DOI:
10.1039/C8AN01637B
(Paper)
Analyst, 2019,
144, 172-179
Solution-processed wrinkled electrodes enable the development of stretchable electrochemical biosensors
Received
23rd August 2018
, Accepted 19th October 2018
First published on 22nd October 2018
Abstract
Wearable biosensors are critical for enabling real-time and continuous health monitoring and disease management. Conductors that retain their conductivity under strain are an essential building block of these systems. Strategies based on stretchable materials or structures have enabled the development of electrodes that can withstand impressive strains before loss of conductivity. In spite of this, it remains challenging to develop three-dimensional and high surface area electrodes that combine stretchability with high analytical sensitivity. Here, we develop stretchable electrochemical biosensors using solution-processed wrinkled gold electrodes. Wrinkling enhances the surface area of the electrodes and allows glucose to be detected with a sensitivity of 750–810 μA M−1 cm−2. Furthermore, wrinkling enables electrodes to be strained by up to 230% without significant loss in conductivity.
Introduction
Wearable biosensors are envisioned to improve human health by enabling continuous disease management and real-time health monitoring.1,2 Stretchable conductors are a key building block in constructing the transducing elements3–5 or the interconnects6–8 of biosensing systems. Specially-designed materials or structures9,10 are used to create electrodes that preserve their conductivity upon stretching. Stretchable materials are created by combining elastomers with networks of conducting building blocks such as silver nanoparticles11 or nanowires,12–14 graphene,15 or carbon nanotubes.16 These materials generally have moderate conductivity under strain as the contact between their conductive building blocks diminishes.9 Stretchable structures are created based on geometries such as wrinkles,17 coils,18 sponges,19 and meshes,20,21 which allow the conductor to unfold or deform under strain. These structures enable high conductivity materials to be incorporated in a stretchable framework and maintain conductivity by minimizing breaks.
Wrinkled films are created when a compliant substrate modified with a stiff skin experiences compressive stress or the removal of tensile stress. The wrinkled geometry allows the applied strain to be relieved through unfolding and alignment of the wrinkles. As a result, stretchable electrodes, withstanding strains of up to 400% are created using conductive wrinkles.7,22,23 Additionally, the micro/nanoscale features of the wrinkled films significantly increase the electrochemical signal24 and analytical sensitivity25,26 of chemical and biological sensors.
The combination of enhanced analytical performance and stretchability makes wrinkled electrodes ideally suited for use in wearable sensors. Our goal was to develop stretchable biosensing electrodes with a wrinkled architecture using a facile, yet scalable, fabrication method. Solution-processed electrodes, where the precursors or building blocks of the conductive film are dispersed in a solvent removed during processing, offer great promise for this purpose. Solution-processing eliminates the need for vacuum-based equipment operated in cleanrooms and it is also applicable to large-area and high-volume manufacturing using roll-to-roll processes.27
Results and discussion
Fabrication of stretchable wrinkled electrodes using solution-processing
To create wrinkled electrodes on stretchable substrates, we fabricated solution-processed wrinkled electrodes26,28 on the surface of a commercially-available pre-strained polystyrene substrate and transferred them to stretchable elastomers.7,23 Pre-strained polystyrene shrinks when it is heated above its glass transition temperature.29 When the surface of pre-strained polystyrene is modified with a stiff skin, the compressive stress imposed on the skin layer causes it to crumple, leading to the creation of wrinkled films.30 To create wrinkled electrodes on stretchable substrates, we used the process schematically demonstrated in Fig. 1(a). We defined the electrode pattern using a vinyl mask adhered onto a pre-strained polystyrene substrate modified with an aminosilane linker. We then deposited a gold nanoparticle (∼12 nm diameter) seed layer on the polystyrene surface by leveraging on its electrostatic interactions with the linker layer. We created a continuous gold film on the seed layer using electroless deposition in a gold chloride solution. For 20 minutes of electroless deposition, the thickness of the gold film is estimated to be 55 nm.28 Following heat shrinking, a wrinkled gold film was created on the shrunk polystyrene substrate (Fig. 1(b)); however, this substrate was rigid and not applicable to stretchable biosensors. To transfer the electrodes to a stretchable substrate, we poured Ecoflex, a silicone elastomer, onto the wrinkled electrodes, and dissolved the polystyrene substrate after the Ecoflex had cured.7 This transferred the wrinkled electrodes from rigid polystyrene to stretchable Ecoflex.
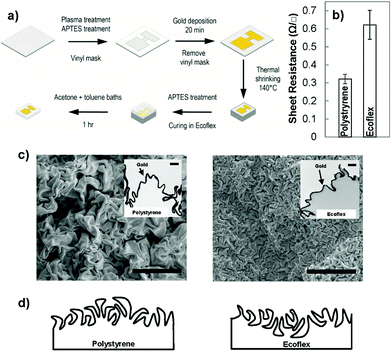 |
| Fig. 1 Fabrication of solution-processed electrodes on stretchable substrates. (a) Schematic of fabrication process for wrinkled electrodes on Ecoflex. The pre-strained polystyrene substrate was activated with plasma to incorporate –OH groups on its surface for coupling to (3-aminopropyl)triethoxysilane (APTES). A self-adhesive vinyl mask was placed on the substrate and patterned using a craft cutter. A gold nanoparticle seed layer followed by an electroless layer of gold were deposited on the masked substrate. After mask removal, the substrate was heat shrunk to create a wrinkled gold layer. The electrode was transferred to the Ecoflex substrate by casting and dissolution of the polystyrene substrate. (b) Sheet resistances of wrinkled gold film on polystyrene and Ecoflex. Error bars represent one standard deviation. (c) Scanning electron microscope images of wrinkled film before (left) and after (right) transfer from polystyrene to Ecoflex (scale bars are 10 μm). Insets show the transmission electron microscope images of the cross-section of the wrinkled film (scale bars are 1 μm). (d) Illustration of the morphology of wrinkles before and after transfer. | |
We compared the sheet resistance of the electroless deposited gold on polystyrene to the transferred gold on Ecoflex (Fig. 1(b)). The sheet resistance of gold on polystyrene is 0.33 ± 0.03 Ω □−1, and is comparable to a previously reported value,31 whereas the transferred wrinkled gold has a higher value. This higher resistance in the transferred film is expected since the transfer process introduces breaks into the previously continuous film due to the swelling of the Ecoflex during solvent processes.7,23 The value obtained here is lower than the previously reported resistance for stretchable wrinkled gold electrodes (85 ± 3 Ω □−1).7 This previous result is obtained using a 25 nm gold film compared to our ∼55 nm gold film. This difference is in line with previous results indicating that increasing the thickness of wrinkled gold films decreases their sheet resistance.25
To understand the structural differences between the wrinkled films created on polystyrene and those transferred to Ecoflex, we performed top-view and cross-sectional imaging using electron microscopy. The top-view scanning electron microscope (SEM) images showed that wrinkles on the surface of Ecoflex are significantly smaller in wavelength than those on polystyrene (Fig. 1(c)). To further examine this structural difference, we performed cross-sectional transmission electron microscopy (TEM) on the wrinkles positioned on Ecoflex and polystyrene (Fig. 1(c) – inset). We observed that the initial wrinkled gold film is rooted in the polystyrene substrate, interfaced with polystyrene on the bottom side and with air on the top side. When the wrinkled film is transferred from polystyrene to Ecoflex, the side of the wrinkles interfaced with polystyrene becomes the top-most surface. We hypothesize that when the gold film is compressed on the polystyrene substrate, it is easier for the crests to overlap one another forming folds while the troughs, embedded in polystyrene, rarely overlap. As a result, the surface of the wrinkled film on polystyrene is dominated by large folds, whereas, the surface of the wrinkled film on Ecoflex is dominated by small troughs, leading to the significant structural difference observed in these two materials architectures. A schematic drawing demonstrating the structure of the wrinkles before transfer (on polystyrene) and after transfer (on Ecoflex) is demonstrated in Fig. 1(d).
Enzyme-free glucose sensing and electrode sensitivity
Following the successful fabrication of solution-processed wrinkled gold electrodes on stretchable substrates, we investigated the suitability of these electrodes for electrochemical sensing. We analyzed their electrochemical surface area using cyclic voltammetry (CV) and their ability to oxidize glucose using chronoamperometry. Lastly, we compared their performance with the wrinkled gold electrodes fabricated on polystyrene (Fig. 2). The CV of the gold electrodes recorded in an acidic solution demonstrated the expected redox signature for gold (Fig. 2(a)). For wrinkled electrodes before and after transfer to the stretchable substrate, oxidation of gold occurred between 1.0 and 1.5 V in the forward scan. In the reverse scan, the characteristic reduction peak of gold oxide occurred at approximately 0.8 V. These results indicate that the transfer process allows the electrical and electrochemical properties of gold to be preserved.
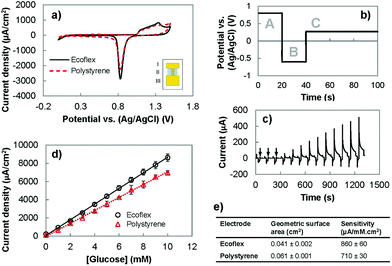 |
| Fig. 2 Electrochemistry using solution-processed wrinkled electrodes on stretchable substrates. (a) Cyclic voltammogram of polystyrene (dash) and Ecoflex electrodes (solid) in 0.05 M H2SO4 at a scan rate of 0.05 V s−1versus a Ag/AgCl reference electrode. The electrode is illustrated in inset: (I) contact pad, (II) insulated gold wire, and (III) working electrode surface. (b) A multi-step potential waveform was applied to the working electrode for detecting glucose. The portion marked A indicates the first step of the waveform (anodic cleaning), the portion marked B indicates the second step of the waveform (cathodic regeneration), and the portion C indicates the third step (analyte detection). (c) Pulsed amperometric signal of the wrinkled gold electrode. During the first three pulses (indicated by arrows), 1 mM KCl was added to ensure signal stability. Each signal thereafter was generated by adding 1 mM glucose. (d) Calibration curve plotting current density as a function of glucose concentration for polystyrene (triangle) and Ecoflex (circle) electrodes. The current was extracted from the first 5 seconds of the C pulse in the pulsed amperometric signal. All error bars represent one standard deviation. (e) Table summarizing the analytical sensitivity and geometric surface area for each class of electrode. | |
We investigated whether it would be possible to use the wrinkled electrodes created here for developing biosensors. We used pulsed amperometric detection (PAD) to monitor the concentration of glucose in the clinically-relevant range of 1–10 mM.32,33 PAD, compared to chronoamperometry, is more suitable for use in wearable biosensing systems because it offers built-in surface cleaning,34,35 which improves reproducibility and extends the linear range of biosensors.26 This method uses a multistep potential waveform (Fig. 2(b) and (c)) for anodic cleaning (0.80 V), cathodic regeneration (−0.60 V), and detection of glucose (0.27 V).26,34,36 The application of a large positive potential removes adsorbed carbohydrates on the gold surface, and causes the formation of a stable oxide layer (AuO) which is removed using a negative potential to restore the electrode surface and allow for continuous testing.33 The current obtained following the detection pulse was averaged, divided by the geometric surface area and plotted as a function of glucose concentration (Fig. 2(d)). We compared the performance of the polystyrene and Ecoflex electrodes for enzyme-free oxidation of glucose by comparing their sensitivities. It was found that the polystyrene and Ecoflex electrodes had sensitivities of 710 and 860 μA mM−1 cm−2 respectively (Fig. 2(e)). The solvent steps used for dissolving polystyrene swell and shrink the Ecoflex layer,7,23 which decrease the two-dimensional footprint of the gold electrode. The reduction in geometric surface area after transfer results in a higher ratio of the electroactive to the geometric surface area of the Ecoflex electrodes. Since the glucose oxidation current is related to the electroactive surface area, the increase in this ratio results in a higher current density and sensitivity for glucose detection using the Ecoflex electrodes. The sensitivity of the Ecoflex electrodes is slightly (∼8%) larger than the wrinkled and nanoporous gold electrodes previously reported for enzyme-free glucose biosensors made from gold.26 This demonstrates that fabricating wrinkled gold electrodes by solution-processing and materials transfer leads to stretchable biosensors with excellent analytical performance.
Electrochemistry under strain
To assess the performance of the stretchable electrodes, we sought to measure their electrochemical current under strain. For this purpose, we created an on-chip electrochemical cell, where the working, counter, and reference electrodes were integrated on a single substrate.31 Using this setup, the electrochemical response of the electrodes was measured, directly in the electrolyte, while strain was applied to the substrate (Fig. 3(a)). The performance of the on-chip electrochemical cell was first evaluated by performing CV in 0.05 M sulfuric acid (Fig. 3(b)) and in 10 mM of glucose in a basic solution (Fig. 3(c)). The CV in sulfuric acid shows the expected redox signature of gold in acidic solutions with a broad peak related to the oxidation of gold and a sharp peak indicating the reduction of gold oxide. However, the peak potentials were shifted compared to the response observed when a conventional off-chip reference electrode was used (Fig. 2(a)). This is expected as the electrochemical potential for the gold pseudo-reference electrode is different from the potential of the conventional Ag/AgCl electrode.37 We also performed CV scans in a basic solution containing glucose using the on-chip electrochemistry cell and single electrode polystyrene and Ecoflex electrodes. For single electrodes, the forward CV scan showed an oxidation peak from ∼−0.2 V to ∼0.4 V and the reverse scan showed a peak at ∼0.1 V, which is in line with previous studies.26 In agreement with the acidic solution scans (Fig. 2(a)), the current density achieved by the Ecoflex electrode is higher than the polystyrene electrode. With the change from the external Ag/AgCl reference electrode to the on-chip gold pseudo-reference, there is a positive peak potential shift of ∼0.1 V. Due to this potential shift, we evaluated the ability of the on-chip electrochemical cell in detecting glucose by modifying the detection potential in the PAD method from 0.27 used with the single electrode chips to 0.4 V.
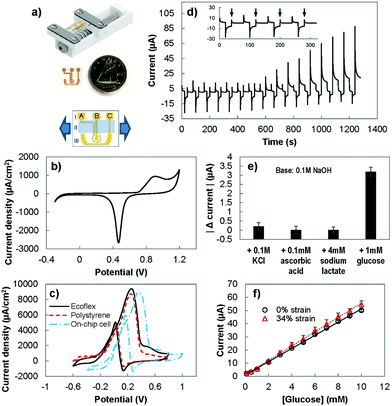 |
| Fig. 3 Electrochemistry using on-chip electrochemical cells on stretchable substrates. (a) Illustration of the setup used for measuring electrochemical response under strain (strain direction indicated by blue arrows). Picture and illustration of an on-chip electrochemical cell: (I) contact pad, (II) insulated gold wire, and (III) electrode surfaces; (A) reference electrode, (B) working electrode, and (c) counter electrode. (b) Cyclic voltammogram obtained using the on-chip electrochemical cell in 0.05 M H2SO4 at a scan rate of 0.05 V s−1versus Ag/AgCl. (c) Cyclic voltammogram obtained for gold on polystyrene versus Ag/AgCl (dash), gold on Ecoflex versus Ag/AgCl (solid), and gold on an Ecoflex electrochemical cell versus a gold reference electrode (dash-dot) in a solution of 0.1 M NaOH and 10 mM glucose at a scan rate of 0.1 V s−1. (d) Pulsed amperometric signal of the on-chip cell with sequential addition of 0.1 M NaOH, 0.1 M KCl, 0.1 mM ascorbic acid, 4 mM sodium lactate, and different concentrations of glucose (0.1 mM, 0.5 mM, and increments of 1 mM from 1 mM until 10 mM). Inset magnifies the first 4 detection steps with arrows corresponding to adding 0.1 M NaOH, 0.1 M KCl, 0.1 mM ascorbic acid, and 4 mM sodium lactate respectively. (e) Change in current before and after sequential additions of 0.1 M KCl, 0.1 mM ascorbic acid, 4 mM sodium lactate and 1 mM glucose to 0.1 M NaOH. (f) Calibration curve demonstrating current as a function of glucose concentration for on-chip electrochemical cells prior to (circle) and after stretching (triangle). All error bars represent one standard deviation. | |
We used this modified PAD method to assess the sensitivity and specificity of the system. We studied the response of the on-chip electrochemical cell to clinically-relevant concentrations38 of interfering species: ascorbic acid (0.1 mM), lactate (4 mM) and potassium chloride (0.1 M) (Fig. 3(d)). The response to the interfering species (Fig. 3(e)) was at the order of ten times smaller than the effect seen with 1 mM of glucose. The sensitivity of the on-chip electrochemical cell in response to glucose is 750 ± 80 μA mM−1 cm−2, which is comparable to the values obtained with polystyrene and Ecoflex electrodes. This indicates that using on-chip reference and counter electrodes does not significantly affect the observed electrochemical behaviour.
To evaluate the ability of the sensor to operate under strain, we measured the sensitivity of the electrochemical cell while under strain (Fig. 3(f)). The sensitivity was measured to be 810 ± 80 μA mM−1 cm−2, which did not change significantly from the unstrained value. The limit-of-detection of the sensor was determined by using the data set with baseline of 0.1 M NaOH, 0.1 M KCl, 0.1 mM ascorbic acid and 4 mM sodium lactate, and the addition of various concentrations of glucose (0.1 mM, 0.5 mM, and increments of 1 mM from 1 mM until 10 mM). Using the H–V method (detailed in the Experimental section),39 the limit of detection for glucose, with and without stretching, was estimated to be 0.9 mM (α = 0.05).
Resistance under strain
To better understand the behaviour of the electrodes under strain, we investigated the sheet resistance and micro/nanostructure of the electrodes under strain. The resistance was measured using two different setups (Fig. 4(a)). In the first setup, we placed the current measuring probes such that the current path was perpendicular to the direction of stretching. This measurement setup reflected the strain-current direction during the electrochemistry scans. In the second setup, the current path was designed to be parallel to the direction of stretching. In the perpendicular setup, the gold film was able to be strained up to 230% before failure and showed a consistently decreasing resistance as the strain was increased. In the parallel setup, the electrodes showed a relatively stable resistance until approximately 30% strain was applied. After this threshold, the resistance increased until the electrodes were no longer conducting at a strain of 100%. The correlation between resistance and electrochemical current can be used as a method for calibrating the changes in sensing current that occur under strain.
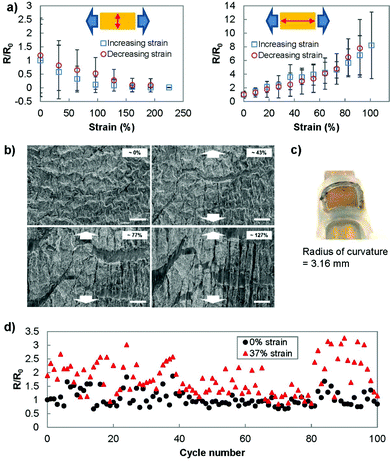 |
| Fig. 4 Resistance study for the transferred wrinkled gold film. (a) Relative resistance of wrinkled gold film on Ecoflex strained to failure and released. Direction of current measured is indicated by double sided arrow (red) and strain direction is indicated by the two larger arrows (blue). (b) SEM images of surface of gold film on Ecoflex under different strain. Direction of strain is indicated by the white arrows. Scale bar is 100 μm. (c) Picture of the wrinkled gold film on Ecoflex wrapped on a tube with a radius of 3.16 mm. (d) Wrinkled gold film on Ecoflex stretched to 37% strain and released for 100 cycles. All error bars represent one standard deviation. | |
The significant difference in electrical characteristics when current flows perpendicular or parallel to the direction of strain was previously reported. Applying strain causes cracks to appear in the direction perpendicular to the strain,40 which was also observed in SEM images obtained under strain (Fig. 4(b)). In the case where the strain and current path are parallel to each other, the propagation of cracks perpendicular to the current path increases the current path length and the resultant resistance.41 This is not the case when the current path is parallel to the direction of the cracks. Additionally, when the substrate is strained, the wrinkles come into contact in the direction perpendicular to the strain (also observed in Fig. 4(b)) and decrease the measured resistance in this direction.42
To better replicate the strain involved at the surface of human skin, we wrapped the transferred gold film (7 by 7 mm) onto a tube with radius of curvature of 3.16 mm (Fig. 4(c)). The measured resistance (current parallel to stretching) showed no significant deviation from the original resistance (R/R0 = 1.01 ± 0.15). In addition, we examined the ability of the transferred wrinkled gold film to recover to its pre-stretched state by stretching and releasing the Ecoflex electrode for 100 cycles (Fig. 4(d)). Throughout this cycling test, the sensor was able to recover its conductivity after the applied strain was removed.
This study demonstrates that the stretchable biosensors developed here work reliably up to a strain of 230% or 100% when the sensor is strained perpendicular or parallel to the current path respectively. This indicates that the design of electrode configuration, in addition to electrode material, is of paramount importance when creating stretchable biosensing platforms.
Conclusion
We demonstrated the fabrication of stretchable wrinkled electrodes for applying electrochemical readout to wearable biosensing systems. Previous work showed that wrinkled electrodes enhance the performance of biosensors by enhancing their analytical sensitivity26 and increasing their detectible electrochemical current.24 Here, we demonstrate that it is possible to create stretchable wrinkled electrochemical biosensors using a facile fabrication method based on solution-processing. It was found that transferring the wrinkled electrodes from rigid substrates to stretchable elastomers does not significantly alter their electrochemical behaviour and analytical sensitivity. The stretchable biosensors created here showed an analytical sensitivity of 750–810 μA M−1 cm−2 for detecting glucose, which is amongst the highest reported using gold electrodes.26,43 It is found that the sensor shows dramatically improved strain resilience when it is stretched in a direction perpendicular to the current path compared to straining parallel to the current direction. Finally, these highly sensitive wrinkled biosensors can be strained by 230% in the direction perpendicular to the current path without significant loss in conductivity.
Experimental section
Reagents
Potassium chloride (KCl, ≥99.0%), sodium L-lactate (∼98%), (3-aminopropyl)triethoxysilane (99%), gold(III) chloride solution (99%) and toluene (≥99.5%) were purchased from Sigma-Aldrich (Oakville, Ontario). Ethanol (anhydrous) was purchased from Commercial Alchohols (Brampton, Ontario). Hydrogen peroxide (30%) was purchased from Fisher Scientific. Ascorbic acid (C6H8O6, >99%), sulfuric acid (H2SO4, 98%), dextrose (CH2OH(CHOH)4CHO, >99%) and acetone were purchased from Caledon (Georgetown, Ontario). Sodium hydroxide (NaOH, 1.0 M) was purchased from LabChem (Zelienople, PA). All reagents were of analytical grade. Milli-Q grade water (18.2 MΩ) was used to prepare all solutions.
Polystyrene substrates preparation
Polystyrene substrates were prepared as described in a previous paper26 with the silanization duration reduced to 2 hours. Vinyl was cut into the designed shape using the Robo Pro CE5000-40-CRP cutter (Graphtec America Inc., Irvine, CA). The polystyrene substrates were covered with a vinyl mask, fixed on petri dishes using double sided tape and submerged in a solution of citrate-stabilized gold nanoparticles (∼12 nm diameter) overnight. The gold nanoparticles were synthesized as described previously (preparation 1).44 The polystyrene substrates were rinsed with water and dried. Electroless deposition was done by submerging polystyrene substrates in 0.1% HAuCl4 with 1
:
20 ratio of H2O2 to water26 for 20 minutes. After removing vinyl masks, the polystyrene substrates were placed in an oven (ED56 Binder, Tuttlingen, Germany) at 140 °C for 5 minutes.
Electrodes transfer
The shrunken polystyrene substrates were submerged in aminosilane bath (10% (3-aminopropyl)triethoxysilane) for 2 hours. The aminosilane improved the bond between the Ecoflex and wrinkled gold surface as it works as a molecular adhesive.45 Afterwards, the polystyrene substrates were rinsed and dried. Part A and part B of the Ecoflex (Ecoflex 0030, Sooth-On, PA) were mixed in a 1
:
1 ratio as outlined by the manufacturer. The mixture was poured over the polystyrene substrates and placed in a desiccator for 10 minutes. After 4 hours of curing, the Ecoflex enveloped polystyrene was placed in the oven at 80 °C for 2 hours, followed by 100 °C for another hour. The Ecoflex on the side of the electrodes was sliced off such that the polystyrene was exposed. The electrodes were placed in an acetone bath at 75 °C for approximately 30 minutes. The electrodes were then rinsed and placed into a toluene bath at 65 °C for approximately 15 minutes until all the polystyrene was dissolved. The electrodes were then placed on a glass slide to air dry.
Glucose sensing
The sensitivity experiments were performed using chronoamperometry on a Reference 600 potentiostat (Gamry Instruments, Warminister, PA, USA). For polystyrene and Ecoflex electrodes, glucose concentrations from 1 to 10 mM were measured in a solution containing 0.1 M NaOH and 0.1 M KCl. Using pulsed amperometric detection, 0.8 V was applied to the electrode for 20 seconds for anodic cleaning, −0.6 V was applied for 20 s for cathodic regeneration, and 0.27 V for 60 seconds for detection. Glucose was added in increments of 1 mM and mixed at 200 rpm on a shaker. The shaker was stopped during each detection step. The measured current over the first 5 seconds of the detection step was averaged, standardized by the electrode geometric surface area, and plotted as a function of the glucose concentration.
The PAD steps for on-chip electrochemical cells were modified to 1 V for anodic cleaning, −0.4 V for cathodic regeneration, and 0.4 V (40 s) for detection. A 60 μL solution with the mixture of analytes to be tested was placed on the electrodes. CV was run from −0.4 to 1 V with a scan rate of 0.1 V s−1 until the signal stabilized. PAD was then applied to the electrodes. All solutions were used within 24 hours of mixing. The sensitivity of the electrodes was determined by measuring the slope of the current versus concentration curve and dividing the slope by the geometric surface area.
The limit-of-detection (LOD) was approximated by39
LOD = 2t(1−α, n−2)[sy/x/b][1 + (1/n)]1/2 |
where
t is the one-sided (1 −
α)100% of the student's
t-distribution,
sy/x is the regression residual standard deviation,
b is the slope of the current
versus concentration curve and
n is the number of samples used for the data.
Sheet resistance measurement
Sheet resistance was measured using the conventional four-point probe method with a sourcemeter (Keithley 2410). The sheet resistance of the material under strain was measured using the van der Pauw method with a square four-point probe configuration. Sheet resistance was calculated by assuming the sample to be a semi-infinite 2D sheet.46 The electrodes were strained by 32 or 9% increments, and the resistance was measured at each increment until it become unstable. Afterwards, the resistance was measured while releasing the strain by 32 or 9% decrements until the initial state.
Surface characterisation
SEM imaging was performed on a JEOL 7000F microscope. TEM imaging was performed on a JEOL 2010F microscope after the samples were embedded in a resin and sectioned by microtoming.
Conflicts of interest
There are no conflicts to declare.
Acknowledgements
The financial support for this work was provided by the Natural Sciences and Engineering Council of Canada (NSERC) and Ontario Ministry of Research and Innovation. L. S. is the Canada Research Chair in Miniaturized Biomedical Devices. The microscopy work was carried out at the Canadian Centre for Electron Microscopy, a facility supported by the Canada Foundation for Innovation under the Major Science Initiative program, NSERC, and McMaster University.
We would like to thank Jie Yang for her help with transmission electron microscopy (TEM) imaging and Marcia Reid for her help in cross-sectional TEM sample preparation.
References
- D.-H. Kim, R. Ghaffari, N. Lu and J. A. Rogers, Flexible and Stretchable Electronics for Biointegrated Devices, Annu. Rev. Biomed. Eng., 2012, 14(1), 113–128 CrossRef CAS PubMed.
- S. Patel, H. Park, P. Bonato, L. Chan and M. Rodgers, A review of wearable sensors and systems with application in rehabilitation, J. Neuroeng. Rehabil., 2012, 9(1), 21 CrossRef PubMed.
- T. Yamada, Y. Hayamizu, Y. Yamamoto, Y. Yomogida, A. Izadi-Najafabadi and D. N. Futaba,
et al., A stretchable carbon nanotube strain sensor for human-motion detection, Nat. Nanotechnol., 2011, 6(5), 296–301 CrossRef CAS PubMed.
- M. Amjadi, A. Pichitpajongkit, S. Lee, S. Ryu and I. Park, Highly Stretchable and Sensitive Strain Sensor Based on Silver Nanowire–Elastomer Nanocomposite, ACS Nano, 2014, 8(5), 5154–5163 CrossRef CAS PubMed.
- E. Roh, B.-U. Hwang, D. Kim, B.-Y. Kim and N.-E. Lee, Stretchable, Transparent, Ultrasensitive, and Patchable Strain Sensor for Human–Machine Interfaces Comprising a Nanohybrid of Carbon Nanotubes and Conductive Elastomers, ACS Nano, 2015, 9(6), 6252–6261 CrossRef CAS PubMed.
- F. Xu and Y. Zhu, Highly Conductive and Stretchable Silver Nanowire Conductors, Adv. Mater., 2012, 24(37), 5117–5122 CrossRef CAS PubMed.
- J. Kim, S. J. Park, T. Nguyen, M. Chu, J. D. Pegan and M. Khine, Highly stretchable wrinkled gold thin film wires, Appl. Phys. Lett., 2016, 108(6), 061901 CrossRef PubMed.
- X. Wang, H. Hu, Y. Shen, X. Zhou and Z. Zheng, Stretchable Conductors with Ultrahigh Tensile Strain and Stable Metallic Conductance Enabled by Prestrained Polyelectrolyte Nanoplatforms, Adv. Mater., 2011, 23(27), 3090–3094 CrossRef CAS PubMed.
- S. Zhao, J. Li, D. Cao, G. Zhang, J. Li and K. Li,
et al., Recent Advancements in Flexible and Stretchable Electrodes for Electromechanical Sensors: Strategies, Materials, and Features, ACS Appl. Mater. Interfaces, 2017, 9(14), 12147–12164 CrossRef CAS PubMed.
- J. A. Rogers, T. Someya and Y. Huang, Materials and Mechanics for Stretchable Electronics, Science, 2010, 327(5973), 1603–1607 CrossRef CAS PubMed.
- Y. Yoon, K. Samanta, H. Lee, K. Lee, A. P. Tiwari and J. Lee,
et al., Highly Stretchable and Conductive Silver Nanoparticle Embedded Graphene Flake Electrode Prepared by In situ Dual Reduction Reaction, Sci. Rep., 2015, 5(1), 14177 CrossRef CAS PubMed.
- S. Yao and Y. Zhu, Wearable multifunctional sensors using printed stretchable conductors made of silver nanowires, Nanoscale, 2014, 6(4), 2345–2352 RSC.
- S. Gong, D. T. H. Lai, B. Su, K. J. Si, Z. Ma and L. W. Yap,
et al., Highly Stretchy Black Gold E-Skin Nanopatches as Highly Sensitive Wearable Biomedical Sensors, Adv. Electron. Mater., 2015, 1(4), 1400063 CrossRef.
- S. Gong and W. Cheng, One-Dimensional Nanomaterials for Soft Electronics, Adv. Electron. Mater., 2017, 3(3), 1600314 CrossRef.
- J. Paek, J. Kim, B. Wan An, J. Park, S. Ji and S.-Y. Kim,
et al., Stretchable electronic devices using graphene and its hybrid nanostructures, FlatChem, 2017, 3, 71–91 CrossRef CAS.
- H. Zhang, N. Liu, Y. Shi, W. Liu, Y. Yue and S. Wang,
et al., Piezoresistive Sensor with High Elasticity Based on 3D Hybrid Network of Sponge@CNTs@Ag NPs, ACS Appl. Mater. Interfaces, 2016, 8(34), 22374–22381 CrossRef CAS PubMed.
- J. Mu, C. Hou, G. Wang, X. Wang, Q. Zhang and Y. Li,
et al., An Elastic Transparent Conductor Based on Hierarchically Wrinkled Reduced Graphene Oxide for Artificial Muscles and
Sensors, Adv. Mater., 2016, 28(43), 9491–9497 CrossRef CAS PubMed.
- F. Xu, W. Lu and Y. Zhu, Controlled 3D Buckling of Silicon Nanowires for Stretchable Electronics, ACS Nano, 2011, 5(1), 672–678 CrossRef CAS PubMed.
- Y. Yu, J. Zeng, C. Chen, Z. Xie, R. Guo and Z. Liu,
et al., Three-Dimensional Compressible and Stretchable Conductive Composites, Adv. Mater., 2014, 26(5), 810–815 CrossRef CAS PubMed.
- X. Li, P. Sun, L. Fan, M. Zhu, K. Wang and M. Zhong,
et al., Multifunctional graphene woven fabrics, Sci. Rep., 2012, 2, 395 CrossRef PubMed.
- S. Gong, W. Schwalb, Y. Wang, Y. Chen, Y. Tang and J. Si,
et al., A wearable and highly sensitive pressure sensor with ultrathin gold nanowires, Nat. Commun., 2014, 5(1), 3132 CrossRef PubMed.
- Y. Zhu and J. Moran-Mirabal, Highly Bendable and Stretchable Electrodes Based on Micro/Nanostructured Gold Films for Flexible Sensors and Electronics, Adv. Electron. Mater., 2016, 2(3), 1500345 CrossRef.
- S. J. Park, J. Kim, M. Chu and M. Khine, Highly Flexible Wrinkled Carbon Nanotube Thin Film Strain Sensor to Monitor Human Movement, Adv. Mater. Technol., 2016, 1(5), 1–8 Search PubMed.
- S. M. Woo, C. M. Gabardo and L. Soleymani, Prototyping of wrinkled nano-/microstructured electrodes for electrochemical DNA detection, Anal. Chem., 2014, 86(24), 12341–12347 CrossRef CAS PubMed.
- C. M. Gabardo, Y. Zhu, L. Soleymani and J. M. Moran-Mirabal, Bench-Top Fabrication of Hierarchically Structured High-Surface-Area Electrodes, Adv. Funct. Mater., 2013, 23(24), 3030–3039 CrossRef CAS.
- R. C. Adams-McGavin, Y. Chan, C. M. Gabardo, J. Yang, M. Skreta and B. C. Fung,
et al., Nanoporous and wrinkled electrodes enhance the sensitivity of glucose biosensors, Electrochim. Acta, 2017, 242, 1–9 CrossRef CAS.
- E. H. Sargent, Infrared photovoltaics made by solution processing, Nat. Photonics, 2009, 3(6), 325–331 CrossRef CAS.
- C. M. Gabardo, R. C. Adams-McGavin, B. C. Fung, E. J. Mahoney, Q. Fang and L. Soleymani, Rapid prototyping of all-solution-processed multi-lengthscale electrodes using polymer-induced thin film wrinkling, Sci. Rep., 2017, 7(February), 42543 CrossRef CAS PubMed.
- C.-S. Chen, D. N. Breslauer, J. I. Luna, A. Grimes, W. Chin and L. P. Lee,
et al., Shrinky-Dink microfluidics: 3D polystyrene chips, Lab Chip, 2008, 8(4), 622 RSC.
- C. M. Gabardo, J. Yang, N. J. Smith, R. C. Adams-McGavin and L. Soleymani, Programmable Wrinkling of Self-Assembled Nanoparticle Films on Shape Memory Polymers, ACS Nano, 2016, 10(9), 8829–8836 CrossRef CAS PubMed.
- C. M. Gabardo, R. C. Adams-McGavin, O. M. Vanderfleet and L. Soleymani, Rapid prototyping of microfluidic devices with integrated wrinkled gold micro-/nano textured electrodes for electrochemical analysis, Analyst, 2015, 140(16), 5781–5788 RSC.
- T. P. Tuomainen, K. Nyyssönen, R. Salonen, A. Tervahauta, H. Korpela and T. Lakka,
et al., Body iron stores are associated with serum insulin and blood glucose concentrations. Population study in 1,013 eastern Finnish men, Diabetes Care, 1997, 20(3), 426–428 CrossRef CAS PubMed.
- M. Egi, R. Bellomo, E. Stachowski, C. J. French, G. K. Hart and C. Hegarty,
et al., Blood glucose concentration and outcome of critical illness: The impact of diabetes*, Crit. Care Med., 2008, 36(8), 2249–2255 CrossRef CAS PubMed.
- R. D. Rocklin, A. P. Clarke and M. Weitzhandler, Improved Long-Term Reproducibility for Pulsed Amperometric Detection of Carbohydrates via a New Quadruple-Potential Waveform, Anal. Chem., 1998, 70(8), 1496–1501 CrossRef CAS.
- D. S. Austin-Harrison and D. C. Johnson, Pulsed amperometric detection based on direct and indirect anodic reactions: A review, Electroanalysis, 1989, 1(3), 189–197 CrossRef CAS.
- D. C. Johnson and W. R. LaCourse, Liquid Chromatography with Pulsed Electrochemical Detection at Gold and Platinum Electrodes, Anal. Chem., 1990, 62(10), 589A–597A CrossRef CAS.
- J.-C. Chen, H.-H. Chung, C.-T. Hsu, D.-M. Tsai, A. S. Kumar and J.-M. Zen, A disposable single-use electrochemical sensor for the detection of uric acid in human whole blood, Sens. Actuators, B, 2005, 110(2), 364–369 CrossRef CAS.
- A. Kratz, M. Ferraro, P. M. Sluss, K. B. Lewandrowski, R. C. Cabot, N. L. Harris, J.-A. O. Shepard, S. H. Ebeling, S. M. Ellender and C. C. Peters, Normal Reference Laboratory Values, N. Engl. J. Med., 2004, 351(15), 1548–1563 CrossRef CAS PubMed.
- I. Lavagnini, R. Antiochia and F. Magno, A calibration-base method for the evaluation of the detection limit of an electrochemical biosensor, Electroanalysis, 2007, 19(11), 1227–1230 CrossRef CAS.
- N. Liu, A. Chortos, T. Lei, L. Jin, T. R. Kim and W.-G. Bae,
et al., Ultratransparent and stretchable graphene electrodes, Sci. Adv., 2017, 3(9), e1700159 CrossRef PubMed.
- J. Lee, S. Kim, J. Lee, D. Yang, B. C. Park and S. Ryu,
et al., A stretchable strain sensor based on a metal nanoparticle thin film for human motion detection, Nanoscale, 2014, 6(20), 11932–11939 RSC.
- Z. F. Liu, S. Fang, F. A. Moura, J. N. Ding, N. Jiang and J. Di,
et al., Hierarchically buckled sheath-core fibers for superelastic electronics, sensors, and muscles, Science, 2015, 349(6246), 400–404 CrossRef CAS PubMed.
- G. Wang, X. He, L. Wang, A. Gu, Y. Huang and B. Fang,
et al., Non-enzymatic electrochemical sensing of glucose, Microchim. Acta, 2013, 180(3–4), 161–186 CrossRef CAS.
- K. C. Grabar, R. G. Freeman, M. B. Hommer and M. J. Natan, Preparation and Characterization of Au Colloid Monolayers, Anal. Chem., 1995, 67(4), 735–743 CrossRef CAS.
- I. Byun, A. W. Coleman and B. Kim, Transfer of thin Au films to polydimethylsiloxane (PDMS) with reliable bonding using (3-mercaptopropyl)trimethoxysilane (MPTMS) as a molecular adhesive, J. Micromech. Microeng., 2013, 23(8), 085016 CrossRef.
- I. Miccoli, F. Edler, H. Pfnür and C. Tegenkamp, The 100th anniversary of the four-point probe technique: the role of probe geometries in isotropic and anisotropic systems, J. Phys.: Condens. Matter, 2015, 27(22), 223201 CrossRef CAS PubMed.
|
This journal is © The Royal Society of Chemistry 2019 |