Strain-tunable magnetic and electronic properties of monolayer CrI3†
Received
15th November 2018
, Accepted 7th January 2019
First published on 7th January 2019
Abstract
Two-dimensional CrI3 has attracted much attention as it is reported to be a ferromagnetic semiconductor with a Curie temperature of around 45 K. By performing first-principles calculations, we find that the magnetic ground state of CrI3 is variable under biaxial strain. Our theoretical investigations show that the ground state of monolayer CrI3 is ferromagnetic under compression, but becomes antiferromagnetic under tension. Particularly, the transition occurs under a feasible in-plane strain of around 1.8%. Accompanied by the transition of the magnetic ground state, CrI3 undergoes a transition from magnetic-metal to half-metal to half-semiconductor to spin-relevant semiconductor when the strain varies from −15% to 10%. We attribute these transitions to the variation of the d-orbitals of Cr atoms and the p-orbitals of I atoms. Generally, we report a series of magnetic and electronic phase transitions in strained CrI3, which will help both theoretical and experimental researchers in further understanding the tunable electronic and magnetic properties of CrI3 and its analogs.
Introduction
Two-dimensional (2D) materials are of great concern theoretically and experimentally because of their unique electronic and optoelectronic properties.1,2 Typically, graphene, which consists of six carbon atoms in a honeycomb lattice, is a zero-gap semimetal with the carrier mobility being reported up to ∼105 cm2 V−1 s−1.3 When it is chemically decorated with added atoms or cut into 1D nanoribbons, it will exhibit tunable electronic and magnetic properties.4–6 When combined with other 2D materials to form van der Waals (vdW) heterostructures,7 it would exhibit much more interesting physical properties.6,8 Besides graphene, other 2D materials from semiconducting black phosphorus9 to transition metal dichalcogenides10–12 to insulating hexagonal boron nitride13 have also been identified as important candidates for the post-silicon electronic and optical devices.14 However, none of them is reported to exhibit intrinsic magnetism, which limits their application in spintronics.15 Theorists predicted that most 2D materials are nonmagnetic because the thermal fluctuations at finite temperature would break the spontaneous symmetry.16 However, a composite of monolayer Cr2Ge2Te6 has been reported to be ferromagnetic recently, which is regardless of the restriction.17 The rise of Cr2Ge2Te6 paves a new way to search for the long-range Ising ferromagnetism in atomically thin 2D materials, where an intrinsic magnetocrystalline material could exist because of the reduction of the crystal symmetry.18 Very recently, another ferromagnetic semiconductor chromium triiodide (CrI3) appeared in the research field again19 because of its high Curie temperature in the monolayer.20 Upon carrier doping, room-temperature magnetism is observed in CrI3 due to its flat band structure.21–23 Both experimental and theoretical research shows that monolayer CrI3 is a ferromagnetic semiconductor.18,24 When increasing the number of layers, the ferromagnetic order persists within each layer, but the antiferromagnetic coupling dominates different layers.8 Moreover, the ferromagnetic and antiferromagnetic states can be switched on and off by changing the external gate voltage.18 When CrI3 forms heterostructures with other 2D materials like graphene, it also exhibits some topological insulating properties.25 However, all this is concluded from the fact that CrI3 is a ferromagnetic semiconductor with an equilibrium lattice constant.20
On the other hand, strain plays an important role in determining the physical properties of 2D materials. Considering the fact that typically CrI3 is transferred on the substrate of SiO2 and on other 2D materials after exfoliation,20 the intrinsic physical properties of CrI3 would be affected by the substrate due to the lattice-mismatch-induced strain.26,27 There are some studies on the physical properties of CrI3 under strain, but they are based on the assumption of a robust ferromagnetic ground state.28 Meanwhile, it is challenging for experimental researchers to identify the magnetic order of the monolayer in the atomic resolution. Here, we wish to identify the magnetic ground states of CrI3 under strain via ab initio first-principles calculations.
In this work, we present a systematic study on the tunable electronic and magnetic properties of monolayer CrI3 under strain. Our results show that the Cr atoms in the unit cell are ferromagnetically aligned under compression strain, and CrI3 retains the magnetic order up to a maximum tension strain of around 2%, then it dramatically becomes an antiferromagnetic half-semiconductor when the tension strain is further increased. During this transition, the magnetic moment on Cr atoms increases, and CrI3 undergoes a transition from magnetic-metal to half-metal to half-semiconductor, owing to the variation of the d-orbitals of Cr atoms and the p-orbitals of I atoms. Our results will provide a new way to understand the magnetic ground state in monolayer CrI3 and its analogs, which is useful for the design of spintronic devices29 based on ferromagnetic semiconductors.17,30–32
Results and discussion
The atomic structure of monolayer CrI3 is shown in Fig. 1a, which can be simply imaged as a
super cell of 1T SnS2 with one point vacancy of Sn atoms.33–35 It belongs to space group C2/m containing two formula units.36 Its optimized lattice parameters are calculated to be a = b = 6.978 Å and c = 21.476 Å, which are in good agreement with the X-ray diffraction data37 and previous DFT results.24 Both spin-unpolarized and spin-polarized calculations are performed to get an overview of the ground state of monolayer CrI3. Our result shows that the ferromagnetic (FM) state is more favorable in energy, which is 63 meV lower than the antiferromagnetic (AFM) state, indicating stable ferromagnetism at room-temperature. Thus, the intrinsic electronic and magnetic properties of CrI3 can be represented by the FM state as shown in Fig. 1b and c. The spin-unpolarized band structure shows that nonmagnetic CrI3 is a metal with several bands crossing through the Fermi level. Upon considering the spin-polarization, the degenerated bands get split, resulting in an indirect energy gap of 1.124 eV and 2.169 eV for the spin-up (solid) and -down (dashed) electrons, respectively. It is found that the spin-polarized electrons in monolayer CrI3 exhibit anisotropic transport properties. For the spin-up electrons, the conduction band minimum (CBM) and valence band maximum (VBM) are located at the Gamma point and in the line from gamma to M; for the spin-down electrons, the indirect gap originates from M and gamma points, respectively. It is also noted that the conduction and valence band edges around the Fermi level are fully spin-polarized and exclusively occupied by electrons with the same spin component, rendering a typical half-semiconductor character. Moreover, the CBM and VBM of the spin-up electrons are contributed by both Cr and I atoms, while the CBM and VBM of the spin-down electrons are contributed by Cr atoms and I atoms, respectively. Our conclusion of the intrinsic electronic structure of monolayer CrI3 is further confirmed by the density of state (DOS) calculations, where sharp peaks composed of hybrid states appear around the Fermi level, suggesting strongly localized states. As a result, electrons are bound in these states and the carrier mobility of CrI3 is very slow, which can be concluded directly from the nearly flat bands around the Fermi level. Thus, in some vdW heterostructures CrI3 is usually used as a FM substrate to generate spin-polarized electrons.7
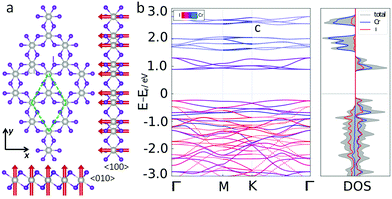 |
| Fig. 1 Atomic and electronic structure of unstrained monolayer CrI3. (a) Structural schematic of CrI3 with top, front and right views. The green dashed line presents the unit cell and the red vectors indicate the spin configuration. (b) Band structure of ferromagnetic CrI3. The Fermi level is set to zero, and the color indicates the attribution of Cr and I atoms. (c) Density of states of CrI3 obtained by spin-polarized calculations. | |
We have shown above that pristine CrI3 is a ferromagnetic semiconductor with the magnetic moment on Cr atoms being 3.106 μB.19,38 However, these 2D materials are usually supported by the substrate in device design. Besides the interlayer interaction, strain induced by lattice mismatch and lattice orientation is the most common case in these 2D materials. We noted in a very recent work that when a non-collinear spin configuration is introduced by considering the spin orbital coupling effect, monolayer CrI3 will undergo a transition from the FM to AFM state under compression.39 Here, we would like to show novel electronic and magnetic properties of monolayer CrI3 under biaxial strain with a collinear spin configuration. The total energies are calculated using spin-polarized calculations for both FM and AFM configurations. It shows typically parabolic characters as in Fig. 2a when biaxial strain is applied. For the AFM configuration, its equilibrium lattice constant is slightly larger than that of the FM configuration. Under compression strain, the FM configuration prefers a much lower energy. To clearly show the variation of the total energy, we further plot the energy difference ΔE = EAFM − EFM as a function of biaxial strain in Fig. 2b, where EAFM and EFM are the total free energies of the monolayer with AFM and FM configurations, respectively. Within a reasonable range from −10% to 10%, ΔE decreases monotonously and drops down to zero around 1.8%, indicating a possible transition from FM to AFM. Taking the tensile strain of 3% as an example, the corresponding ΔE is 64 meV, which is twice larger than that calculated from the fluctuation of 300 K, suggesting stable AFM states at room temperature. When tension strain is further increased, the AFM configuration becomes much more stable. To confirm our conclusion, we performed further calculations with DFT+U, which usually give better results for transition metals with d orbitals.40,41 The parameters J and U are chosen to be 0.7 eV and 2.7 eV, respectively, which have shown great success in predicting the magnetic anisotropic properties of monolayer CrI3.39,42 We listed the total energies in Table S1 of the ESI.† One can clearly see that though the total energy of monolayer CrI3 is higher than that of the standard DFT results, the relative variation trends of ΔE, EAFM and EFM are the same. As a result, the transition from the FM to AFM state occurs when the tension strain is applied.
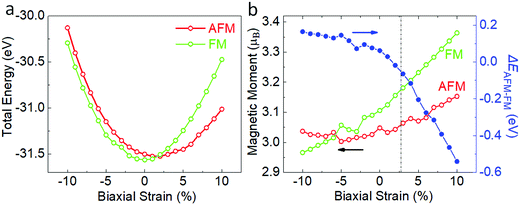 |
| Fig. 2 Ground state of monolayer CrI3 under biaxial strain. (a) Strain effect on the total energy of magnetic CrI3. (b) Energy difference and magnetic moment of FM and AFM CrI3 as a function of strain. Red and green symbols present the data of AFM and FM configurations, respectively. | |
AFM CrI3 is reported to be stable under tensile strain in previous work, but their physical properties are less studied. The band structure of AFM CrI3 plotted in Fig. 3 shows that tensed CrI3 is an indirect gap spin-relevant semiconductor with the VBM and CBM at the K and M points, respectively. Different from that of the FM state, spin-polarized electrons degenerate in AFM CrI3 as the inversion symmetry is preserved. As a result, spin-polarized electrons are strongly localized as seen from the nearly flat bands both around 1.0 and 2.0 eV, which disperse with an energy window of up to 0.5 eV in the FM configuration. The high degeneracy of the spin-polarized electrons is confirmed by the DOS as well, where the spin-up and -down electrons show identical distribution with mirror symmetry. Detailed analysis shows that though the contribution of the band structure is from the same atoms as FM CrI3, the non-degenerated bands at some highly symmetric K points get split, indicating the symmetry breaking of the px and py orbitals in I atoms. It is also noted that when biaxial strain is applied, the magnetic moment on the Cr atoms can be tuned by biaxial strain. When in-plane strain is applied from the compression to the tension region, the magnetic moment on Cr atoms increases for both FM and AFM configurations. Remarkably, the magnetic moment on the Cr atoms increases from 2.966 μB to 3.364 μB for FM CrI3 when the strain varies from −10% to 10%. But the slope of the magnetic moment variation is much smaller in the AFM case.
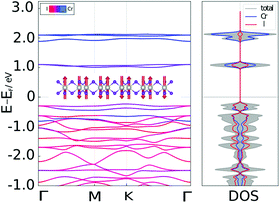 |
| Fig. 3 Electronic structure of stretched CrI3. Band structure and DOS of CrI3 with a tension strain of 4%. The inset shows the spin configuration with the antiferromagnetic order. The Fermi level is set to zero. | |
In addition to the transition and modulation of the magnetic state, the electronic properties of CrI3 show an interesting response to the external strain. We show the band gap of CrI3 for both spin-up and -down electrons in Fig. 4. The left and right panels show the band gap modulation in the FM and AFM states, respectively. For the masked section, we will neglect the variation of the band gaps as the magnetic ground state has changed. It is obvious that the FM state is more sensitive to the biaxial strain as the slope of the modulated band gap is sharper than that of the AFM state. In the non-strained case (left panel), both spin-up and -down electrons open a gap showing the character of a half-semiconductor (HS); when a biaxial compression smaller than 13% is applied, the band gaps of both the spin-up and -down electrons decrease, as the band gap of spin-down electrons is larger than that of the spin-up electrons, at a critical value around 14%, the spin-up gap becomes zero, while the spin-down gap remains open with 0.103 eV, making CrI3 a half-metal (HM); upon further compressing the monolayer, both the spin-up and -down bands close, and CrI3 becomes a magnetic-metal (MM). In contrast, when tension strain is applied in a reasonable range (right panel), AFM CrI3 shows spin-relevant semiconductor (SS) characters. Both spin-up and -down electrons occupy the same band and the band gap drops down in a slow slope around 0.015 eV/1%. Even when CrI3 is stretched by 10%, it remains open with a large band gap of 1.189 eV, suggesting that CrI3 is a robust AFM-SS when it is under tensile strain. The transition is further confirmed by our benchmark calculations of DFT+U as shown in Fig. S1 in the ESI.†
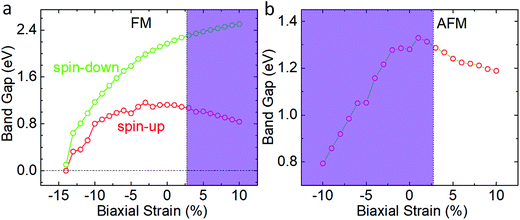 |
| Fig. 4 Strain-dependent band gaps in CrI3. (a and b) spin-relevant band gaps as a function of biaxial strain. Blue and green represent spin-up and -down electrons, respectively. The shadow indicates the artificial band gap modulation in the FM or AFM configuration. | |
To understand the mechanism of the biaxial-strain-induced electronic phase transition from MM to HM to HS to SS, we examined the projected density of states (PDOS) of CrI3 under various strains as shown in Fig. 5. Our result shows that the low-energy electronic properties of CrI3 are mainly attributed to the in-plane components of the d orbitals of Cr atoms and the p orbitals of I atoms. And the px and py orbitals of I atoms are degenerated, which explains the degenerated VBs at some highly symmetric K points in FM CrI3. On the other hand, the PDOS of Cr atoms is much higher than that of I atoms above the Fermi level, suggesting that the transport properties of electrons are dominated by the variation of the d-orbitals of Cr atoms, while the electronic properties of holes are determined by the px and py orbitals of I atoms. When the compression strain increases, the PDOS of all d-orbitals of Cr atoms shift downwards with a slightly decreasing gap for both the spin-up and spin-down electrons. However, the px and py orbitals of I atoms are much more sensitive to the biaxial strain, and the p orbitals at the conduction and valence region become hybridized, resulting in a closed gap. Specifically, at a compression strain state of −15%, all the d and p orbitals show peaks at the Fermi level; when the compression strain decreases to −14%, only the py orbitals of the spin-up electrons occupy the Fermi level, resulting in a MM–HM transition. For stretched CrI3 with a tensile strain of 10%, the peaks of the PDOS from the px orbital of I atoms are enhanced and the conduction region is contributed by two Cr atoms with mirror symmetry. We also show the spin density of CrI3 at the bottom of Fig. 5. As the compression strain decreases from −15% to −10%, the spin density represented by the iso-surface increases slightly, which is in good agreement with the magnetic moment modulation in Fig. 2. To this end, we have shown that the electronic and magnetic properties of monolayer CrI3 can be effectively tuned by biaxial strain, which is dominated by the dxy, dyz, dz2 and dxz orbitals of Cr atoms and the px and py orbitals of I atoms.
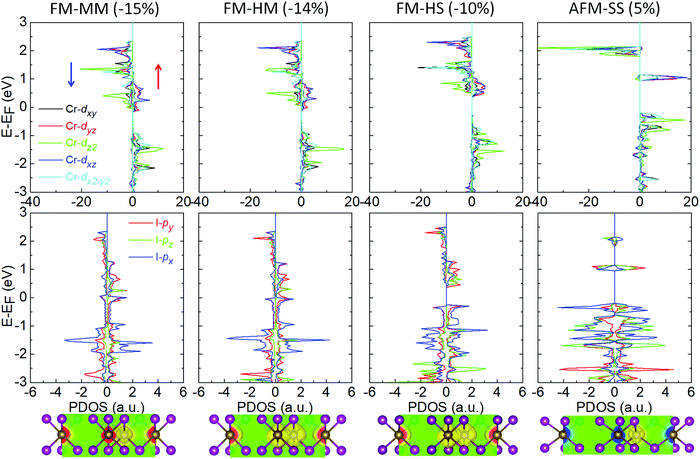 |
| Fig. 5 Projected density of states and spin density at different strains for CrI3 showing the MM–HM–HS transition. The scale bar in the slice of the spin density is set from −0.04 e Å−3 to 0.04 e Å−3. | |
Conclusions
In summary, we have systematically investigated the electronic and magnetic modulations of monolayer CrI3 under biaxial strain by first-principles calculations. The applied strain yields a pronounced transition of the magnetic ground state between FM and AFM. When compression strain is applied, CrI3 remains ferromagnetic. As the strain increases from −15% to 2%, a series of electronic phase transitions of MM–HM–HS–SS occur. In contrast, it becomes antiferromagnetic under tensile strain and the band gap of AFM CrI3 is robust against external strain. These modulations of electronic and magnetic properties stem from the shift of the d-orbitals in Cr atoms and the p-orbitals in I atoms under strain. The tunable electronic and magnetic properties of monolayer CrI3 investigated in this work are helpful in understanding the magnetism in CrI3 and its analogs observed by experimental researchers and would inspire extensive research interest in modulation of the electronic and magnetic properties in ferromagnetic semiconductors.
Computational methods
All our simulations were carried out by performing spin-polarized density functional theory (DFT) calculations as implemented in the Vienna ab initio Simulation Package (VASP).43 The Perdew–Burke–Ernzerhof (PBE) pseudopotentials44 within the general gradient approximation (GGA)45 were used to describe the electron exchange and correlation interactions and the energy cutoff was set at 520 eV. The Brillouin zone was represented by a 12 × 12 × 1 mesh for geometry optimization and the total energy calculation. And for the DOS calculation, a much denser grid of 24 × 24 × 1 was used. The atomic structure was fully relaxed with the energy convergence being 10−5 eV. To avoid the interlayer interaction between adjacent images, the vacuum was set to be 21 Å normal to the monolayer.
Conflicts of interest
There are no conflicts to declare.
Acknowledgements
This work is supported by the National Key R&D Program of China (Grant No. 2018FYA0305800) and the Dutch Science Foundation NWO/FOM 16PR1024. Yu acknowledges financial support from the NSFC grant (No. U1530401) and MOST 2017YFA0303404 from the Beijing Computational Science Research Center. Numerical calculations presented in this paper have been performed on a supercomputing system in the Supercomputing Center of Wuhan University.
References
- A. K. Geim and K. S. Novoselov, The rise of graphene, Nat. Mater., 2007, 6(3), 183–191 CrossRef CAS PubMed
.
- A. K. Geim, Graphene: Status and Prospects, Science, 2009, 324, 1530–1534 CrossRef PubMed
.
- K. S. Novoselov, A. K. Geim, S. V. Morozov, D. Jiang, Y. Zhang, S. V. Dubonos, I. V. Grigorieva and A. A. Firsov, Electric Field Effect in Atomically Thin Carbon Films, Science, 2004, 306, 666–669 CrossRef CAS PubMed
.
- Y. W. Son, M. L. Cohen and S. G. Louie, Half-metallic graphene nanoribbons, Nature, 2006, 444(7117), 347–349 CrossRef CAS PubMed
.
- J. Yu and W. Guo, A New Paradigm to Half-Metallicity in Graphene Nanoribbons, J. Phys. Lett., 2013, 4(6), 951–955 CAS
.
- D. R. Klein, D. MacNeill, J. L. Lado, D. Soriano, E. Navarro-Moratalla, K. Watanabe, T. Taniguchi, S. Manni, P. Canfield, J. Fernández-Rossier and P. Jarillo-Herrero, Probing magnetism in 2D van der Waals crystalline insulators via electron tunneling, Science, 2018, 360, 1218–1222 CrossRef CAS PubMed
.
- D. Zhong, K. L. Seyler, X. Linpeng, R. Cheng, N. Sivadas, B. Huang, E. Schmidgall, T. Taniguchi, K. Watanabe, M. A. McGuire, W. Yao, D. Xiao, K.-M. C. Fu and X. Xu, van der Waals engineering of ferromagnetic semiconductor heterostructures for spin and valleytronics, Sci. Adv., 2017, 3 Search PubMed
.
- B. Huang, G. Clark, E. Navarro-Moratalla, D. R. Klein, R. Cheng, K. L. Seyler, D. Zhong, E. Schmidgall, M. A. McGuire, D. H. Cobden, W. Yao, D. Xiao, P. Jarillo-Herrero and X. Xu, Layer-dependent ferromagnetism in a van der Waals crystal down to the monolayer limit, Nature, 2017, 546(7657), 270–273 CrossRef CAS PubMed
.
- L. Li, Y. Yu, G. J. Ye, Q. Ge, X. Ou, H. Wu, D. Feng, X. H. Chen and Y. Zhang, Black phosphorus field-effect transistors, Nat. Nanotechnol., 2014, 9(5), 372–377 CrossRef CAS PubMed
.
- Q. H. Wang, K. Kalantar-Zadeh, A. Kis, J. N. Coleman and M. S. Strano, Electronics and optoelectronics of two-dimensional transition metal dichalcogenides, Nat. Nanotechnol., 2012, 7, 699 CrossRef CAS PubMed
.
- H. Zhong, R. Quhe, Y. Wang, Z. Ni, M. Ye, Z. Song, Y. Pan, J. Yang, L. Yang, M. Lei, J. Shi and J. Lu, Interfacial Properties of Monolayer and Bilayer MoS2 Contacts with Metals: Beyond the Energy Band Calculations, Sci. Rep., 2016, 6, 21786 CrossRef CAS PubMed
.
- J. He and S. Li, Two-dimensional Janus transition-metal dichalcogenides with intrinsic ferromagnetism and half-metallicity, Comput. Mater. Sci., 2018, 152, 151–157 CrossRef CAS
.
- L. Song, L. Ci, H. Lu, P. B. Sorokin, C. Jin, J. Ni, A. G. Kvashnin, D. G. Kvashnin, J. Lou, B. I. Yakobson and P. M. Ajayan, Large Scale Growth and Characterization of Atomic Hexagonal Boron Nitride Layers, Nano Lett., 2010, 10(8), 3209–3215 CrossRef CAS PubMed
.
- S. Z. Butler, S. M. Hollen, L. Cao, Y. Cui, J. A. Gupta, H. R. Gutiérrez, T. F. Heinz, S. S. Hong, J. Huang, A. F. Ismach, E. Johnston-Halperin, M. Kuno, V. V. Plashnitsa, R. D. Robinson, R. S. Ruoff, S. Salahuddin, J. Shan, L. Shi, M. G. Spencer, M. Terrones, W. Windl and J. E. Goldberger, Progress, Challenges, and Opportunities in Two-Dimensional Materials Beyond Graphene, ACS Nano, 2013, 7(4), 2898–2926 CrossRef CAS PubMed
.
- Z. Li, T. Cao and S. G. Louie, Two-dimensional ferromagnetism in few-layer van der Waals crystals: renormalized spin-wave theory and calculations, J. Magn. Magn. Mater., 2018, 463, 28–35 CrossRef CAS
.
- N. D. Mermin and H. Wagner, Absence of Ferromagnetism or Antiferromagnetism in One- or Two-Dimensional Isotropic Heisenberg Models, Phys. Rev. Lett., 1966, 17(22), 1133–1136 CrossRef CAS
.
- D. Yang, W. Yao, Q. Chen, K. Peng, P. Jiang, X. Lu, C. Uher, T. Yang, G. Wang and X. Zhou, Cr2Ge2Te6: High Thermoelectric Performance from Layered Structure with High Symmetry, Chem. Mater., 2016, 28(6), 1611–1615 CrossRef CAS
.
- W.-B. Zhang, Q. Qu, P. Zhu and C.-H. Lam, Robust intrinsic ferromagnetism and half semiconductivity in stable two-dimensional single-layer chromium trihalides, J. Mater. Chem. C, 2015, 3(48), 12457–12468 RSC
.
- J. F. D. Jr. and C. E. Olson, Magnetization, Resonance, and Optical Properties of the Ferromagnet CrI3, J. Appl. Phys., 1965, 36(3), 1259–1260 CrossRef
.
- J. Liu, Q. Sun, Y. Kawazoe and P. Jena, Exfoliating biocompatible ferromagnetic Cr-trihalide monolayers, Phys. Chem. Chem. Phys., 2016, 18(13), 8777–8784 RSC
.
- H. Wang, F. Fan, S. Zhu and H. Wu, Doping enhanced ferromagnetism and induced half-metallicity in CrI3 monolayer, EPL, 2016, 114(4), 47001 CrossRef
.
- S. Jiang, L. Li, Z. Wang, K. F. Mak and J. Shan, Controlling magnetism in 2D CrI3 by electrostatic doping, Nat. Nanotechnol., 2018, 13(7), 549–553 CrossRef CAS PubMed
.
- L. Webster and J.-A. Yan, Strain-tunable magnetic anisotropy in monolayer CrCl3, CrBr3, and CrI3, Phys. Rev. B, 2018, 98(14), 144411 CrossRef
.
- H. Wang, V. Eyert and U. Schwingenschlogl, Electronic structure and magnetic ordering of the semiconducting chromium trihalides CrCl3, CrBr3, and CrI3, J. Phys.: Condens. Matter, 2011, 23(11), 116003 CrossRef CAS PubMed
.
- Q. Tong, F. Liu, J. Xiao and W. Yao, Skyrmions in the Moiré of van der Waals 2D Magnets, Nano Lett., 2018, 18(11), 7194–7199 CrossRef CAS PubMed
.
- J. Zhao, H. Liu, Z. Yu, R. Quhe, S. Zhou, Y. Wang, C. C. Liu, H. Zhong, N. Han, J. Lu, Y. Yao and K. Wu, Rise of silicene: a competitive 2D material, Prog. Mater. Sci., 2016, 83, 24–151 CrossRef CAS
.
- X. Peng, Q. Wei and A. Copple, Strain-engineered direct-indirect band gap transition and its mechanism in two-dimensional phosphorene, Phys. Rev. B: Condens. Matter Mater. Phys., 2014, 90(8), 085402 CrossRef
.
- F. Zheng, J. Zhao, Z. Liu, M. Li, M. Zhou, S. Zhang and P. Zhang, Tunable spin states in the two-dimensional magnet CrI3, Nanoscale, 2018, 10(29), 14298–14303 RSC
.
- S. A. Wolf, D. D. Awschalom, R. A. Buhrman, J. M. Daughton, S. von Molnár, M. L. Roukes, A. Y. Chtchelkanova and D. M. Treger, Spintronics: A Spin-Based Electronics Vision for the Future, Science, 2001, 294(5546), 1488 CrossRef CAS PubMed
.
- W. Han, R. K. Kawakami, M. Gmitra and J. Fabian, Graphene spintronics, Nat. Nanotechnol., 2014, 9(10), 794–807 CrossRef CAS PubMed
.
- P. Jiang, L. Li, Z. Liao, Y. X. Zhao and Z. Zhong, Spin Direction-Controlled Electronic Band Structure in Two-Dimensional Ferromagnetic CrI3, Nano Lett., 2018, 18(6), 3844–3849 CrossRef CAS PubMed
.
- V. Carteaux, D. Brunet, G. Ouvrard and G. Andre, Crystallographic, magnetic and electronic structures of a new layered ferromagnetic compound Cr2Ge2Te6, J. Phys.: Condens. Matter, 1995, 7(1), 69 CrossRef CAS
.
- B. Qu, C. Ma, G. Ji, C. Xu, J. Xu, Y. S. Meng, T. Wang and J. Y. Lee, Layered SnS2-Reduced Graphene Oxide Composite – A High-Capacity, High-Rate, and Long-Cycle Life Sodium-Ion Battery Anode Material, Adv. Mater., 2014, 26(23), 3854–3859 CrossRef CAS PubMed
.
- J.-w. Seo, J.-t. Jang, S.-w. Park, C. Kim, B. Park and J. Cheon, Two-Dimensional SnS2 Nanoplates with Extraordinary High Discharge Capacity for Lithium Ion Batteries, Adv. Mater., 2008, 20(22), 4269–4273 CrossRef CAS
.
- J. Yu, E. van Veen, M. I. Katsnelson and S. J. Yuan, Effective lattice Hamiltonian for monolayer tin disulfide: tailoring electronic structure with electric and magnetic fields, Phys. Rev. B, 2018, 97(24), 245410 CrossRef
.
- M. A. McGuire, H. Dixit, V. R. Cooper and B. C. Sales, Coupling of Crystal Structure and Magnetism in the Layered, Ferromagnetic Insulator CrI3, Chem. Mater., 2015, 27(2), 612–620 CrossRef CAS
.
- A. Frisk, L. B. Duffy, S. Zhang, G. van der Laan and T. Hesjedal, Magnetic X-ray spectroscopy of two-dimensional CrI3 layers, Mater. Lett., 2018, 232, 5–7 CrossRef CAS
.
- B. Rajeswaran, D. I. Khomskii, A. K. Zvezdin, C. N. R. Rao and A. Sundaresan, Field-induced polar order at the Néel temperature of chromium in rare-earth orthochromites: interplay of rare-earth and Cr magnetism, Phys. Rev. B: Condens. Matter Mater. Phys., 2012, 86(21), 214409 CrossRef
.
- L. Webster and J. A. Yan, Strain-tunable magnetic anisotropy in monolayer CrCl3, CrBr3, and CrI3, Phys. Rev. B, 2018, 98(14), 144411 CrossRef
.
- P. Guss, M. E. Foster, B. M. Wong, F. P. Doty, K. Shah, M. R. Squillante, U. Shirwadkar, R. Hawrami, J. Tower and D. Yuan, Results for aliovalent doping of CeBr3 with Ca2+, J. Appl. Phys., 2014, 115(3), 034908 CrossRef
.
- V. I. Anisimov, F. Aryasetiawan and A. I. Lichtenstein, First-principles calculations of the electronic structure and spectra of strongly correlated systems: the LDA+U method, J. Phys.: Condens. Matter, 1997, 9(4), 767–808 CrossRef CAS
.
- J. L. Lado and J. Fernandez-Rossier, On the origin of magnetic anisotropy in two dimensional CrI3, 2D Mater., 2017, 4, 3 Search PubMed
.
- J. Hafner,
Ab-initio simulations of materials using VASP: density-functional theory and beyond, J. Comput. Chem., 2008, 29(13), 2044–2078 CrossRef CAS PubMed
.
- B. Hammer, L. B. Hansen and J. K. Nørskov, Improved adsorption energetics within density-functional theory using revised Perdew–Burke–Ernzerhof functionals, Phys. Rev. B: Condens. Matter Mater. Phys., 1999, 59(11), 7413–7421 CrossRef
.
- J. P. Perdew, K. Burke and M. Ernzerhof, Generalized Gradient Approximation Made Simple, Phys. Rev. Lett., 1996, 77(18), 3865–3868 CrossRef CAS PubMed
.
Footnote |
† Electronic supplementary information (ESI) available. See DOI: 10.1039/c8cp07067a |
|
This journal is © the Owner Societies 2019 |
Click here to see how this site uses Cookies. View our privacy policy here.