Optical properties of pyridine adsorbed polycyclic aromatic hydrocarbons using quantum chemical calculations†
Received
30th October 2018
, Accepted 28th November 2018
First published on 10th December 2018
Abstract
Polycyclic aromatic hydrocarbons (PAHs), the molecular version of graphene, having edges saturated with hydrogen atoms, have recently emerged as a novel nanoplasmonic material. In this work, we investigate the optical properties of pristine and pyridine adsorbed circular and triangular PAHs. We base our calculations on computationally efficient first-principles time-dependent density-functional theory (TD-DFT) calculations. We find substantial changes in the optical absorption spectra induced by the presence of the pyridine molecule. In addition, with the help of electron difference density (EDD) maps, we demonstrate a strong optical interaction between PAHs and pyridine molecules. The main effect of pyridine adsorption is to split the plasmon band and to redistribute the optical absorption in a wider energy range. We believe that our findings can help in the design of novel plasmonic devices having PAHs as basic building blocks.
Introduction
Plasmons, the collective oscillations of valence electrons in conducting materials, have the ability to confine light to nanometric spatial regions, where the optical field intensity is enhanced by several orders of magnitude.1–3 This feature has been widely employed for applications in areas such as optical sensing4–7 and photo-catalysis,8–11 to list a few. Graphene has recently surfaced as a powerful plasmonic material12–18 offering unique advantages of being electrically,19–21 magnetically22–24 and optically25–28 tunable while displaying long excitation lifetimes and reduced losses,29 making it amenable to display novel plasmonic properties. While graphene plasmons have been mainly investigated at lower frequencies (mid-infrared range) of the electromagnetic spectrum, extending to the higher frequencies (UV-vis spectral range) is highly demanding.25 Results from recent TD-DFT calculations show that this can be possible via tailor-made graphene molecules known as PAHs.30–36 They support molecular plasmons having outstanding structural and electrical tunability, demonstrated by a broad range of theoretical methods.31,37,38 For example, Garcia de Abajo and coworkers showed that PAHs exhibit molecular plasmons37 having remarkable structural and electrical tunability, much higher than graphene, allowing them to be switched on/off by addition or removal of a single electron.31 At the same time, Guidez and coworkers found the dominant band in the low-energy region of the PAH spectrum as the molecular plasmon, confirmed from the induced electron density profiles.38
In a different context, many factors affect the interaction of light with molecules adjacent to a plasmonic structure.39–42 The molecules that can be chemisorbed (such as pyridine) on the nanostructure surface show the largest local field enhancement in comparison to those that can be physisorbed (such as benzene).43 Since the analyte molecules are much smaller than the optical wavelength, their interaction with light is very weak. Luckily, the huge local field enhancement generated by plasmons offers a solution to increase this interaction. By exposing the analyte molecules to the plasmons, their ability to absorb light is greatly improved.44 This is the working principle of the techniques known as surface-enhanced infrared absorption and surface-enhanced Raman scattering. While the PAH molecular plasmons are well studied and understood theoretically, their interactions with molecules, to our knowledge, have not been addressed previously. Therefore, in the present work, using state-of-the-art TD-DFT calculations, we investigate the optical properties of different PAHs with respect to the changes in size and shape and due to the adsorption of pyridine molecules.
Computational aspects
All the calculations in this work were performed using the ORCA DFT/TD-DFT software (version 4.0).45 We address both pristine and pyridine adsorbed PAHs having circular and triangular geometries. We consider the following circular PAHs: C1 (C24H12), C2 (C54H18), C3 (C96H24), C4 (C150H30) and C5 (C216H36) and the following triangular PAHs: T1 (C22H14), T2 (C78H24), T3 (C141H33), T4 (C222H42) and T5 (C321H51). Structural optimizations were performed using the Perdew–Burke–Ernzerhof (PBE) exchange–correlation functional and the def2-TZVP basis set (more details available in the ORCA online manual). The particular combination “PBE/def2-TZVP” was found optimal for structure optimization in terms of accuracy and performance. We employed a tight convergence criterion for the structural optimization by setting SCF convergence to 10−8, gradient convergence to 10−6 and energy convergence to 10−6. Optical absorption spectra are obtained by solving the time-dependent Kohn–Sham equations using the eigenvalue equation ΩFI = ωI2FI, where Ω is a matrix consisting of products of occupied-virtual Kohn–Sham orbitals and the eigenvalues ωI2 correspond to squared excitation energies while the oscillator strengths are extracted from the eigenvectors FI and employing the wB97X long range corrected hybrid exchange–correlation functional. Note that the TD-DFT technique has been widely used for computing the optical properties of a variety of nanostructures,46–55 to list a few. All absorption spectra are broadened by a Gaussian smearing of width σ = 0.15 eV.
Results and discussion
Before discussing the optical properties of pristine and pyridine adsorbed PAHs in detail, it is instructive first to analyze the optical absorption spectrum of a pyridine molecule. To this aim, we plot in Fig. 1 the optical absorption spectrum of a pyridine molecule in an energy range of 0 to 5.0 eV. One finds two different well-localized bands in the spectrum: one band (focused and dominant) located at 4.75 eV and one low-intensity band located at 4.20 eV.
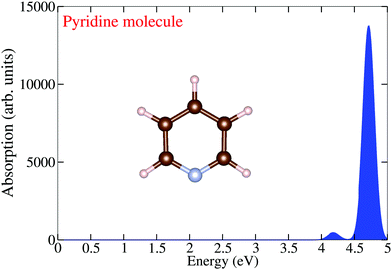 |
| Fig. 1 TDDFT calculated optical absorption spectrum of a pyridine molecule. | |
Let us now discuss the optical absorption spectra of pristine circular PAHs, see Fig. 2. With the help of the EDD map corresponding to the dominant band, we gain further insight into the nature of optical excitation. It should be stressed that the dominant band is composed of many single particle transitions. Let us begin our analysis starting from the smallest circular PAH considered in this work, i.e., the C1 structure (C24H12). Fig. 2 shows that the absorption spectrum of this structure consists of a band located at 4.90 eV and the corresponding EDD map exhibits plasmon-like resonance having similar characteristics to the molecular plasmons.31 When moving to the C2 structure (C54H18), one finds that the dominant band is red-shifted to 3 eV and three new low-intensity bands emerge right to the dominant band. The EDD map corresponding to the dominant band exhibits typical plasmon-like resonance. Interesting optical response modifications emerge starting from the C3 structure (C96H24). One finds two dominant bands in the absorption spectrum. The first band is located at 2.75 eV and the second band is located at 4.60 eV. As expected, the EDD map corresponding to the first dominant band shows typical plasmon-like resonance. Interesting changes appear in the case of the C4 structure (C150H30). One sees that the second dominant band is now more focused and both the bands are slightly red-shifted. In the case of the largest circular PAH considered in this work [C5 (C216H36)], we find two dominant bands having comparable absorption intensities. It is worth noting that with increasing size, both the dominant bands red-shift systematically. Now let us shed some light on the optical response modifications of circular PAHs upon pyridine adsorption, see Fig. 3. We placed the pyridine molecule of size 0.5 nm on top of PAHs. The prima facie analysis provides some interesting findings. In the case of pyridine@C1, pyridine@C2, pyridine@C3 and pyridine@C4, the key effect of pyridine adsorption is to transform the dominant band into multiple low-intensity bands distributed over a wider energy range. This could be due to the mixing of the PAH plasmon with the pyridine states. Interestingly, pyridine adsorption has only a marginal effect on the optical properties of the pyridine@C5 system since both the absorption spectrum and the EDD map look similar to those of the corresponding pristine C5 structure. More precisely, in the case of the large-sized PAHs, the plasmonic and pyridine excitation can be clearly identified and separated. It is useful to see how the optical gap (the energy difference between the ground state and the first excited state) of the circular PAHs change as a function of the PAH size. One easily concludes that the optical gap increases more or less monotonically with the reduction in size, see Table 1. This is understandable since by decreasing the PAH size, the density of states transforms from a continuum to a discrete set of levels, exhibiting the molecular character of the PAHs.
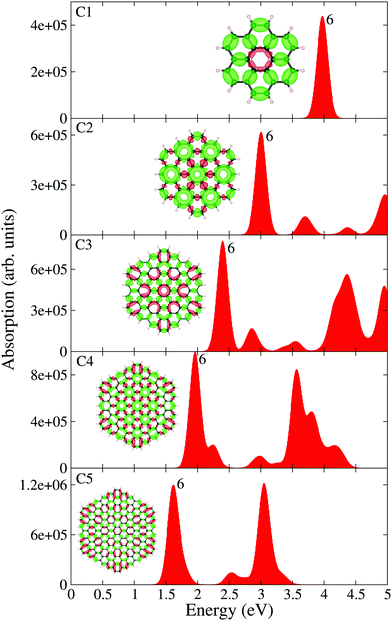 |
| Fig. 2 TDDFT calculated optical absorption spectra of circular PAHs as a function of their size. EDD map (with two different orientations for an easy visual inspection) and the root number corresponding to the dominant band are also shown in the figure. Red and green colors represent electron density accumulation (depletion) regions during the excitation, respectively. Both densities are plotted using the same iso-surface contour value. The values of the density of states are shown in the ESI,† see Fig. S1. | |
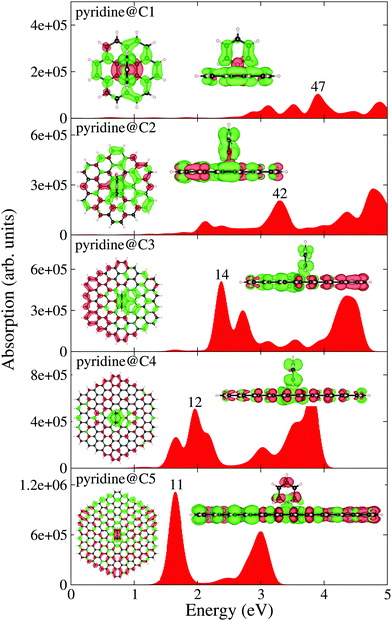 |
| Fig. 3 TDDFT calculated optical absorption spectra of pyridine adsorbed circular PAHs as a function of their size. EDD map (with two different orientations for an easy visual inspection) and the root number corresponding to the dominant band are also shown in the figure. Red and green colors represent electron density accumulation (depletion) regions during the excitation, respectively. Both densities are plotted using the same iso-surface contour value. The values of the density of states are shown in the ESI,† see Fig. S2. | |
Table 1 TD-DFT calculated optical gap of the circular and triangular PAHs
PAH |
Optical gap |
C1 (C24H12) |
3.97 |
C2 (C54H18) |
3.00 |
C3 (C96H24) |
2.40 |
C4 (C150H30) |
1.96 |
C5 (C216H36) |
1.62 |
T1 (C22H14) |
3.30 |
T2 (C78H24) |
2.78 |
T3 (C141H33) |
2.63 |
T4 (C222H42) |
2.49 |
T5 (C321H51) |
2.36 |
Having analyzed the optical properties of pristine and pyridine adsorbed circular PAHs, we next analyze the optical properties of triangular PAHs. This analysis is important to understand the influence of shape effects on the optical properties. Let us analyze the pristine triangular PAHs, see Fig. 4. We begin our discussion starting from the smallest triangular PAH, i.e., the T1 structure (C22H14). Fig. 4 shows that the absorption spectrum consists of a dominant band located at 3.50 eV and a couple of low-intensity bands located between 2 and 3 eV. Note that the EDD map corresponding to the dominant bands does not exhibit plasmon-like resonance. Interesting modifications in the absorption spectrum emerge in the case of the T2 structure (C78H24). Note that a new low-intensity band appears at 0.50 eV and the dominant band is red-shifted by 0.50 eV. Starting from the T3 structure (C141H33), one observes that the low-energy band splits into several bands and a few new bands emerge to the left of the dominant band. Further splitting of the low-energy band can be observed in the case of the T4 structure (C222H42). Interestingly, one finds that the dominant band starts to split further. Finally, in the case of the T5 structure (C321H51), one finds several new peaks emerging in the low-energy spectral region and the dominant band is now clearly split into two well-focused bands. EDD maps shown in Fig. 4 suggest that the electron excitation corresponding to the dominant band is not plasmonic. This is a vital result since it shows the importance of shape in exhibiting plasmons. In addition, the optical gap of triangular PAHs increases more or less monotonically with the reduction in PAH size, see Table 1. Now let us provide some insight into the triangular PAHs having pyridine molecules adsorbed on top, see Fig. 5. One readily notices that the effect of pyridine adsorption is to transform the dominant band into multiple low-intensity bands distributed in a wider energy range, similar to the circular PAHs shown in Fig. 3. Finally, it is also interesting to see how the molecular electrostatic potential energy (MEP) map changes due to pyridine adsorption. Some representative results are shown in Fig. 6.
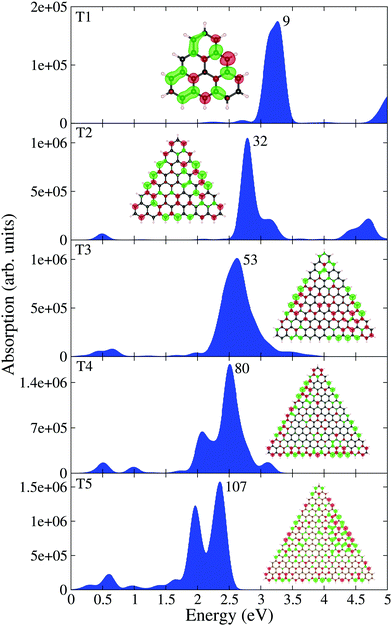 |
| Fig. 4 TDDFT calculated optical absorption spectra of triangular PAHs as a function of their size. EDD map (with two different orientations for an easy visual inspection) and the root number corresponding to the dominant band are also shown in the figure. Red and green colors represent electron density accumulation (depletion) regions during the excitation, respectively. Both densities are plotted using the same iso-surface contour value. The values of the density of states are shown in the ESI,† see Fig. S3. | |
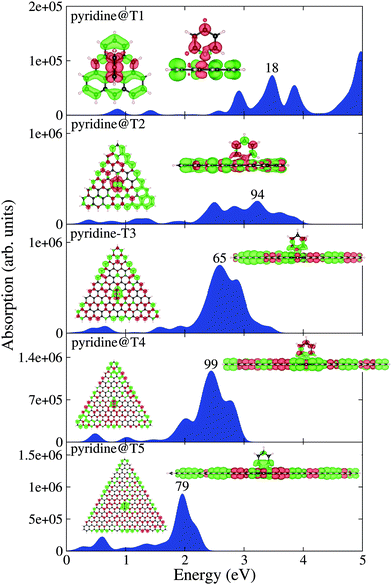 |
| Fig. 5 TDDFT calculated optical absorption spectra of pyridine adsorbed triangular PAHs as a function of their size. EDD map (with two different orientations for an easy visual inspection) and the root number corresponding to the dominant band are also shown in the figure. Red and green colors represent electron density accumulation (depletion) regions during the excitation, respectively. Both densities are plotted using the same iso-surface contour value. The values of the density of states are shown in the ESI,† see Fig. S4. | |
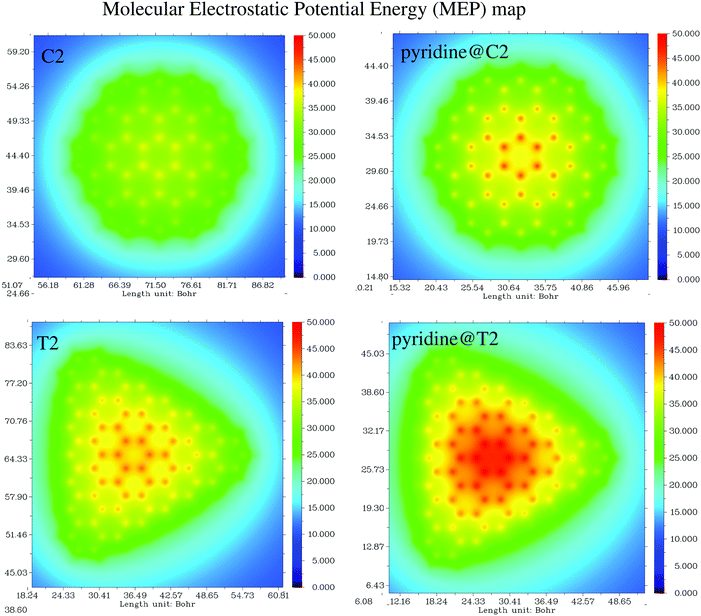 |
| Fig. 6 Molecular electrostatic potential map (MEP) of C2, pyridine@C2, T2, and pyridine@T2 structures. | |
Conclusion
Using time-dependent density-functional-theory calculations, we have demonstrated the optical properties of pristine and pyridine adsorbed circular and triangular PAHs. Our results reveal the complex nature of the optical properties with the help of optical absorption spectra and electron difference density maps. Our simulations allow us to draw the following conclusions: (1) pristine circular PAHs exhibit plasmon-like resonances; (2) pyridine adsorbed circular PAHs [C1, C2, C3 and C4] damp the plasmon resonance, whereas in the case of C5, the plasmonic and pyridine excitation can be separately identified; (3) pristine triangular PAHs do not exhibit plasmon-like resonances and the dominant band in the optical absorption spectra was found to be damped as a result of pyridine adsorption. We hope that our theoretical findings are pivotal for the emerging field of PAH based molecular sensing applications.
Conflicts of interest
There are no conflicts to declare.
Acknowledgements
The research reported in this publication was supported by funding from Kuwait College of Science and Technology (KCST).
References
- K. Li, M. I. Stockman and D. J. Bergman, Self-Similar Chain of Metal Nanospheres as an Efficient Nanolens, Phys. Rev. Lett., 2003, 91, 227402 CrossRef PubMed.
- R. Alvarez-Puebla, L. M. Liz-Marzán and F. J. García de Abajo, Light Concentration at the Nanometer Scale, J. Phys. Lett., 2010, 1, 2428–2434 CAS.
- A. Manjavacas, J. G. Liu, V. Kulkarni and P. Nordlander, Plasmon-Induced Hot Carriers in Metallic Nanoparticles, ACS Nano, 2014, 8, 7630–7638 CrossRef CAS PubMed , PMID: 24960573.
- B. Liedberg, C. Nylander and I. Lunström, Surface plasmon resonance for gas detection and biosensing, Sens. Actuators, 1983, 4, 299–304 CrossRef CAS.
- S. Zeng, D. Baillargeat, H.-P. Ho and K.-T. Yong, Nanomaterials enhanced surface plasmon resonance for biological and chemical sensing applications, Chem. Soc. Rev., 2014, 43, 3426–3452 RSC.
- M. Li, S. K. Cushing and N. Wu, Plasmon-enhanced optical sensors: a review, Analyst, 2015, 140, 386–406 RSC.
- I. Pastoriza-Santos, A. Sánchez-Iglesias, F. J. García de Abajo and L. M. Liz-Marzán, Environmental Optical Sensitivity of Gold Nanodecahedra, Adv. Funct. Mater., 2007, 17, 1443–1450 CrossRef CAS.
- C. Clavero, Plasmon-induced hot-electron generation at nanoparticle/metal-oxide interfaces for photovoltaic and photocatalytic devices, Nat. Photonics, 2014, 8, 95 CrossRef CAS.
- J. Y. Park, L. R. Baker and G. A. Somorjai, Role of Hot Electrons and Metal-Oxide Interfaces in Surface Chemistry and Catalytic Reactions, Chem. Rev., 2015, 115, 2781–2817 CrossRef CAS PubMed , PMID: 25791926.
- L. Zhou, C. Zhang, M. J. McClain, A. Manjavacas, C. M. Krauter, S. Tian, F. Berg, H. O. Everitt, E. A. Carter, P. Nordlander and N. J. Halas, Aluminum Nanocrystals as a Plasmonic Photocatalyst for Hydrogen Dissociation, Nano Lett., 2016, 16, 1478–1484 CrossRef CAS PubMed , PMID: 26799677.
- D. F. Swearer, H. Zhao, L. Zhou, C. Zhang, H. Robatjazi, J. M. P. Martirez, C. M. Krauter, S. Yazdi, M. J. McClain, E. Ringe, E. A. Carter, P. Nordlander and N. J. Halas, Heterometallic antenna-reactor complexes for photocatalysis, Proc. Natl. Acad. Sci. U. S. A., 2016, 113, 8916–8920 CrossRef CAS PubMed.
- M. Jablan, H. Buljan and M. Soljačić, Plasmonics in graphene at infrared frequencies, Phys. Rev. B: Condens. Matter Mater. Phys., 2009, 80, 245435 CrossRef.
- J. Chen, M. Badioli, P. Alonso-González, S. Thongrattanasiri, F. Huth, J. Osmond, M. Spasenovic, A. Centeno, A. Pesquera, P. Godignon, A. Zurutuza Elorza, N. Camara, F. J. G. de Abajo, R. Hillenbrand and F. H. L. Koppens, Optical nano-imaging of gate-tunable graphene plasmons, Nature, 2012, 487, 77 CrossRef CAS PubMed.
- H. Yan, T. Low, W. Zhu, Y. Wu, M. Freitag, X. Li, F. Guinea, P. Avouris and F. Xia, Damping pathways of mid-infrared plasmons in graphene nanostructures, Nat. Photonics, 2013, 7, 394 EP CrossRef.
- G. X. Ni, A. S. McLeod, Z. Sun, L. Wang, L. Xiong, K. W. Post, S. S. Sunku, B.-Y. Jiang, J. Hone, C. R. Dean, M. M. Fogler and D. N. Basov, Fundamental limits to graphene plasmonics, Nature, 2018, 557, 530–533 CrossRef CAS PubMed.
- Q. Guo, C. Li, B. Deng, S. Yuan, F. Guinea and F. Xia, Infrared Nanophotonics Based on Graphene Plasmonics, ACS Photonics, 2017, 4, 2989–2999 CrossRef CAS.
- M. B. Lundeberg, Y. Gao, R. Asgari, C. Tan, B. Van Duppen, M. Autore, P. Alonso-González, A. Woessner, K. Watanabe, T. Taniguchi, R. Hillenbrand, J. Hone, M. Polini and F. H. L. Koppens, Tuning quantum nonlocal effects in graphene plasmonics, Science, 2017, 357, 187–191 CrossRef CAS PubMed.
- D. Rodrigo, O. Limaj, D. Janner, D. Etezadi, F. J. Garca de Abajo, V. Pruneri and H. Altug, Mid-infrared plasmonic biosensing with graphene, Science, 2015, 349, 165–168 CrossRef CAS PubMed.
- Z. Fei,
et al., Infrared Nanoscopy of Dirac Plasmons at the Graphene-SiO2 Interface, Nano Lett., 2011, 11, 4701–4705 CrossRef CAS PubMed , PMID: 21972938.
- Z. Fang, S. Thongrattanasiri, A. Schlather, Z. Liu, L. Ma, Y. Wang, P. M. Ajayan, P. Nordlander, N. J. Halas and F. J. García de Abajo, Gated Tunability and Hybridization of Localized Plasmons in Nanostructured Graphene, ACS Nano, 2013, 7, 2388–2395 CrossRef CAS PubMed , PMID: 23390960.
- N. K. Emani, T.-F. Chung, X. Ni, A. V. Kildishev, Y. P. Chen and A. Boltasseva, Electrically Tunable Damping of Plasmonic Resonances with Graphene, Nano Lett., 2012, 12, 5202–5206 CrossRef CAS PubMed , PMID: 22950873.
- H. Yan, Z. Li, X. Li, W. Zhu, P. Avouris and F. Xia, Infrared Spectroscopy of Tunable Dirac Terahertz Magneto-Plasmons in Graphene, Nano Lett., 2012, 12, 3766–3771 CrossRef CAS PubMed , PMID: 22690695.
- N. Kumada, P. Roulleau, B. Roche, M. Hashisaka, H. Hibino, I. Petković and D. C. Glattli, Resonant Edge Magnetoplasmons and Their Decay in Graphene, Phys. Rev. Lett., 2014, 113, 266601 CrossRef CAS PubMed.
- Y. Fan, N.-H. Shen, F. Zhang, Q. Zhao, Z. Wei, P. Zhang, J. Dong, Q. Fu, H. Li and C. M. Soukoulis, Photoexcited Graphene Metasurfaces: Significantly Enhanced and Tunable Magnetic Resonances, ACS Photonics, 2018, 5, 1612–1618 CrossRef CAS.
- F. J. García de Abajo, Graphene Plasmonics: Challenges and Opportunities, ACS Photonics, 2014, 1, 135–152 CrossRef.
- G. X. Ni, L. Wang, M. D. Goldflam, M. Wagner, Z. Fei, A. S. McLeod, M. K. Liu, F. Keilmann, B. Özyilmaz, A. H. Castro Neto, J. Hone, M. M. Fogler and D. N. Basov, Ultrafast optical switching of infrared plasmon polaritons in high-mobility graphene, Nat. Photonics, 2016, 10, 244 CrossRef CAS.
- S. Song, J. Heo, T. K. Lee, S. Park, B. Walker, S. K. Kwak and J. Y. Kim, Optically Tunable Plasmonic Two-Dimensional Ag Quantum Dot Arrays for Optimal Light Absorption in Polymer Solar Cells, J. Phys. Chem. C, 2017, 121, 17569–17576 CrossRef CAS.
- J. Chen, M. Badioli, P. Alonso-González, S. Thongrattanasiri, F. Huth, J. Osmond, M. Spasenović, A. Centeno, A. Pesquera, P. Godignon, A. Z. Elorza, N. Camara, F. J. J. G. de Abajo, R. Hillenbrand and F. H. L. Koppens, Optical nano-imaging of gate-tunable graphene plasmons, Nature, 2012, 487, 77–81 CrossRef CAS PubMed.
- A. Woessner, M. B. Lundeberg, Y. Gao, A. Principi, P. Alonso-González, M. Carrega, K. Watanabe, T. Taniguchi, G. Vignale, M. Polini, J. Hone, R. Hillenbrand and F. H. L. Koppens, Highly confined low-loss plasmons in graphene-boron nitride heterostructures, Nat. Mater., 2014, 14, 421 CrossRef PubMed.
- A. Lauchner, A. E. Schlather, A. Manjavacas, Y. Cui, M. J. McClain, G. J. Stec, F. J. García de Abajo, P. Nordlander and N. J. Halas, Molecular Plasmonics, Nano Lett., 2015, 15, 6208–6214 CrossRef CAS PubMed , PMID: 26244925.
- A. Manjavacas, F. Marchesin, S. Thongrattanasiri, P. Koval, P. Nordlander, D. Sánchez-Portal and F. J. García de Abajo, Tunable Molecular Plasmons in Polycyclic Aromatic Hydrocarbons, ACS Nano, 2013, 7, 3635–3643 CrossRef CAS PubMed , PMID: 23484678.
- H. Richter, T. G. Benish, O. A. Mazyar, W. H. Green and J. B. Howard, Formation of polycyclic aromatic hydrocarbons and their radicals in a nearly sooting premixed benzene flame, Proc. Combust. Inst., 2000, 28, 2609–2618 CrossRef CAS.
- S. Bhattacharya, K. Ghosh, S. Banerjee and M. Banerjee, Energies of charge transfer and formation equilibria of the complexes of [70]fullerene with some interesting polyaromatic molecules, Spectrochim. Acta, Part A, 2006, 64, 47–53 CrossRef PubMed.
- S. Bhattacharya, S. K. Nayak, S. Chattopadhyay, M. Banerjee and A. K. Mukherjee, Study of ground state EDA complex formation between [70]fullerene and a series of polynuclear aromatic hydrocarbons, Spectrochim. Acta, Part A, 2002, 58, 289–298 CrossRef.
- S. Bhattacharya, S. K. Nayak, S. Chattopadhyay, M. Banerjee and A. K. Mukherjee, Study of Molecular Complex Formation between [60]Fullerene and Two Series of Donors by the NMR Method, J. Phys. Chem. A, 2001, 105, 9865–9868 CrossRef CAS.
- A. Ray, K. Santhosh and S. Bhattacharya, Absorption spectrophotometric, fluorescence, transient absorption and quantum chemical investigations on fullerene/phthalocyanine supramolecular complexes, Spectrochim. Acta, Part A, 2011, 78, 1364–1375 CrossRef PubMed.
- A. J. Wilson and K. A. Willets, Molecular Plasmonics, Annu. Rev. Anal. Chem., 2016, 9, 27–43 CrossRef PubMed , PMID: 27049633.
- E. B. Guidez and C. M. Aikens, Origin and TDDFT Benchmarking of the Plasmon Resonance in Acenes, J. Phys. Chem. C, 2013, 117, 21466–21475 CrossRef CAS.
- T. Martin, M. Stefan, D.-G. Tanja, R. Carsten, D. Volker and M. Philipp, Distinguishing chemical and electromagnetic enhancement in surface-enhanced Raman spectra: The case of para-nitrothiophenol, J. Raman Spectrosc., 2013, 44, 1497–1505 CrossRef.
- S. K. Saikin, Y. Chu, D. Rappoport, K. B. Crozier and A. Aspuru-Guzik, Separation of Electromagnetic and Chemical Contributions to Surface-Enhanced Raman Spectra on Nanoengineered Plasmonic Substrates, J. Phys. Lett., 2010, 1, 2740–2746 CAS.
- M. M. Dvoynenko and J.-K. Wang, Finding electromagnetic and chemical enhancement factors of surface-enhanced Raman scattering, Opt. Lett., 2007, 32, 3552–3554 CrossRef CAS PubMed.
- D. P. Fromm, A. Sundaramurthy, A. Kinkhabwala, P. J. Schuck, G. S. Kino and W. E. Moerner, Exploring the chemical enhancement for surface-enhanced Raman scattering with Au bowtie nanoantennas, J. Chem. Phys., 2006, 124, 061101 CrossRef PubMed.
- M. Kerker, D.-S. Wang and H. Chew, Surface enhanced Raman scattering (SERS) by molecules adsorbed at spherical particles, Appl. Opt., 1980, 19, 3373–3388 CrossRef CAS PubMed.
- A. Marini, I. Silveiro and F. J. Garca de Abajo, Molecular Sensing with Tunable Graphene Plasmons, ACS Photonics, 2015, 2, 876–882 CrossRef CAS.
- F. Neese, Software update: the ORCA program system, version 4.0, Wiley Interdiscip. Rev.: Comput. Mol. Sci., 2018, 8, e1327 Search PubMed.
- N. Durante, A. Fortunelli, M. Broyer and M. Stener, Optical Properties of Au Nanoclusters from TD-DFT Calculations, J. Phys. Chem. C, 2011, 115, 6277–6282 CrossRef CAS.
- M. Kuisma, A. Sakko, T. P. Rossi, A. H. Larsen, J. Enkovaara, L. Lehtovaara and T. T. Rantala, Localized surface plasmon resonance in silver nanoparticles: Atomistic first-principles time-dependent density-functional theory calculations, Phys. Rev. B: Condens. Matter Mater. Phys., 2015, 91, 115431 CrossRef.
- P. Zhang, J. Feist, A. Rubio, P. García-González and F. J. García-Vidal, Ab initio nanoplasmonics: The impact of atomic structure, Phys. Rev. B: Condens. Matter Mater. Phys., 2014, 90, 161407 CrossRef.
- G.-T. Bae and C. M. Aikens, TDDFT and CIS Studies of Optical Properties of Dimers of Silver Tetrahedra, J. Phys. Chem. A, 2012, 116, 8260–8269 CrossRef CAS PubMed , PMID: 22838848.
- T. V. Teperik, P. Nordlander, J. Aizpurua and A. G. Borisov, Quantum effects and nonlocality in strongly coupled plasmonic nanowire dimers, Opt. Express, 2013, 21, 27306–27325 CrossRef PubMed.
- J. H. Mokkath and J. Henzie, One-dimensional aluminum nanoparticle chains: the influence of interparticle spacing and chain length on plasmon coupling behavior, J. Mater. Chem. C, 2017, 5, 4379–4383 RSC.
- J. H. Mokkath, Shapes matter: examining the optical response evolution in stretched aluminium nanoparticles via time-dependent density functional theory, Phys. Chem. Chem. Phys., 2018, 20, 51–55 RSC.
- A. Castro, M. A. L. Marques, J. A. Alonso and A. Rubio, Optical Properties of Nanostructures from Time-Dependent Density Functional Theory, J. Comput. Theor. Nanosci., 2004, 1, 230–253 CrossRef.
- F. De Angelis and L. Armelao, Optical properties of ZnO nanostructures: a hybrid DFT/TDDFT investigation, Phys. Chem. Chem. Phys., 2011, 13, 467–475 RSC.
- Y.-L. Wang and G.-S. Wu, Improving the TDDFT calculation of low-lying excited states for polycyclic aromatic hydrocarbons using the Tamm-Dancoff approximation, Int. J. Quantum Chem., 2008, 108, 430–439 CrossRef CAS.
Footnote |
† Electronic supplementary information (ESI) available: Density of states of PAHs and pyridine adsorbed PAHs. See DOI: 10.1039/c8cp06744a |
|
This journal is © the Owner Societies 2019 |
Click here to see how this site uses Cookies. View our privacy policy here.