of the underlayer, e.g. by forming an ultra-thin metal oxide layer at the interface.) The same concept was also applied for the FT-IR measurements of POM films onto Al-coated and bare Si-wafers. FT-IR transmission spectra of POM films onto those substrates were obtained on a Bruker Tensor 27 spectrometer (at 4 cm−1 resolution, 128 scans) with a DTGS detector. In the case of thermally treated POM films onto the above substrates, the UV-Vis and FT-IR measurements were performed immediately after (not in situ) the thermal treatment step. The thickness of POM films on all substrates (Al, ITO, SiO2) investigated in the present work was measured with an Ambios XP-2 profilometer, whereas the thickness of POM films on the non-transparent SiO2 substrates was also measured with a M2000 Woolam ellipsometer. The thicknesses of POM films are given in approximate values (i.e. ∼2 nm for thin POM films and ∼10 nm for thick POM films) in the manuscript, because these values are at the limits of profilometry. Moreover, as proven later in the manuscript (pls. see Section 3.1.1 and also Fig. S5 (ESI†) and discussion therein) the POM films' thickness affect the interfacial redox processes of those films on the above substrates in an apparent way and therefore, only relative changes in POM film thicknesses are necessary to reach this conclusion.
For the chemical analysis by X-ray and Ultraviolet Photoelectron Spectroscopy (XPS, UPS) POM films (7–10 nm thick) were formed (through spin-coating or solvent-evaporation) onto the following substrates: Al (∼10 nm) coated Si-wafers, ITO (100 nm) coated glass slides and SiO2 (9 nm) coated Si-wafers. For the XPS measurements the Mg Kα (1253.6 eV) line and a hemispherical EA-11 Leybold analyzer with a pass energy of 100 eV were used. The C 1s peak of the surface adventitious carbon at 284.8 eV was taken as a reference for the binding energy scale. The stoichiometry of POMs was estimated from the XPS W 4f and Mo 3d core levels respectively, and the respective O 1s photoemission peaks. The fitting of the O 1s and W 4f (or Mo 3d) spectra area was performed, after a Shirley background subtraction with a non-linear least-squares routine using peaks with a mix of Gaussian and Lorentzian shapes. The error was estimated at ±10% in all the XPS-derived atomic percentages. For the determination of valence band and work function of POMs, UPS measurements were performed using the HeI (21.22 eV) excitation line. A negative bias of 12.28 V was applied to the samples during UPS measurements in order to separate the sample and analyzer high binding energy (BE) cut-offs and estimate the absolute work function value from the secondary BE cut-off region of the UPS spectra. The analyzer resolution was determined from the width of the Au Fermi edge to be 0.16 eV.
3. Results and discussion
Due to the highly promising results (i.e. high performance in OLED and OPV devices) obtained in a recent publication of our group by using reduced POM layers onto Al cathodes,28 we decided to investigate in more depth the reduction of POM films onto Al substrates and extend it also to other substrates for a possibly wider field of applications. Thus, in the present work, the interfacial redox reactions of POM films (both Wells–Dawson- and Keggin-POM films) on top of metallic (Al), semiconducting (ITO) and dielectric (SiO2) substrates is investigated as a guide for a variety of applications such as organic optoelectronics, sensors, molecular electronics, catalysis, and photocatalysis. In most of the cases, in organic optoelectronics, and possibly in photocatalytic applications, interfacial redox reactions take place also between POM films and subsequent device layers deposited on top of them, but in the present paper we focus only on the interactions of POM films with commonly used substrates. For the study of these interfacial redox reactions of POM films onto the above substrates the following factors are investigated: (1) effect of substrate's nature (metallic, semiconducting or dielectric) with emphasis on the substrate work function value (EF) especially for metallic (Al, −4.3 eV) and semiconducting (ITO, −4.7 eV) substrates; (2) effect of POM structure with emphasis on the LUMO position of POMs, and POM film thickness; and (3) effect of thermal treatment temperature (in ambient air) to reveal the important role of ammonium counterions.
3.1. Redox chemistry of POM films onto Al substrates
3.1.1. Effect of POM structure and POM film thickness.
Initially, the effect of POM structure, i.e. Wells–Dawson structure ([P2Mo18O62]6−, [P2W18O62]6−) versus Keggin structure ([PMo12O40]3−, [PW12O40]3−) on the spontaneous reduction of POM films onto Al substrates upon contact was investigated. This reduction has been already reported in a previous publication of our group as having a critical importance in the use of POMs as cathode interfacial layers of organic optoelectronic devices.28 Here we extend this work investigating the influence of parameters that may affect the extent of the reduction. The reduction of POM films was traced with UV-Vis absorption spectroscopy, where the degree of reduction was deduced mainly from the position of the intervalence charge transfer (IVCT) band of POMs (Fig. 1). As shown in this figure, both Keggin heteropolyanions ([PMo12O40]3−, [PW12O40]3−) are approximately reduced to the same degree (∼2e−) based on the position of the IVCT band.23,36,49 In contrast, the Wells–Dawson POMs ([P2Mo18O62]6−, [P2W18O62]6−) are more highly reduced (4–6e−) onto Al substrates, due to their lower lying LUMO levels with respect to the Al Fermi level.36,50 These UV-Vis results are in agreement with the results previously reported,28 whereas small differentiations (e.g. in the reduction degree of [P2Mo18O62]6− anions) are obtained with variation of POM film thickness. The higher reduction degree of Wells–Dawson POMs (on Al substrates) in relation to the respective Keggin POMs was confirmed by XPS spectroscopy (Fig. S3, ESI†) in accordance also with our previous results.28 In particular, Fig. S3a and c (ESI†) depicts the W4f core level XPS peaks along with their fittings of [PW12O40]3− and [P2W18O62]6− films, while Fig. S3b and d (ESI†) depicts the Mo 3d XPS peaks and fittings of [PMo12O40]3− and [P2Mo18O62]6− films on Al substrates. It is obvious that the contribution of doublets attributed to lower oxidation states (i.e. W5+ and Mo5+) is significantly higher in the spectra of Wells–Dawson POMs as compared with the corresponding Keggin POMs. This clearly suggests a higher degree of reduction of Wells–Dawson POMs. Notably, the Mo-based POMs (either Keggin or Wells–Dawson type) appear more reduced as compared to the W-based POMs of the same type, a result which can be explained by taking into account the lower LUMO position of the Mo-based POMs as discussed below. Furthermore, the effect of POM film thickness on the reduction degree of Wells–Dawson POMs ([P2Mo18O62]6−, [P2W18O62]6−) onto Al substrates was also investigated with UV-Vis spectroscopy (Fig. S5, ESI†). It was proven that the lower the POM film thickness the higher the reduction degree of Wells–Dawson POMs, a finding which was rather apparent due to the self-limiting nature of this interfacial process.
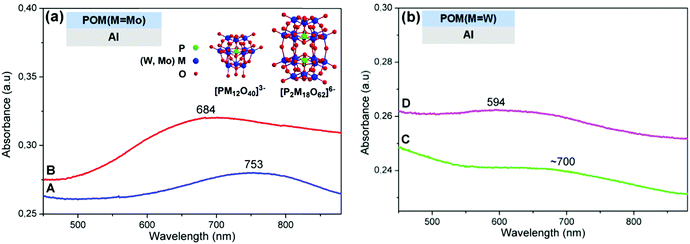 |
| Fig. 1 UV-Vis spectra of thin POM films: (a) POMs based on M = Mo addenda atoms, (A) H3PMo12O40, (B) (NH4)6P2Mo18O62, and (b) POMs based on M = W addenda atoms, (C) H3PW12O40, (D) (NH4)6P2W18O62, onto Al-coated quartz slides. In all cases the change of the IVCT band of POMs is shown. Inset in (a) Keggin structure, [PM12O40]3−, and Wells–Dawson structure, [P2M18O62]6−, M = Mo, W. (Concentration of Keggin and Wells–Dawson POMs, 10 and 5 mg ml−1 in MeOH respectively; spin-coating, 600 rpm, 40 s; no thermal treatment applied; 5 nm-thick Al film.) | |
3.1.2. Effect of thermal treatment temperature – the role of ammonium counterions.
Having confirmed that Wells–Dawson POM films are more highly reduced onto Al substrates than the respective Keggin POM films, the effect of thermal treatment temperature on the reduction of thin (∼2 nm) Wells–Dawson POM films ((NH4)6P2Mo18O62, (NH4)6P2W18O62) onto Al substrates was investigated with UV-Vis absorption spectroscopy (Fig. 2). Initially, the thin (NH4)6P2Mo18O62 film presented an IVCT band at 692 nm attributed to an electron transfer MoV → MoVI (with the MoVI atom belonging to a different Mo3O13 group).1,51 This result indicates that the film is reduced with ∼4e− on Al substrate, in agreement with literature (Fig. 2a right).36,50 Upon increase of thermal treatment temperature, the decrease in intensity and red-shift of the IVCT band until its complete elimination was observed indicating that the (NH4)6P2Mo18O62 film is gradually reoxidized up to ∼190 °C in ambient air.50 Similarly, upon increase of thermal treatment temperature of a thin (NH4)6P2Mo18O62 film onto Al substrate, the oxygen-to-metal charge transfer (OMCT) band at ∼328 nm attributed to Ob,c → Mo transitions increases in intensity (whereas the OMCT band at ∼237 nm ascribed to Od → Mo slightly decreases in intensity and blue-shifts; Fig. 2a left).7,19 This result indicates also that thermal reoxidation of a thin (NH4)6P2Mo18O62 film takes place onto Al substrate, with reformation of a significant number of Mo–Ob,c–Mo bridges. Furthermore, a distinct change observed to both OMCT and IVCT bands at 130 °C during thermal reoxidation of (NH4)6P2Mo18O62 film could be attributed to a change of class II → III according to Robin–Day's classification (i.e. from the model of mixed-valence species to the model of complete delocalization of added electrons via annealing).19,50,52 The thermal reoxidation of (NH4)6P2Mo18O62 film onto Al substrate appears to be a similar (but reverse) process to the electrochemical and photochemical reduction of [P2Mo18O62]6− anions within alcoholic aqueous solutions and hydroxyl group-containing polymeric films.7,50,53 Analogous observations were made for the thermal reoxidation of thin (NH4)6P2W18O62 film onto Al substrate (Fig. 2b), as well as the thick (∼10 nm) (NH4)6P2Mo18O62 and (NH4)6P2W18O62 films, and the thin Keggin H4SiW12O40 film (onto Al substrates; Fig. S6, ESI†).19,45,50
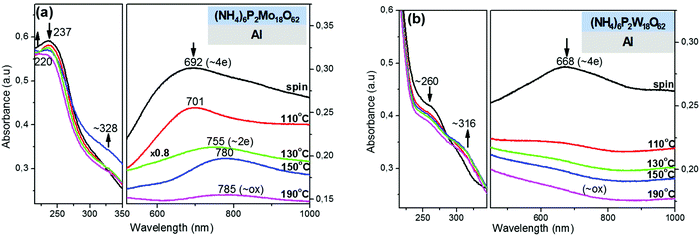 |
| Fig. 2 UV-Vis monitoring of thin POM films: (a) (NH4)6P2Mo18O62 and (b) (NH4)6P2W18O62, onto Al-coated quartz slides, upon increase of thermal treatment temperature. In all cases the change of both IVCT and OMCT bands of POMs is shown. (POM concentration, 10 mg ml−1 in MeOH; spin-coating, 700 rpm, 30 s; thermal treatment in air, at a temperature range of RT–190 °C, for 5 min; 5–10 nm thick Al film.) | |
Next, the effect of thermal treatment temperature on the chemical bonds of Wells–Dawson POMs (in form of films) on Al substrates was investigated using FT-IR transmission spectroscopy (Fig. 3). In particular, upon increase of thermal treatment temperature of (NH4)6P2Mo18O62 film, the following blue-shifts of peaks were observed: (1) 1069 → 1076 cm−1 attributed to antisymmetric stretching (vas) of P–Oa bonds;36–38 (2) 995 → 1002 and 932 → 938 cm−1 ascribed to vs, vas of Mo
Od bonds respectively;39 and (3) ∼900 → 904 cm−1 attributed to vas of Mo–Ob–Mo bridges (where Oa are the oxygen atoms bound to central heteroatom P; Ob the bridging oxygen atoms that belong to corner-sharing octahedra (i.e. in different M3O13 triads); Oc the bridging oxygen atoms that belong to edge-sharing octahedra (i.e. inside a M3O13 triad); and Od the terminal oxygen atoms).37,38 Those shifts indicate clearly strengthening of the respective chemical bonds (P–Oa, Mo
Od, and Mo–Ob–Mo) of [P2Mo18O62]6− anions upon thermal treatment on Al substrate, a result which fully supports the thermal reoxidation of those anions and was more or less expected.36–38
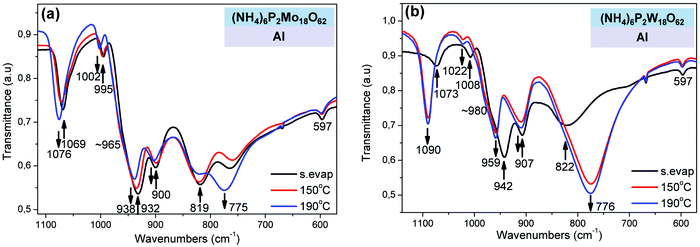 |
| Fig. 3 FT-IR monitoring of thick POM films: (a) (NH4)6P2Mo18O62 and (b) (NH4)6P2W18O62, onto Al-coated Si wafers, upon increase of thermal treatment temperature. (POM concentration, 5 mg ml−1 in MeOH; drop casting at RT; thermal treatment in air, at a temperature range of RT–190 °C, for 5 min; 10 nm-thick Al film.) | |
On the other hand, the band at 819 cm−1 attributed to vas of Mo–Oc–Mo bridges, decreases in intensity and undergoes a large red-shift (819 → 775 cm−1) indicating that those bridges are significantly weakened and reform during the thermal reoxidation of (NH4)6P2Mo18O62 film. That result is astonishing and in complete contrast with the above FT-IR results. In this case, the large weakening of Mo–Oc–Mo bridges during the thermal reoxidation of (NH4)6P2Mo18O62 film could be explained only on the basis of decrease of protonation of Oc oxygens. In particular, during the initial contact of (NH4)6P2Mo18O62 film on Al substrate, POM clusters are spontaneously reduced possibly through protonation of Oc oxygens leading to the formation of MoVI–Oc–H bonds and weakening of the adjacent reduced MoV–Oc bonds (Scheme 1; straight reaction to the right). The protonation of Oc oxygens is expected to induce high strengthening of Mo–Oc bonds (considerably higher than the weakening of the adjacent reduced MoV–Oc bonds). Upon thermal reoxidation of (NH4)6P2Mo18O62 film onto Al substrate, the degree of protonation of Oc oxygens decreases and therefore the reformation and weakening of Mo–Oc–Mo bridges is observed in the FT-IR spectrum (Scheme 1; reverse reaction to the left). In this case, the protons (which induce the protonation of Oc oxygens) originate possibly from the oxidation (via hydrogen abstraction) of ammonium counterions or maybe by water molecules preassociated with [P2Mo18O62]6− anions. The selective protonation of Oc oxygens in [P2Mo18O62]6− anions occurs possibly, because Oc oxygens are mainly bonded to equatorial (belt) metals, which have been reported as the major contributors to the LUMO and LUMO+1 levels, and therefore favorable reduction sites in Wells–Dawson POMs.54,55 Furthermore, the Mo–Oc–Mo bridges have been reported to be dissociated during the photochemical reduction of [P2Mo18O62]6− anions within polymeric films,53 and in (electro)chemical reduction of the equivalent Keggin [PMo12O40]3− anions in solution and vapor phase.39,56,57 Similar FT-IR results were also obtained for the thermal reoxidation of (NH4)6P2W18O62 film onto Al substrate (Fig. 3b).40,45
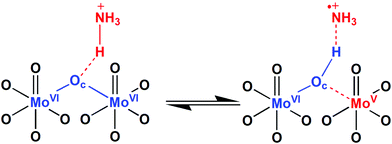 |
| Scheme 1 A possible reduction mechanism of (NH4)6P2Mo18O62 film (via oxidation of ammonium counterions by [P2Mo18O62]6− anions) that takes place spontaneously on Al substrate and thermally on SiO2 substrate (straight reaction to the right). The reoxidation of the reduced (NH4)6P2Mo18O62 film takes place thermally on Al substrate (reverse reaction to the left). | |
3.2. Redox chemistry of POM films onto ITO substrates
Next, the spontaneous reduction of thin POM films on ITO substrates were complimentarily investigated with XPS spectroscopy (Fig. 4). The reduction of Mo-containing POMs (H3PMo12O40, (NH4)6P2Mo18O62) was monitored using their XPS Mo 3d photoemission peaks. The data collected were fitted with two pairs of Mo 3d doublet components. The first component has Mo 3d5/2 and Mo 3d3/2 with BEs of 232.7 eV and 235.8 eV respectively, which is attributed to fully oxidized (Mo6+) Mo atoms.58,59 The second component has Mo 3d5/2 and Mo 3d3/2 with BEs of 231.6 eV and 234.9 eV respectively, characteristic of the reduced (Mo5+) molybdenum.59 The contribution of the second doublet (reduced +5 states) in the overall photoemission peaks is significantly higher in the Wells–Dawson (NH4)6P2Mo18O62 film than in the Keggin H3PMo12O40 film. Similar behavior was also observed in W-containing POMs ((NH4)6P2W18O62, H3PW12O40; spectra not shown),60 but in that case the reduction degree of POMs was generally lower than the reduction degree of the respective Mo-containing POMs. Thus, according to the XPS results the increase of the reduction degree of POMs on ITO substrates follows the order: H3PW12O40 < (NH4)6P2W18O62 < H3PMo12O40 < (NH4)6P2Mo18O62, which is in agreement with the order of decreasing of POMs' LUMO energy (Fig. 7; the MO values of POMs in this diagram were deduced from the UPS spectra of POM films on Al substrates (Fig. S4, ESI†) and the UV-Vis spectra of POM films on SiO2 substrates (Fig. S7 and Table S1, ESI†)). In other words, it is evidenced that the lower the LUMO level of POMs (with respect to the Fermi level of ITO), the higher the reduction degree of POMs on ITO substrates. A small exception to this rule seems to be the (NH4)6P2W18O62 film, which appears to be less reduced on ITO substrate (according to XPS) than the H3PMo12O40 film despite the slightly lower LUMO position of the former (−5.3 vs. −5.1 eV respectively), a fact that could be attributed to the relatively low homogeneity and film thickness of (NH4)6P2W18O62 film.
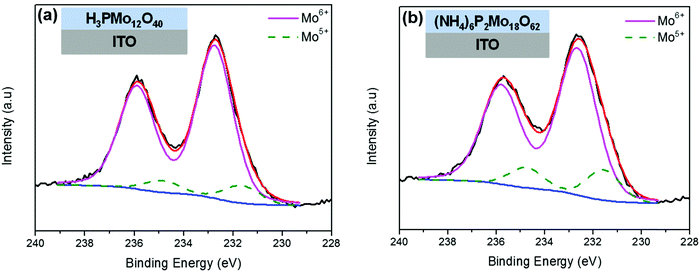 |
| Fig. 4 XPS measurements of thin POM films: (a) H3PMo12O40, and (b) (NH4)6P2Mo18O62, onto ITO substrates. (Concentration of Keggin and Wells–Dawson POMs, 10 and 5 mg ml−1 in MeOH respectively; spin-coating, 700 rpm, 40 s; no thermal treatment applied; 100 nm-thick ITO film.) | |
Similar observations regarding the spontaneous reduction of POM films onto ITO substrates were also made with UV-Vis absorption spectroscopy. In particular, on ITO substrates the thin POM films are spontaneously reduced, whereas the thick POM films are practically not reduced. From the thin POM films, the Wells–Dawson POM films are reduced to a significant degree (e.g. the thin (NH4)6P2Mo18O62 film is reduced with ∼4e− based on the IVCT band position; Fig. 5a),50 whereas the thin Keggin POM films are reduced to a small degree (spectra not shown). Furthermore, the effect of thermal treatment temperature on the reduction of a thick (NH4)6P2Mo18O62 film onto ITO substrate, was investigated with UV-Vis spectroscopy (Fig. 5b). Initially the thick (NH4)6P2Mo18O62 film on ITO substrate was in oxidized state. Upon thermal treatment the (NH4)6P2Mo18O62 film is thermally reduced (with 2–4e−) up to 110 °C, whereas at a higher temperature (≥130 °C) the film reoxidizes thermally exhibiting a redox behavior similar to the one observed on Al substrate. This result indicates that Wells–Dawson POM films coated directly on ITO substrates and thermally treated at ∼130 °C are reduced with ∼2e−, a fact that is often overlooked in organic optoelectronic applications. Possibly, this fact explains the capability of Wells–Dawson POM films to act also as hole transport layers on ITO substrates (i.e. through occupying LUMO levels as it occurs on Al substrates).
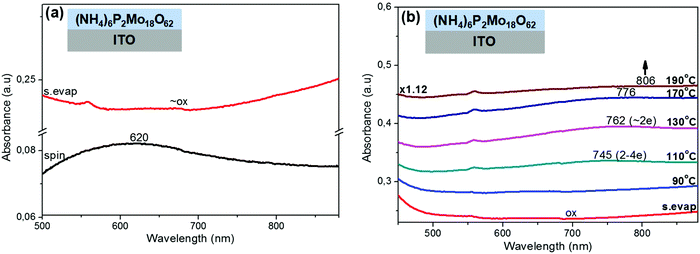 |
| Fig. 5 (a) UV-Vis spectra of one thin and one thick (NH4)6P2Mo18O62 films, and (b) UV-Vis monitoring of thermal treatment of a thick (NH4)6P2Mo18O62 film, onto ITO-coated glass substrate. (POM concentration, 5 mg ml−1 in MeOH; spin-coating (for thin film), 700 rpm, 30 s; drop casting (for thick film) at RT. In (b), thermal treatment in air, at a temperature range of RT–190 °C, for 5 min; ∼100 nm-thick ITO film.) | |
3.3. Redox chemistry of POM films onto SiO2 substrates
The reduction of POM films onto SiO2 substrates is an issue of high importance, because SiO2 is the most commonly used dielectric substrate in electronic and photocatalytic applications, and POMs are generally considered as not being reduced onto those substrates. In our investigation, initially all POM films (Keggin or Wells–Dawson, thin spin-coated or thick solvent-evaporated POM films) were in oxidized state, as observed with both UV-Vis and XPS spectroscopy (Fig. 6a, Fig. S8 and S9, ESI†). Upon thermal treatment (from RT to 190 °C in air), only the thin (NH4)6P2Mo18O62 film was reduced on SiO2 substrate (Fig. 6a right), whereas all the other thin POM films were not thermally reduced at all (Fig. S9a and b, ESI†). In particular, the thin (NH4)6P2Mo18O62 film is thermally reduced onto SiO2 showing a typical reduction behavior (i.e. appearance of the IVCT band at the visible region and blue-shift of that band, 767 → 741 nm; Fig. 6a right) similarly to the initially highly reduced state of (NH4)6P2Mo18O62 film on Al substrate (Fig. 2a). The thin (NH4)6P2Mo18O62 film is thermally reduced with 2–4e− at 190 °C onto SiO2, based on the IVCT band position (741 nm).49 The thermal reduction of (thin) (NH4)6P2Mo18O62 film onto SiO2 was also confirmed by the slight decrease in intensity and red-shift of the OMCT band (217 → 221 nm; Fig. 6a left).50 Similar observations were also made by UV-Vis monitoring of thermal treatment of a thick (NH4)6P2Mo18O62 film onto SiO2 leading to a lower reduction degree (<2e−; Fig. S9c, ESI†).
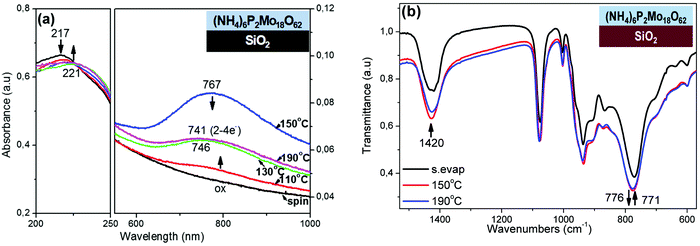 |
| Fig. 6 (a) UV-Vis monitoring of a thin (NH4)6P2Mo18O62 film onto bare quartz slide, and (b) FT-IR monitoring of a thick (NH4)6P2Mo18O62 film onto bare Si wafer (with a native ∼3 nm-thick SiO2), upon increase of thermal treatment temperature. In (a) the change of both IVCT and OMCT bands of POM is shown. ((a) POM concentration, 10 mg ml−1 in MeOH; spin-coating, 700 rpm, 30 s; thermal treatment in air, at a temperature range of RT–190 °C, for 5 min. (b) POM concentration, 5 mg ml−1 in MeOH; drop casting at RT; thermal treatment in air, at a temperature range of RT–190 °C, for 5 min.) | |
Furthermore, the thermal reduction of (NH4)6P2Mo18O62 film onto SiO2 was also confirmed with FT-IR spectroscopy (Fig. 6b). In particular, the slight shift of the vas (Mo–Oc–Mo) band to higher wavenumbers (771 → 776 cm−1) indicates strengthening of that band, which was previously attributed to protonation of Oc oxygens (similarly to the initially highly-reduced state of (NH4)6P2Mo18O62 film on Al substrate; Fig. 3a).39,53,56,57 Moreover, the slight decrease in intensity of NH4+ band (at 1420 cm−1) indicates possibly decrease of NH4+ concentration during thermal reduction of (NH4)6P2Mo18O62 film onto SiO2.40,41,44
From the above spectroscopic results, it is apparent that the (NH4)6P2Mo18O62 film is thermally reduced onto SiO2 substrate. The oxidation of the remaining solvent (methanol) by POM anions is rather unlikely, especially for spin-coated POM films. On the other hand, the possibility of oxidation of water molecules (derived from the atmosphere and preassociated with POM anions) via H-abstraction and formation of hydroxyl radicals (OH˙) is rather limited, though not completely eliminated.5–9 Consequently, the thermal reduction of (NH4)6P2Mo18O62 film onto SiO2 substrate is possibly induced by thermal oxidation of NH4+ counterions, via H-abstraction, by the ground state of [P2Mo18O62]6− anions.6,7,9,61 During this thermally-induced reduction process, one electron is transferred from the highest occupied molecular orbital (HOMO) of NH4+ cations to the LUMO of the ground state of POM anions. According to the proposed mechanism (Scheme 1; straight reaction to the right), upon thermal treatment an H-atom is abstracted by an Oc oxygen from a preassociated NH4+ cation leading to the formation of a MoVI–Oc–H bond and ammonia cation-radical (NH3+˙). Concurrently, the MoVI–Oc bond (of the same oxygen) belonging to an adjacent octahedral MoO6 site is homolytically cleaved and the MoVI atom is reduced by one electron (to MoV). The reaction then proceeds through the formation of a charge-transfer complex involving two MoO6 octahedra and one NH4+ counterion (in contrast to the complex reported for the photochemical reduction of polyoxomolybdates in solid-state, where one MoO6 octahedron and one NH4+ counterion were involved).5,62 Furthermore, the reduction of [P2Mo18O62]6− anions induced by thermally-oxidized NH4+ counterions onto SiO2 seems to proceed not only to 1e− (i.e. to formation of [HP2Mo18O62]6−) shown in the mechanism, but up to 2–4e− (i.e. to formation of [H2P2Mo18O62]6− or [H4P2Mo18O62]6−) according to the UV-Vis results. In this case, the further oxidation of the 1e− oxidized cation-radicals (NH3+˙) cannot be confirmed, neither can the formation of stable NH3 molecules (since the 1e− reduction, instead of oxidation, of NH3+˙ cation-radicals would be necessary). Thus, it seems that highly oxidized, nitrogen-based products possibly form, but the whole issue will be investigated in a forthcoming publication.
3.4. Discussion on the interfacial electron transfer mechanisms of POM films on top of Al, ITO and SiO2 substrates
Recapitulating and based on the molecular orbitals (MO) diagram of POM anions (the MO values of POMs were deduced from UPS and UV-Vis spectra of POM films; Section 3.2), counter-cations, water, and substrates (Fig. 7) the following findings are extracted regarding the interfacial electron transfer mechanisms of Wells–Dawson ammonium salts onto metallic, semiconducting and dielectric substrates.
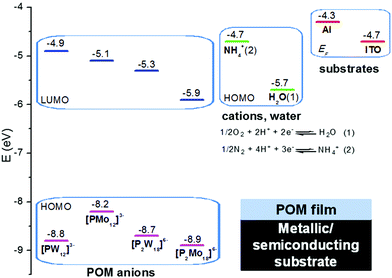 |
| Fig. 7 Molecular orbitals (MO) diagram of Keggin and Wells–Dawson POM anions, ammonium counter-cations, water and substrates used in the present investigation. (The MO values of POMs in this diagram were deduced from UPS and UV-Vis spectra of POM films; Section 3.2.) | |
On Al substrates, the spontaneous reduction of (NH4)6P2Mo18O62 films proceeds mainly through electron transfer from the Fermi level of Al (−4.3 eV) to the LUMO of [P2Mo18O62]6− anions (−5.9 eV) according to energetic considerations. A synergistic (rather than competitive) mechanism is the electron transfer from the HOMO of NH4+ cations (−4.71 eV) to the LUMO of [P2Mo18O62]6− anions, a mechanism which is evidenced by FT-IR results and favored by the preassociation of NH4+ counterions with [P2Mo18O62]6− anions. A less possible, but still present, mechanism is the electron transfer from the HOMO of water (−5.67 eV) to the LUMO of [P2Mo18O62]6− anions, especially due to preassociation of water molecules with POM anions. Similar observations are valid for the reduction of (NH4)6P2W18O62 films, whereas for the respective Keggin heteropolyacids a single redox mechanism from the Fermi level of Al to the LUMO of POMs occurs. Upon thermal treatment, the reoxidation of the highly reduced Wells–Dawson ammonium salts by dioxygen is observed.
On ITO substrates, the reduction of (NH4)6P2Mo18O62 films seems to proceed equally via electron transfer from the Fermi level of ITO (−4.7 eV) and the HOMO of NH4+ cations according to energetic considerations. Nevertheless, the electron transfer from the HOMO of NH4+ cations is possibly favored more, due to preassociation. Again the electron transfer from the HOMO of water to the LUMO of [P2Mo18O62]6− anions is a feasible, less possible process. In the Keggin heteropoly acids, the most likely reduction mechanism is the electron transfer from the Fermi level of ITO to the LUMO of POMs. Upon thermal treatment, initially the Wells–Dawson (NH4)6P2Mo18O62 film is thermally reduced (up to 2–4e− at 110 °C), whereas at a higher temperature it is reoxidized by dioxygen exhibiting a redox behavior similar to the one on Al substrates.
On the other hand, on SiO2 substrates, all POM films regardless of structure type and film thickness are not spontaneously reduced under ambient conditions. Upon thermal treatment, only the (NH4)6P2Mo18O62 film is reduced, possibly from the HOMO of ammonium counterions, since it is favored both energetically and by preassociation, and evidenced by FT-IR results as well. Again, the electron transfer from the HOMO of water is a feasible, less possible process. The inability of the other POM compounds to be reduced thermally onto SiO2 (under ambient conditions) could be explained as followingly. First, the H3PMo12O40 film and the corresponding H3PW12O40 film are not reduced thermally onto SiO2, possibly due to absence of ammonium counterions. In contrast, the (NH4)6P2W18O62 film is not reduced thermally on SiO2, mainly for kinetic reasons, i.e. the re-oxidation rate of the reduced [P2W18O62]n− (n > 6) anions by dioxygen is much higher than the reduction rate of [P2W18O62]6− anions.7,63 From the above, it is clear that the thermal reduction of POMs onto SiO2 can be attributed only to the oxidation of ammonium counterions and not to the direct reduction from the substrate, since the absence of thermal reduction of H3PMo12O40 film suggests against the second mechanism. (If the latter didn’t happen, then the reduced [PMo12O40]n− (n > 3) anions that are stable towards dioxygen, would have been detected spectroscopically.)7,9
4. Conclusions
We have demonstrated that the multi-electron reduction of films of Wells–Dawson ammonium salts, namely (NH4)6P2Mo18O62 and (NH4)6P2W18O62, on top of metallic and semiconducting substrates (Al, ITO) is affected not only by the relative position of the LUMO level of POMs with respect to the Fermi level of the substrate that would allow spontaneous electron transfer, but also by the presence of ammonium counterions, mostly in a synergistic way. In contrast, on dielectric substrates (SiO2) the reduction of Wells–Dawson POMs ((NH4)6P2Mo18O62) proceeds only through the oxidation of ammonium counterions. Thus, using a combination of optical and electronic spectroscopy techniques, we have unveiled the critical and synergistic role of ammonium counterions to the basic electron transfer mechanism of Wells–Dawson POMs (i.e. from the Fermi level of the substrate to the LUMO of POM anions) on top of metallic, semiconducting and dielectric substrates. For comparison, the respective Keggin heteropolyacids (H3PMo12O40, H3PW12O40) were also investigated, since in those heteropolyacids only the basic reduction mechanism takes place. In brief, the following observations were made for each substrate.
On Al substrates, the Wells–Dawson ammonium salts are spontaneously reduced to a higher degree (4–6e−) than the respective Keggin heteropolyacids (∼2e−) in accordance with the deeper lying LUMO level of the former POMs, as evidenced by both XPS and UV-Vis results. The POM film thickness seems to affect the reduction degree of POMs in an apparent way: the lower the POM film thickness the higher the reduction degree of POMs. Upon thermal treatment, the highly reduced Wells–Dawson ammonium salts are reoxidized by dioxygen. On ITO substrates, the thin POM films are spontaneously reduced, with the Wells–Dawson POMs (especially the (NH4)6P2Mo18O62) being more reduced than the Keggin POMs, as evidenced by XPS results. Upon thermal treatment, the thick (NH4)6P2Mo18O62 films (which were initially in oxidized state) are thermally reduced (2–4e−) up to ∼110 °C, whereas at a higher temperature they are re-oxidized by dioxygen exhibiting a thermal redox behavior similar to the one on Al substrates. On the other hand, on SiO2 substrates, all POM films studied regardless of structure type and film thickness are in oxidized state. Upon thermal treatment, only the (NH4)6P2Mo18O62 film is reduced on SiO2. The thermal reduction of (NH4)6P2Mo18O62 film on SiO2 proceeds possibly through the oxidation of NH4+ counterions (via hydrogen abstraction) by [P2Mo18O62]6− anions leading to the formation of NH3+˙ cation radicals and probably to highly oxidized, nitrogen-based products. Overall, the exploration of the mechanisms responsible for the multi-electron redox chemistry of POM films on top of various substrates investigated in the present work is expected to shed light to hidden aspects of this subject and definitely pave the way for a more effective and wider use of POMs in electronic and catalytic applications.
Conflicts of interest
The authors declare no competing financial interest.
Acknowledgements
The project “Implementing advanced interfacial engineering strategies for highly efficient hybrid solar cells” (Acronym: IMAGINE-HYSOL) is implemented under the “ARISTEIA II” Action of the “OPERATIONAL PROGRAMME EDUCATION AND LIFELONG LEARNING” and is co-funded by the European Social Fund (ESF) and Greek Resources.
References
-
M. T. Pope, Heteropoly and Isopoly Oxometalates, Springer-Verlag, Berlin, 1983 Search PubMed.
- C. L. Hill, Polyoxometalates (Special Issue), Chem. Rev., 1998, 98, 1–390 CrossRef CAS PubMed.
- S.-S. Wang and G.-Y. Yang, Recent Advances in Polyoxometalate-Catalyzed Reactions, Chem. Rev., 2015, 115, 4893–4962 CrossRef CAS PubMed.
- D.-L. Long, R. Tsunashima and L. Cronin, Polyoxometalates: Building Blocks for Functional Nanoscale Systems, Angew. Chem., Int. Ed., 2010, 49, 1736–1758 CrossRef CAS PubMed.
- T. Yamase, Photo- and Electrochromism of Polyoxometalates and Related Materials, Chem. Rev., 1998, 98, 307–325 CrossRef CAS PubMed.
-
C. L. Hill and C. M. Prosser-McCartha, Photocatalytic and Photoredox Properties of Polyoxometalate Systems, in Photosensitization and Photocatalysis using Inorganic and Organometallic Compounds, ed. K. Kalyanasundaram and M. Grätzel, Kluwer Academic Publishers, Dordrecht, 1993, pp. 307–330 Search PubMed.
- E. Papaconstantinou, Photochemistry of Polyoxometallates of Molybdenum and Tungsten and/or Vanadium, Chem. Soc. Rev., 1989, 18, 1–31 RSC.
- A. Mylonas, A. Hiskia, E. Androulaki, D. Dimotikali and E. Papaconstantinou, New Aspect of the Mechanism of Photocatalytic Oxidation of Organic Compounds by Polyoxometalates in Aqueous Solutions. The Selective Photooxidation of Propan-2-ol to Propanone: The Role of OH Radicals, Phys. Chem. Chem. Phys., 1999, 1, 437–440 RSC.
- C. Streb, New Trends in Polyoxometalate Photoredox Chemistry: From Photosensitisation to Water Oxidation Catalysis, Dalton Trans., 2012, 41, 1651–1659 RSC.
- Y. V. Geletii, B. Botar, P. Kögerler, D. A. Hillesheim, D. G. Musaev and C. L. Hill, An All-Inorganic, Stable, and Highly Active Tetraruthenium Homogeneous Catalyst for Water Oxidation, Angew. Chem., Int. Ed., 2008, 47, 3896–3899 CrossRef CAS PubMed.
- A. Sartorel, M. Carraro, G. Scorrano, R. De Zorzi, S. Geremia, N. D. McDaniel, S. Bernhard and M. Bonchio, Polyoxometalate Embedding of a Tetraruthenium(IV)-oxo-core by Template-Directed Metalation of [γ-SiW10O36]8-: A Totally Inorganic Oxygen-Evolving Catalyst, J. Am. Chem. Soc., 2008, 130, 5006–5007 CrossRef CAS PubMed.
- Q. Yin, J. M. Tan, C. Besson, Y. V. Geletii, D. G. Musaev, A. E. Kuznetsov, Z. Luo, K. I. Hardcastle and C. L. Hill, A Fast Soluble Carbon-Free Molecular Water Oxidation Catalyst Based on Abundant Metals, Science, 2010, 328, 342–345 CrossRef CAS PubMed.
- J. Niu, F. Li, J. Zhao, P. Ma, D. Zhang, B. Bassil, U. Kortz and J. Wang, Tetradecacobalt(II)-Containing 36-Niobate [Co14(OH)16(H2O)8Nb36O106]20− and Its Photocatalytic H2 Evolution Activity, Chem. – Eur. J., 2014, 20, 9852–9857 CrossRef CAS PubMed.
- M. Natali, I. Bazzan, S. Goberna-Ferrón, R. Al-Oweini, M. Ibrahim, B. S. Bassil, H. Dau, F. Scandola, J. R. Galán-Mascarós, U. Kortz, A. Sartorel, I. Zaharieva and M. Bonchio, Photo-assisted water oxidation by high-nuclearity cobalt-oxo cores: tracing the catalyst fate during oxygen evolution turnover, Green Chem., 2017, 19, 2416–2426 RSC.
- A. Panagiotopoulos, A. M. Douvas, P. Argitis and A. G. Coutsolelos, Porphyrin Sensitized Evolution of Hydrogen using Dawson and Keplerate Polyoxometalate Photocatalysts, ChemSusChem, 2016, 9, 3213–3219 CrossRef CAS PubMed.
- M. Sadakane and E. Steckhan, Electrochemical Properties of Polyoxometalates as Electrocatalysts, Chem. Rev., 1998, 98, 219–237 CrossRef CAS PubMed.
-
B. Keita and L. Nadjo, Electrochemistry of Isopoly and Heteropoly Oxometalates, in Encyclopedia of Electrochemistry, ed. A. J. Bard and M. Stratmann, Wiley-VCH, Weinheim, Germany, 2006, vol. 7, pp. 607–700 Search PubMed.
- B. Keita and L. Nadjo, Polyoxometalate-Based Homogeneous Catalysis of Electrode Reactions: Recent Achievements, J. Mol. Catal. A:
Chem., 2007, 262, 190–215 CrossRef CAS.
- C. L. Hill and C. M. Prosser-McCartha, Homogeneous catalysis by transition metal oxygen anion clusters, Coord. Chem. Rev., 1995, 143, 407–455 CrossRef CAS.
- X. Xi and S. Dong, Electrocatalytic reduction of nitrite using Dawson-type tungstodiphosphate anions in aqueous solutions, adsorbed on a glassy carbon electrode and doped in polypyrrole film, J. Mol. Catal. A: Chem., 1996, 114, 257–265 CrossRef CAS.
- B. Keita, D. Bouaziz and L. Nadjo, Solvent Effects on the Redox Potentials of Potassium 12-Tungstosilicate and 18-Tungstodiphosphate, J. Electrochem. Soc., 1988, 135, 87–91 CrossRef CAS.
- P. Gouzerh and M. Che, From Scheele and Berzelius to Müller: Polyoxometalates (POMs) Revisited and the “Missing Link” Between the Bottom Up and Top Down Approaches, Actual. Chim., 2006, 298, 9–22 CAS.
- C. Sanchez, J. Livage, J. P. Launay, M. Fournier and Y. Jeannin, Electron Delocalization in Mixed-Valence Molybdenum Polyanions, J. Am. Chem. Soc., 1982, 104, 3194–3202 CrossRef CAS.
- M. Vasilopoulou, A. M. Douvas, D. G. Georgiadou, L. C. Palilis, S. Kennou, L. Sygellou, A. Soultati, I. Kostis, G. Papadimitropoulos, D. Davazoglou and P. Argitis, The Influence of Hydrogenation and Oxygen Vacancies on Molybdenum Oxides Work Function and Gap States for Application in Organic optoelectronics, J. Am. Chem. Soc., 2012, 134, 16178–16187 CrossRef CAS PubMed.
- A. M. Douvas, E. Makarona, N. Glezos, P. Argitis, J. A. Mielczarski and E. Mielczarski, Polyoxometalate-Based Layered Structures for Charge Transport Control in Molecular Devices, ACS Nano, 2008, 2, 733–742 CrossRef CAS PubMed.
- L. C. Palilis, M. Vasilopoulou, D. G. Georgiadou and P. Argitis, A Water Soluble Inorganic Molecular Oxide as a Novel Efficient Electron Injection Layer for Hybrid Light-Emitting Diodes (HyLEDs), Org. Electron., 2010, 11, 887–894 CrossRef CAS.
- L. C. Palilis, M. Vasilopoulou, A. M. Douvas, D. G. Georgiadou, S. Kennou, N. A. Stathopoulos, V. Constantoudis and P. Argitis, Solution Processable Tungsten Polyoxometalate as Highly Effective Cathode Interlayer for Improved Efficiency and Stability Polymer Solar Cells, Sol. Energy Mater. Sol. Cells, 2013, 114, 205–213 CrossRef CAS.
- M. Vasilopoulou, A. M. Douvas, L. C. Palilis, S. Kennou and P. Argitis, Old metal Oxide Clusters in New Applications: Spontaneous Reduction of Keggin and Dawson Polyoxometalate Layers by a Metallic Electrode for Improving Efficiency in Organic Optoelectronics, J. Am. Chem. Soc., 2015, 137, 6844–6856 CrossRef CAS PubMed.
- M. Vasilopoulou, E. Polydorou, A. M. Douvas, L. C. Palilis, S. Kennou and P. Argitis, Annealing-Free Highly Crystalline Solution-Processed Molecular Metal Oxides for Efficient Single-Junction and Tandem Polymer Solar Cells, Energy Environ. Sci., 2015, 8, 2448–2463 RSC.
- M. Alaaeddine, Q. Zhu, D. Fichou, G. Izzet, J. E. Rault, N. Barrett, A. Proust and L. Tortech, Enhancement of photovoltaic efficiency by insertion of a polyoxometalate layer at the anode of an organic solar cell, Inorg. Chem. Front., 2014, 1, 682–688 RSC.
- S. Ohisa, S. Kagami, Y.-J. Pu, T. Chiba and J. Kido, A Solution-Processed Heteropoly Acid Containing MoO3 Units as a Hole-Injection Material for Highly Stable Organic Light-Emitting Devices, ACS Appl. Mater. Interfaces, 2016, 8, 20946–20954 CrossRef CAS PubMed.
- S. Ohisa, K. Endo, K. Kasuga, M. Suzuki, T. Chiba, Y.-J. Pu and J. Kido, Post-Treatment-Free Solution-Processed Reduced Phosphomolybdic Acid Containing Molybdenum Oxide Units for Efficient Hole-Injection Layers in Organic Light-Emitting Devices, Inorg. Chem., 2018, 57, 1950–1957 CrossRef CAS PubMed.
- G. Ji, Y. Wang, Q. Luo, K. Han, M. Xie, L. Zhang, N. Wu, J. Lin, S. Xiao, Y.-Q. Li, L.-Q. Luo and C.-Q. Ma, Fully Coated Semitransparent Organic Solar Cells with a Doctor-Blade-Coated Composite Anode Buffer Layer of Phosphomolybdic Acid and PEDOT:PSS and a Spray-Coated Silver Nanowire Top Electrode, ACS Appl. Mater. Interfaces, 2018, 10, 943–954 CrossRef CAS PubMed.
- H. Wu, Contribution to the Chemistry of Phosphomolybdic Acids, Phosphotungstic Acids, and Allied Substances, J. Biol. Chem., 1920, 43, 189–220 CAS.
-
G. A. Tsigdinos, in Heteropoly Compounds of Molybdenum and Tungsten, ed. M. J. S. Dewer, Topic in Current Chemistry, Springer-Verlag, New York, 1978, vol. 76, pp. l–64 Search PubMed.
- R. I. Buckley and R. J. H. Clark, Structural and Electronic Properties of some Polymolybdates Reducible to Molybdenum Blues, Coord. Chem. Rev., 1985, 65, 167–218 CrossRef CAS.
- C. Rocchiccioli-Deltcheff, M. Fournier, R. Franck and R. Thouvenot, Vibrational Investigations of Polyoxometalates. 2. Evidence for Anion-Anion Interactions in Molybdenum(VI) and Tungsten(VI) Compounds Related to the Keggin Structure, Inorg. Chem., 1983, 22, 207–216 CrossRef CAS.
- M. Fournier, R. Thouvenot and C. Rocchiccioli-Deltcheff, Catalysis by Polyoxometalates. Part 1. Supported Polyoxoanions of the Keggin Structure: Spectroscopic Study (IR, Raman, UV) of Solutions used for Impregnation, J. Chem. Soc., Faraday Trans., 1991, 87, 349–356 RSC.
- K. Eguchi, Y. Toyozawa, N. Yamazoe and T. Seiyama, An Infrared Study on the Reduction Processes of Dodecamolybdophosphates, J. Catal., 1983, 83, 32–41 CrossRef CAS.
- J. F. Garvey and M. T. Pope, Chirality of Oxidized and Reduced Octadecamolybdodiphosphate Anions. Observation of a Pfeiffer Effect, Inorg. Chem., 1978, 17, 1115–1118 CrossRef CAS.
- J. Hu, R. C. Burns and J.-P. Guerbois, The Solid-State Thermal Rearrangement of the Dawson Anion [P2Mo18O62]6− into a Keggin-Type [PMo12O40]3− – Containing Phase and their Reactivity in the Oxidative Dehydrogenation of Isobutyraldehyde, J. Mol. Catal. A: Chem., 2000, 152, 141–155 CrossRef CAS.
- T. Ueda, Y. Nishimoto, R. Saito, M. Ohnishi and J. Nambu, Vanadium(V)-Substitution Reactions of Wells–Dawson-Type Polyoxometalates: From [X2M18O62]6− (X = P, As; M = Mo, W) to [X2VM17O62]7−, Inorganics, 2015, 3, 355–369 CrossRef CAS.
- S. Himeno, M. Hashimoto and T. Ueda, Formation and conversion of molybdophosphate and -arsenate complexes in aqueous solution, Inorg. Chim. Acta, 1999, 284, 237–245 CrossRef CAS.
-
E. Pretsch, P. Bühlmann and C. Affolter, Structure Determination of Organic Compounds, Springer-Verlag, Berlin, Heidelberg, 3rd edn, 2000, pp. 268–279 Search PubMed.
- G. Jian, C. Yaguang, Q. Lunyu and L. Qin, Preparation and Characterization of New Peroxyniobium-Containing Phosphotungstates with Dawson Structures, Polyhedron, 1996, 15, 2273–2277 CrossRef.
- C. Comuzzi, G. Dolcetti, A. Trovarelli, F. Cavani, F. Trifirò, J. Llorca and R. G. Finke, The solid-state rearrangement of the Wells–Dawson K6P2W18O62·10H2O to a stable Keggin-type heteropolyanion phase: a catalyst for the selective oxidation of isobutane to isobutene, Catal. Lett., 1996, 36, 75–79 CrossRef CAS.
- R. Contant, M. Abbessi, R. Thouvenot and G. Herve, Dawson Type Heteropolyanions. 3. Syntheses and 31P, 51V, and 183W NMR Structural Investigation of Octadeca(molybdotungstovanado)diphosphates Related to the [H2P2W12O48]12− Anion, Inorg. Chem., 2004, 43, 3597–3604 CrossRef CAS PubMed.
- R. Contant, W. G. Klemperer and O. Yaghi, Potassium Octadecatungstodiphosphates(V) and Related Lacunary Compounds, Inorg. Synth., 1990, 27, 104–111 CAS.
- G. M. Varga, Jr., E. Papaconstantinou and M. T. Pope, Heteropoly Blues. IV. Spectroscopic and Magnetic Properties of Some Reduced Polytungstates, Inorg. Chem., 1970, 9, 662–667 CrossRef.
- E. Papaconstantinou and M. T. Pope, Heteropoly Blues. V. Electronic Spectra of One- to Six-Electron Blues of 18-Metallodiphosphate Anions, Inorg. Chem., 1970, 9, 667–669 CrossRef CAS.
- J. M. Fruchart, G. Herve, J. P. Launay and R. Massart, Electronic Spectra of Mixed Valence Reduced Heteropolyanions, J. Inorg. Nucl. Chem., 1976, 38, 1627–1634 CrossRef CAS.
- M. B. Robin and P. Day, Mixed Valence Chemistry-A survey and Classification, Adv. Inorg. Chem. Radiochem., 1967, 10, 247–422 CrossRef CAS.
- A. M. Douvas, A. Kapella, D. Dimotikali and P. Argitis, Photochemically-Induced Acid Generation from 18-Molybdodiphosphate and 18-Tungstodiphosphate within Poly(2-Hydroxyethyl Methacrylate) Films, Inorg. Chem., 2009, 48, 4896–4907 CrossRef CAS PubMed.
- J. M. Poblet, X. López and C. Bo,
Ab Initio and DFT Modelling of Complex Materials: Towards the Understanding of Electronic and Magnetic Properties of Polyoxometalates, Chem. Soc. Rev., 2003, 32, 297–308 RSC.
- X. López, C. Bo, J.-M. Poblet and J. P. Sarasa, Relative Stability in α- and β-Wells–Dawson Heteropolyanions: A DFT Study of [P2M18O62]n− (M = W and Mo) and [P2W15V3O62]n−, Inorg. Chem., 2003, 42, 2634–2638 CrossRef PubMed.
- N. Mizuno, K. Katamura, Y. Yoneda and M. Misono, Catalysis by Heteropoly Compounds. V. The Reduction Mechanism of H3PMo12O40, J. Catal., 1983, 83, 384–392 CrossRef CAS.
- H. Taketa, S. Katsuki, K. Eguchi, T. Seiyama and N. Yamazoe, Electronic Structure and Redox Mechanism of Dodecamolybdophosphate, J. Phys. Chem., 1986, 90, 2959–2962 CrossRef CAS.
- M. T. Greiner, L. Chai, M. G. Helander, W.-M. Tang and Z.-H. Lu, Transition Metal Oxide Work Functions: The Infl uence of Cation Oxidation State and Oxygen Vacancies, Adv. Funct. Mater., 2013, 23, 215–226 CrossRef CAS.
- H. N. Miras, J. Yan, D.-L. Long and L. Cronin, Engineering Polyoxometalates with Emergent Properties, Chem. Soc. Rev., 2012, 41, 7403–7430 RSC.
- L. Weinhardt, M. Blum, M. Bär, C. Heske, B. Cole, B. Marsen and E. L. Miller, Electronic Surface Level Positions of WO3 Thin Films for Photoelectrochemical Hydrogen Production, J. Phys. Chem. C, 2008, 112, 3078–3082 CrossRef CAS.
- C. Tanielian, K. Duffy and A. Jones, Kinetic and Mechanistic Aspects of Photocatalysis by Polyoxotungstates: A Laser Flash Photolysis, Pulse Radiolysis, and Continuous Photolysis Study, J. Phys. Chem. B, 1997, 101, 4276–4282 CrossRef CAS.
- G. Yang, J. Gong, R. Yang, H. Guo, Y. Wang, B. Liu and S. Dong, Modification of Electrode Surface Through Electrospinning Followed by Self-Assembly Multilayer Film of Polyoxometalate and its Photochromic, Electrochem. Commun., 2006, 8, 790–796 CrossRef CAS.
- A. Hiskia, A. Mylonas and E. Papaconstantinou, Comparison of the Photoredox Properties of Polyoxometallates and Semiconducting Particles, Chem. Soc. Rev., 2001, 30, 62–69 RSC.
Footnote |
† Electronic supplementary information (ESI) available: Additional figures (Fig. S1–S9) and tables (Table S1) and relevant discussion as noted in text. See DOI: 10.1039/c8cp07101b |
|
This journal is © the Owner Societies 2019 |