The effect of bisphenol A on testicular steroidogenesis and its amelioration by quercetin: an in vivo and in silico approach†
Received
3rd June 2017
, Accepted 13th September 2017
First published on 14th September 2017
Abstract
Bisphenol A (BPA), a phenyl ring containing synthetic xenoestrogen, is widely used in the manufacture of polycarbonate plastics, epoxy resins and as a non-polymer additive to other plastics. Food is considered as the main source of exposure to BPA as it leaches out from food containers as well as surface coatings. It causes toxicity in the liver, kidney, brain, and other organs by initiating the process of lipid peroxidation. The present investigation was an attempt to evaluate the effect of BPA on steroidogenesis and its amelioration by quercetin. Inbred Swiss strain male albino mice were orally administered with 80, 120 and 240 mg per kg body weight per day of BPA for 45 days. The results revealed that BPA causes significant (p < 0.05) and dose-dependent changes in the body weight and biochemical parameters like protein, cholesterol and lipid contents as well as activities of 3β-and 17β-hydroxysteroid dehydrogenases in the testis of mice. It was also found to significantly reduce the testosterone level in serum. Oral administration of quercetin (30, 60 and 90 mg per kg body weight per day) along with a high dose of BPA (240 mg per kg body weight per day) for 45 days caused significant amelioration in the body weight and steroidogenesis as compared to the BPA alone treated group. The effect was dose-dependent. This amelioration in BPA-induced toxicity might be due to the antioxidative properties of quercetin. The reduction in the function of enzymes was confirmed by in silico bindings. BPA and quercetin show competitive binding with steroidogenic enzymes as well as binding with each other. This could be a possible mechanism to reduce the toxic effect of BPA which has been supported by molecular dynamics simulations for molecular level recognition with structural insights.
Introduction
Bisphenol A (BPA), 2,2-bis (4-hydroxyphenyl)propane is a well-known xenoestrogen,1,2 one of the highest volume produced chemicals worldwide.3 It gives characteristics like clearness and toughness to plastic materials and so it is widely used in the preparation of epoxy resins (water pipes) and polycarbonate plastics (food and drink packaging, water bottles, sports equipment, and compact discs).4,5 Food is considered as the main source of exposure as BPA leaches out from food containers as well as from surface coatings.6–9 Some dental sealants and composites may also contribute to its exposure.10 It is also present in cigarette filters, and thus inhalation of cigarette smoke may expose individuals to BPA.11 Significant BPA exposure to children is from toys, books and feeding bottles.12–14
BPA acts as an endocrine disrupting chemical.15,16 In addition, it also increases oxidative stress by forming reactive oxygen species (ROS),15,17–19 which is independent of its estrogenic activity. According to an in vitro and in silico study, BPA induces oxidative stress20 and has a toxic effect on the liver, kidney, and other vital organs.11,21,22 However, its effect on testicular steroidogenesis has remained unexplained.
Quercetin is a strong antioxidant and a major dietary flavonoid. It is found in many plants and foods, such as red wine, onions, green tea, apples, berries, and others.23 It has the ability to prevent oxidation by scavenging free radicals and chelating transition metal ions.23–25
The molecular docking simulation technique has been evaluated for the exploration of the possible binding modes of receptors with selected ligands which reveals binding energetics and molecular interactions.26,27 Molecular dynamics simulations have been performed for the exploration of the possible binding nodes of selected targets to determine the structural integrity, binding energetics, molecular interactions and induced conformational changes.27,28
The present study is an attempt to investigate the effect of BPA administration on testicular steroidogenesis in male Swiss albino mice and its possible amelioration using quercetin, and moreover to confirm its binding with enzymes involved in steroidogenesis.
Materials and methods
Chemicals
Analytical grade BPA and quercetin were purchased from Hi Media Laboratories Pvt. Ltd, Mumbai, India. Olive oil was obtained from Figaro, Madrid, Spain. All the other chemicals used in the study were of AR grade and procured from Hi Media Laboratories Pvt. Ltd, Sisco Research Laboratories Pvt. Ltd, Mumbai, India and Sigma-Aldrich St. Louis, MO, USA.
Experimental animals
In the experiment, inbred healthy adult Swiss strain male albino mice weighing 35–40 g obtained from Cadila Research Center, Ahmedabad, India, were kept in the Animal House of Zoology Department of Gujarat University, Ahmedabad, India. They were housed in an air conditioned room at a temperature of 25 ± 2 °C and 50–55% relative humidity with a 12 h light/dark cycle throughout the experiment. Animals were fed with certified pelleted rodent feed supplied by Amrut Feeds, Pranav Agro Industries Ltd, Pune, India and potable water ad libitum. All the experimental protocols were approved by the Committee for the Purpose of Control and Supervision of Experiments on Animals (Reg. – 167/1999/CPCSEA), New Delhi, India. Animals were handled according to the guidelines published by Indian National Science Academy, New Delhi, India (1991).
Dose selection
Different doses of BPA were selected based on the LD50 value.29 Animals of BPA-treated groups received three different doses of BPA, which are 1/10th, 1/20th and 1/30th of LD50 (240, 120 and 80 mg per kg bw per day respectively) for 45 days. For the amelioration of BPA-induced toxicity different doses of quercetin (30, 60 and 90 mg per kg bw per day) were selected on the basis of the study of Sangai and Verma.30
Experimental design
The experimental protocol is shown in Table 1. Mice were randomly divided into nine groups, each containing 10 animals. The treatment schedule of the animals was as follows. Animals from group I (untreated control) were kept untreated and given free access to food and water. Group II (vehicle control) animals were administered with olive oil (0.2 ml per animal per day), which has been used as a vehicle to dissolve BPA. Group III (antidote control) animals were treated with quercetin (90 mg per kg body weight per day), which has been used for amelioration of BPA-induced toxicity.
Table 1 Experimental protocol
Experimental groups |
Number of animals |
Duration (days) |
Necropsy |
Control groups |
I |
Untreated control |
10 |
45 |
46th |
II |
Vehicle control (0.2 ml olive oil per animal per day) |
10 |
45 |
46th |
III |
Antidote control (0.2 ml quercetin per animal per day) |
10 |
45 |
46th |
BPA-treated groups |
IV |
BPA-low dose (80 mg per kg body weight per day) |
10 |
45 |
46th |
V |
BPA-medium dose (120 mg per kg body weight per day) |
10 |
45 |
46th |
VI |
BPA-high dose (240 mg per kg body weight per day) |
10 |
45 |
46th |
HD BPA + quercetin treated groups |
VII |
BPA-HD + quercetin (30 mg per kg body weight per day) |
10 |
45 |
46th |
VIII |
BPA-HD + quercetin (60 mg per kg body weight per day |
10 |
45 |
46th |
IX |
BPA-HD + quercetin) (90 mg per kg body weight per day) |
10 |
45 |
46th |
Animals of groups IV, V and VI received three different doses of BPA (80, 120 and 240 mg per kg bw per day) for 45 days, however, animals of groups VII, VIII and IX received three different doses of quercetin (30, 60 and 90 mg per kg bw per day) along with a high dose of BPA (240 mg per kg bw per day). All treatments were given orally using a feeding tube attached to a hypodermic syringe for 45 days.
Analysis of biochemical parameters
After treatment, the animals were sacrificed on the 46th day by using anesthetic ether. Blood samples were collected by cardiac puncture in non-anticoagulant added tubes, allowed to clot for 20–30 min and centrifuged at 1000g for 10 min at 4 °C. The non-haemolysed serum samples obtained were stored at −20 °C and used for analysis of the serum testosterone level. The testis was dissected out quickly, blotted free from blood and used for determining the biochemical parameters such as protein, lipid and cholesterol contents as well as the activities of 3β- and 17β-hydroxysteroid dehydrogenases.
Protein content.
The protein content in the testis of the control and all treated groups of animals was estimated by the method of Lowry et al. (1951)31 using bovine serum albumin as the standard. The protein containing preparation reacts with the Folin and Ciocalteu's phenol reagent to give a colored complex. This color development is due to two reactions occurring simultaneously i.e. the reaction of alkaline copper sulphate solution with peptide bonds and reduction of phosphotungstic acids by aromatic amino acids like tyrosine and tryptophan present in the protein. The blue colour developed is quantitatively proportional to the total protein, which is measured colorimetrically. The optical density (O.D.) of the blue colour developed was read at 540 nm on a Systronics 106 colorimeter. The protein content was expressed as mg per 100 mg tissue weight.
Lipid content.
The total lipid content in the testis was estimated according to the method of Frings et al. (1972)32 using olive oil as a standard. Lipid on being heated with sulphuric acid followed by addition of vanillin and phosphoric acid produces a pink color whose optical density is measured at 530 nm. The total lipid content was expressed as mg per 100 mg of tissue weight.
Cholesterol content.
The concentration of cholesterol was estimated in the testis of the control and all treated groups of mice by the method of Zlatki et al. (1953).33 In the presence of concentrated sulphuric acid and glacial acetic acid, cholesterol forms a colored complex with ferric chloride (FeCl3) that can be measured colorimetrically at 540 nm. The cholesterol content was expressed as mg per 100 mg tissue weight.
3β-Hydroxysteroid dehydrogenase (E.C.1.1.1.51) activity.
The 3β-hydroxysteroid dehydrogenase (3β-HSD) activity was assayed by the method of Talalay (1962)34 in the testis of the control and all treated groups of animals. The enzyme 3β-hydroxysteroid dehydrogenase acts on the substrate epiandrosterone (Sigma Chemical Co., USA) in the presence of nicotinamide adenine dinucleotide (NAD+) which is reduced to NADH and androstenedione was formed. The absorbance was measured at 340 nm on a UV-Vis spectrophotometer. The enzyme activity was expressed as HSD-n moles of androstenedione formed per mg protein per min.
17β-Hydroxysteroid dehydrogenase (E.C.1.1.1.51) activity.
The testicular 17β-hydroxysteroid dehydrogenase (17β-HSD) activity was assayed by the method of Talalay (1962)34 in the testis of the control and all treated groups. The enzyme 17-β-hydroxysteroid dehydrogenase acts on the substrate testosterone in the presence of nicotinamide adenine dinucleotide (NAD+) which is reduced to NADH and androstenedione was formed. The absorbance was measured at 340 nm on a UV-Vis spectrophotometer. The enzyme activity was expressed as HSD-n moles of androstenedione formed per mg protein per min.
Serum testosterone level
Serum samples from the control and all treated animals were collected. The testosterone level in serum was estimated by the chemiluminescence immunoassay (CLIA) method using an Advia Centaur XP (Siemens) Immunoassay system.
Statistical analysis
The results were expressed as mean ± SEM. The data were statistically analysed using one way Analysis of Variance (ANOVA) followed by Tukey's post hoc test in Graph pad prism 5 (graph pad, software, USA). Statistical significance was accepted with p < 0.05. The correlation coefficient was measured to estimate the strength of linear association between two variables. Pearson's correlation analysis was used to find the correlation between the vehicle control and BPA toxicant treated groups.
Molecular docking
To identify the plausible mechanism of BPA and quercetin, molecular docking has been performed using YASARA software.35–37 This analysis provided the interaction and structural insights into BPA and quercetin compounds. Three dimensional structures of 3beta/17beta-hydroxysteroid dehydrogenases having resolutions 1.22 Å and 1.6 Å were retrieved from the RCSB (Research Collaboratory for Structural Bio-informatics) protein data bank with the pdb code of 1hxh and 5fyd. We selected two proteins for the molecular docking of 1HXH and 5FYD. 1HXH does not contain any ligand in its three dimensional structure, so there is no possibility for re-docking. We had performed blind docking for 1HXH. The same approach was carried out for 5FYD, because it possesses glycerol in its structure. We have chosen the binding pocket based on the literature review on their PDB pages. Both the ligands were docked individually to invoke the structural affinity. Protein preparation and ligand preparation have been performed for this analysis by using YASARA software,35 which includes water removal, binding site identification, simulation box generation and energy minimization.38 Molecular docking analysis demonstrates the best binding conformations between the receptor and ligand with the best binding energy which can be calculated using the following equation:27
ΔG = ΔGvdW + ΔGHbond + ΔGelec + ΔGtor + ΔGdesolv |
where ΔGvdW = van der Waals term for docking energy, ΔGHbond = H bonding term for docking energy, ΔGelec = electrostatic term for docking energy, ΔGtor = torsional free energy term for the ligand when the ligand transits from the unbound to the bound state, and ΔGdesolv = desolvation term for docking energy.
After the completion of the molecular docking experiment, the interaction profiles have been evaluated to understand the mechanism of better binding affinity37,39 which involves van der Waals, conventional hydrogen bond, alkyl, Pi-alkyl, unfavourable donor–donor, unfavourable acceptor–acceptor, Pi–Pi T-shaped, carbon hydrogen bond, Pi–anion and Pi–Sigma40 interactions to anticipate the interacting atoms with the respective selected receptors.
Molecular dynamics simulation
To understand the natural state effect with conformational changes and structural stability of receptor–ligand complexes, molecular dynamics simulations have been carried out for a 5 ns time trajectory using YASARA software.27,36,41 The structural mechanism of the receptor–ligand complexes was evaluated by using the key intermolecular interactions and molecular mechanics.42,43 Newton's equations of motion are being utilized for MD simulations which determine the atom distribution in the specific force field level (Amber03 force field).39 The real system prepared with the pKa (acid dissociation constant) values of protein titratable amino acids which includes different parameters like energy minimization Amber03 force field, neutralization, periodic boundary pKa (acid dissociation constant) values, and pressure and temperature maintenance. The transferable intermolecular potential 3 points (TIP3P) of the water model (density: 0.997 g l−1) was solvated by using an orthorhombic simulation box with the steepest gradient approach for the energy minimization (100 cycles) and neutralized in 0.9% NaCl. The Berendsen thermostat and barostat43–45 were used for the pressure (1 bar) and temperature maintenance at 298 K (with pH = 7.4 under physiological conditions).41 The time trajectories of the selected receptor–ligand complexes have been evaluated at every 1 ns time period to understand the stabilization and conformational change in the native structure at different time points.
Results and discussion
Clinical symptoms
The oral administration of BPA for 45 days in mice caused reduction in feed intake, dullness, lethargy and body hair loss. Treatment related mortality was absent in all the treated groups. No treatment related clinical signs were observed in the untreated, vehicle and antidote control group animals. No other abnormal clinical symptoms were observed.
Body weight
The present study revealed that the oral administration of BPA caused a significant (p < 0.05), dose-dependent reduction in the body weight as well as absolute and relative weights of the testis as compared to the vehicle control group of animals.45 No significant change in the body weight was observed between the animals of the untreated and vehicle control groups (groups I, II and III). As compared to the vehicle control, the oral administration of BPA caused reduction in the body weight (groups IV, V and VI). The effect was significant (p < 0.05) and dose-dependent (r = 0.843) (Table 2). The reduction in the body weight and organ weights could be due to the decrease in the feed intake and/or biochemical changes observed. Co-treatment with different concentrations of quercetin along with high doses of BPA (groups VII–IX) caused a significant (p < 0.05) increase in the body weight as compared to the BPA-HD group (group VI). This effect was dose dependent (r = 0.819). This could be due to the improvement in the biochemical changes due to the anti-oxidative properties of quercetin. In the in vivo experiment conducted by Zheng et al. (2016), quercetin (100, 150 and 200 mg kg−1) was administered through intraperitoneal injection. Chen et al. (2017) have administered different doses of quercetin (30, 60 and 120 mg kg−1) in rats for a protective study. Quercetin showed beneficial effects and no toxicity was observed.46–48
Table 2 Effect of BPA treatment on the body weight (g) of mice and its amelioration by quercetin
Sr. no. |
Experimental group |
Days of treatment |
0 |
45 |
Values are mean ± S.E.M., n = 10. Level of significance. p < 0.05 as compared to the vehicle control. p < 0.05 as compared to the BPA-treated group. No significant difference was noted between untreated and vehicle control groups. |
Control groups |
I |
Untreated control |
40.10 ± 0.35 |
40.00 ± 0.49 |
II |
Vehicle treated control (0.2 ml olive oil per animal per day) |
40.20 ± 0.39 |
39.80 ± 0.47 |
III |
Antidote control (90 mg quercetin per kg body weight day) |
39.90 ± 0.46 |
40.00 ± 0.57 |
BPA-treated groups |
IV |
BPA-low dose (80 mg per kg body weight per day) |
39.80 ± 0.42 |
36.30 ± 0.45a |
V |
BPA-medium dose (120 mg per kg body weight per day) |
40.10 ± 0.41 |
34.22 ± 0.44a |
VIII |
BPA-high dose (240 mg per kg body weight per day) |
40.10 ± 0.38 |
31.20 ± 0.53a |
BPA (HD) + quercetin-treated groups |
VII |
BPA-HD + quercetin (30 mg per kg body weight per day) |
39.90 ± 0.41 |
34.33 ± 0.32b |
VIII |
BPA-HD + quercetin (60 mg per kg body weight per day) |
39.80 ± 0.42 |
36.89 ± 0.51b |
IX |
BPA-HD + quercetin (90 mg per kg body weight per day) |
39.90 ± 0.43 |
39.40 ± 0.54b |
No significant alteration was observed in the absolute and relative testis weights of the untreated and vehicle control groups (groups I and II). The results revealed that the oral administration of BPA (groups III–V) for 45 days caused a significant (p < 0.05), dose-dependent decrease in the absolute (r = 0.977) and relative (r = 0.874) weights of the testis as compared to the vehicle control group (group II). The maximum reduction in the absolute and relative weights were noted up to 31.36% and 21.78%, respectively, in the high dose BPA-treated group of animals (Table 3). The administration of different concentrations of quercetin (30, 60, and 90 mg per kg body weight per day) along with high doses of BPA (groups VII, VIII and IX) shows dose-dependent amelioration in the absolute (r = 0.968) and relative weights (r = 0.822), respectively, as compared to high doses of BPA (Table 3). The oral administration of quercetin along with a high dose of BPA shows a dose-dependent increase in the body weight and organ weight. This could be due to the improvement in the biochemical changes and anti-oxidative properties of quercetin.24
Table 3 Effect of BPA treatment on the testis weight of mice and its amelioration by quercetin
Sr. no. |
Experimental groups |
Absolute weight |
Relative weight |
Values are mean ± S.E.M.; n = 10. Level of significance. p < 0.05, as compared to the untreated control. p < 0.05, as compared to the BPA-HD-treated group. No significant difference was noted between different control groups (groups I–III). Units: absolute weight, mg; relative weight, mg per 100 g body weight. |
Control groups |
I |
Untreated control |
217.40 ± 0.50 |
567.88 ± 6.19 |
II |
Vehicle control (0.2 ml olive oil per animal per day) |
217.80 ± 0.38 |
564.24 ± 5.01 |
III |
Antidote control (0.2 ml quercetin per animal per day) |
216.50 ± 0.460 |
563.75 ± 3.15 |
BPA-treated groups |
III |
BPA-low dose (80 mg per kg body weight per day) |
188.20 ± 0.49a |
523.33 ± 2.53a |
IV |
BPA-medium dose (120 mg per kg body weight per day) |
170.80 ± 0.52a |
481.78 ± 7.36a |
V |
BPA-high dose (240 mg per kg body weight per day) |
149.50 ± 0.48a |
441.34 ± 10.69a |
BPA (HD) + quercetin-treated groups |
VII |
BPA HD + quercetin (30 mg per kg body weight per day) |
176.90 ± 0.57b |
497.63 ± 6.16b |
VIII |
BPA HD + quercetin (60 mg per kg body weight per day) |
194.60 ± 0.51b |
526.17 ± 3.82b |
IX |
BPA HD + quercetin (90 mg per kg body weight per day) |
216.50 ± 0.52b |
554.61 ± 5.17b |
Biochemical analysis
Steroidogenesis is a complex multi-enzyme process by which cholesterol is converted to biologically active steroid hormones. Cholesterol is a precursor for steroid hormones and its concentration is significantly (p < 0.05) decreased as per BPA doses. A biochemical study shows that there is a dose-dependent decrease in 3β- and 17β-hydroxysteroid dehydrogenase enzymes (Table S1†). The transformation of delta-5-3-beta-hydroxysteroid into the corresponding delta-4-3-keto-steroids is an essential step for the biosynthesis of androgens. These steroid hormones play a crucial role in the differentiation, development, growth, and physiological function of most human tissues. There are numerous experimental studies upon laboratory animals which show that BPA can affect the development of gonads, accessory glands and spermatogenesis38,49–52 by the formation of reactive oxygen species.14–16
The oral administration of BPA for 45 days (groups III–V) caused a significant (p < 0.05), dose-dependent decrease in total lipid (r = 0.722) and cholesterol contents (r = 0.971) as compared to the vehicle control group II. Total protein (r = 0.937) estimated in the testis was found to be significantly decreased according to doses. BPA also caused a significant (p < 0.05), dose-dependent reduction in the activities of 3β- (r = 0.869) and 17β-hydroxysteroid dehydrogenase (r = 0.922) as compared to the vehicle control. BPA treatment also caused a significant (p < 0.05), dose dependent decrease in the testosterone level (r = 0.983) in serum (Table S1†). The maximum alterations were found with high doses of BPA in all parameters. No significant changes in the biochemical parameters were observed between different control groups of animals (groups I–III). The administration of quercetin along with high doses of BPA (groups VII–IX) caused a significant (p < 0.005), dose-dependent increase in total protein (r = 0.903), lipid (r = 0.722) and cholesterol contents (r = 0.949) as compared to the BPA high dose alone treated group (group VI). Similarly, co-treatment of quercetin with BPA (high dose) caused a significant (p < 0.05), dose-dependent increase in the activity of 3β-hydroxysteroid dehydrogenase (r = 0.897) and 17β-hydroxysteroid dehydrogenase (r = 0.928). Maximum protection was achieved at the high dose of quercetin.
The result revealed that the administration of quercetin along with high doses of BPA caused a significant (p < 0.05), dose dependent increase in the total contents of protein, lipid and cholesterol as well as the activity of 3β and 17β-hydroxysteroid dehydrogenases. Quercetin is considered to be a strong antioxidant due to its ability to scavenge free radicals and bind transition metal ions. These properties of quercetin allow the inhibition of lipid peroxidation.22
BPA is a chemical with an estrogenic effect.1 It causes a significant (p < 0.05), dose-dependent reduction of steroid hormones in the serum of Swiss albino mice after 45 days of treatment.40,41 Testosterone deprivation can suppress spermatogenesis, leading to low sperm count. Thus reduction in testosterone may cause sexual dysfunction. Degeneration in Leydig cells could also affect the testosterone level.42,43,52 This effect is caused by direct action upon the interaction between Sertoli cells and germinal line cells. The reproductive toxicity is caused by their interaction with androgen and estrogen receptors.43–45,49,53–56 Quercetin ameliorates BPA-induced toxicity, which could be due to its antioxidative properties. Co-treatment with quercetin along with BPA-HD caused a significant (p < 0.05), dose-dependent (r = 0.972) increase in the testosterone hormone level by 36.18%, 69.37% and 96.22% as calculated from the organ protective index.
The in vivo study revealed that the oral administration of BPA causes a reduction in the activity of steroidogenic enzymes that leads to a reduction in the testosterone level. The toxic effect of BPA was mitigated by quercetin. To find out the possible mechanism behind the effect on steroidogenesis, we used in silico tools.
Molecular docking of BPA and quercetin into steroid binding proteins (3beta/7beta/17beta-hydroxysteroid dehydrogenases)
The binding affinities of BPA and quercetin towards 1hxh and 5fyd are investigated in detail. The number of H-bonds and binding residues of 1hxh and 5fyd with both compounds are shown in Table 4. From the post docking analysis, it is identified that quercetin possesses the highest binding affinity to both proteins with the four and five hydrogen bonds including Ser 16, Ile 18, Asp 37 and Gly 89 with 1hxh and Gly 15, Glu 18, Gly 39, Asp 66 and Cys 93 with 5fyd, respectively. A binding energy of 7.852 kcal mol−1 was obtained from 1hxh–quercetin docking, while a binding energy of 7.896 kcal mol−1 has been retrieved from 5fyd–quercetin docking analysis.
Table 4 Molecular docking results analysis: binding energy, hydrogen bonds, contacting residues
Proteins |
Ligands |
Binding energy [kcal mol−1] |
Hydrogen bonds |
Contacting receptor residues |
1hxh |
BPA |
6.888 |
Asp 184 |
Ser 16, Gly 17, Val 18, Gly 19, Asn 87, Leu 91, Ser 138, Tyr 151, Asp 184, Gly 185, Ile 186, Thr 188, Pro 219 |
|
Quercetin |
7.852 |
Ser 16, Ile 18, Asp 37, Gly 89 |
Gly 13, Ala 15, Ser 16, Gly 17, Val 18, Asp 37, Ile 38, Asn 87, Ala 88, Gly 89, Ile 90, Leu 91, Tyr 151, Lys 155, Asp 184, Gly 185, Ile 186, Thr 188, Met 191 |
5fyd |
BPA |
7.185 |
Asp 66 |
Gly 15, Thr 17, Glu 18, Gly 39, Arg 40, Arg 41, Ala 65, Asp 66, Phe 67, Ser 68, Val 91, Ala 92, Cys 93, Leu 94, Val 114 |
|
Quercetin |
7.896 |
Gly 15, Glu 18, Gly 39, Asp 66, Cys 93 |
Gly 15, Thr 17, Glu 18, Gly 39, Arg 40, Arg 41, Ala 65, Asp 66, Phe 67, Ser 68, Val 91, Ala 92, Cys 93, Leu 94, Val 114, Lys 160 |
The conventional hydrogen bonds of the 1hxh–quercetin complex (Fig. 1) have been observed to be higher in number when compared to the 1hxh–BPA complex (Fig. 2) which mainly possesses three conventional hydrogen bonds at the hydroxyl positions with Ser 16 and Asp 37 with the distances of 4.58, 4.60 and 5.36 Å. That means hydrogen bonding plays a vital role in the binding of the 1hxh–quercetin complex, while the alkyl interactions have disappeared in the 1hxh–quercetin complex which shows greater association with BPA binding. The vast difference determined in the formation of van der Waals interactions which remains 10 in number with the 1hxh–BPA complex becomes higher up to 15 in number in the 1hxh–quercetin complex. And the Pi–alkyl interactions remained the same in both docked compounds.
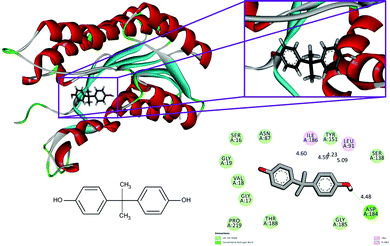 |
| Fig. 1 Binding modes of BPA with the crystal structure of 3beta/17beta hydroxysteroid dehydrogenase protein (PDB code: 1hxh), receptor–ligand interactions with 2D representation of BPA. | |
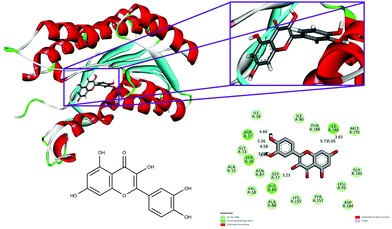 |
| Fig. 2 Binding modes of quercetin with the crystal structure of 3beta/17beta hydroxysteroid dehydrogenase protein (PDB code: 1hxh), receptor–ligand interactions with 2D representation of quercetin. | |
With respect to the docking of 5fyd and above mentioned two compounds, the conventional hydrogen bond remains the same at the position of Asp 66 in both 5fyd–BPA (Fig. 3), and 5fyd–quercetin complexes. Decreased numbers have been listed in the 5fyd–quercetin complex when compared to the 5fyd–BPA complex (Fig. 4), and 4 carbon hydrogen bonds have been retrieved. The addition of an unfavourable donor–donor as well as a Pi–anion have been noticed in the 5fyd–quercetin complex which makes quercetin more suitable for binding (Table 4). These observations are significant and might be the probable cause for the higher affinity of quercetin to the 1hxh and 5fyd complexes.
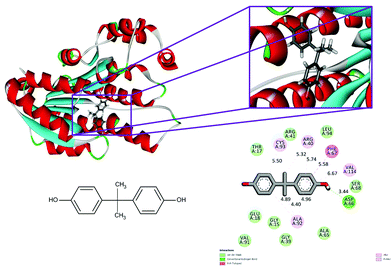 |
| Fig. 3 Binding modes of BPA with the crystal structure of 7beta-hydroxysteroid dehydrogenase protein (PDB code: 5fyd), receptor–ligand interactions with 2D representation of BPA. | |
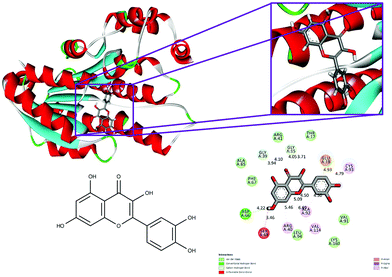 |
| Fig. 4 Binding modes of quercetin with the crystal structure of 7beta-hydroxysteroid dehydrogenase protein (PDB code: 5fyd), receptor–ligand interactions with 2D representation of quercetin. | |
Molecular dynamics simulation
MD simulations were employed for the evaluation of stabilization and changes occurring in conformational receptor–ligand complexes during the whole event which have been carried out for 10 ns time trajectories. Four MD simulations have been performed to identify the structural conformation of both compounds (BPA and quercetin). The best docked poses of selected complexes were considered for the MD simulations. The equilibrium of the MD trajectories has been evaluated using energy and Root Mean Square Deviation (RMSD) parameters.
We have analysed both energy and RMSD parameters for all selected docked complexes (Fig. 5 and 6). The energy values have been found to be −172
147.543 kcal mol−1 to −171
519.277 kcal mol−1 at the initial stage of MD simulation for all complexes while it remains higher with the value from −130
650.283 kcal mol−1 to −130
294.189 kcal mol−1 at the end of MD simulation for a 10 ns time period. With respect to RMSD calculations it starts with 0.397 Å to 0.424 Å and ends with the 1.927 Å, 2.686 Å values for all simulated complexes. Nominal fluctuations were observed for all receptor–ligand complexes through the MD simulation energy graph, while major fluctuations have been determined by the RMSD graph values.
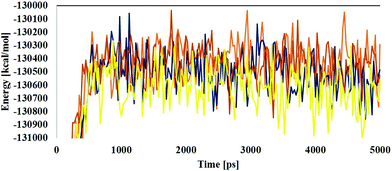 |
| Fig. 5 Molecular dynamics analysis through time vs. energy graph plots of the 1hxh–BPA complex (blue color), 1hxh–quercetin (dark blue color), 5fyd–BPA complex (brown color) and 5fyd–quercetin complex (yellow color). | |
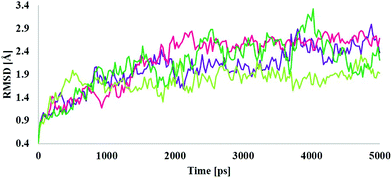 |
| Fig. 6 Molecular dynamics analysis through time vs. RMSD graph plots for the 1hxh–BPA complex (purple color), 1hxh–quercetin (magenta color), 5fyd–BPA complex (light green color) and 5fyd–quercetin complex (dark green color). | |
Interaction analysis through MD simulations
Different interactions have been identified during the whole event of MD simulations for all receptor–ligand complexes. All 1 ns time trajectories have been considered for MD simulation analysis. Conventional hydrogen bond, van der Waals, carbon hydrogen bond, unfavourable donor–donor, alkyl, Pi–alkyl Pi–donor hydrogen bond, Pi–sulfur, amide–Pi–stacked, Pi–Pi–T-shaped and Pi–anion type interactions have been observed in the MD simulations of all receptor–ligand complexes (Fig. 7–10 and Table S2†).
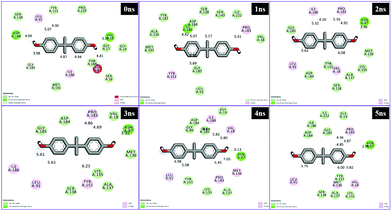 |
| Fig. 7 Schematic representation of the interactions between 1hxh and BPA compounds generated using Discovery studio visualizer. | |
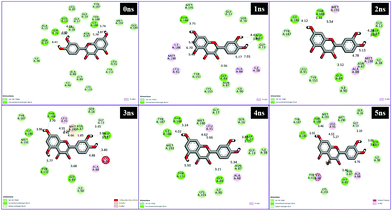 |
| Fig. 8 Schematic representation of the interactions between 1hxh and quercetin compounds generated using Discovery studio visualizer. | |
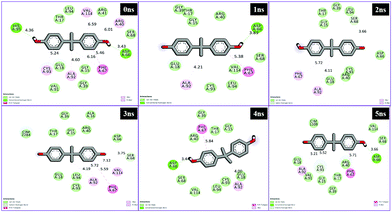 |
| Fig. 9 Schematic representation of the interactions between 5fyd and BPA compounds generated using Discovery studio visualizer. | |
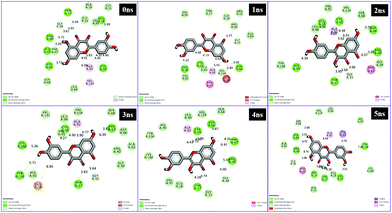 |
| Fig. 10 Schematic representation of the interactions between 1hxh and quercetin compounds generated using Discovery studio visualizer. | |
From the analysis results of 1hxh and 5fyd proteins with both compounds, it is clearly observed that quercetin possesses potential affinity towards both proteins, while BPA shows less affinity to both proteins. The major difference determined by this analysis is about the conventional hydrogen bonds which remain higher in both MD simulations for 1hxh–quercetin and 5fyd–quercetin which determines that quercetin is a potential target for steroidogenesis. To determine the changes occurring in the conformation of proteins we have performed superposition of proteins by considering pre-MD as well as post-MD files. All simulated receptor–ligand complexes show RMSD within 5 Å values in the range of 3.0072 Å, 3.2126 Å, 2.5542 Å and 2.8258 Å (Fig. 11).
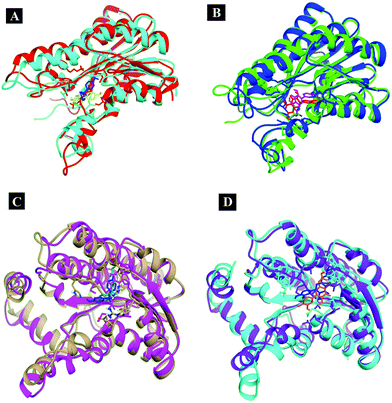 |
| Fig. 11 Superposition of the simulated complexes to decipher the changes occurring in the conformation. | |
Conclusions
The present study concludes that BPA administration for 45 days can cause reduction in the synthesis of steroid hormones52,54 and it causes a significant (p < 0.05), dose-dependent reduction of steroid hormones in the serum of Swiss albino mice. Furthermore, quercetin supplementation can ameliorate the toxicity induced by high doses of BPA. The molecular docking and dynamics simulation study reveals the same plausible mechanism which supports the in vivo study.
Conflicts of interest
The authors declare no conflict of interest.
Acknowledgements
Sanman Samova, Chirag N. Patel and Hetal Doctor acknowledge financial assistance from University Grants Commission (UGC), Govt. of India as Rajiv Gandhi National Fellowship. The authors are sincerely thankful to the Department of Zoology, B.M.T.C. and Human Genetic and Department of Botany, Bioinformatics and Climate Change Impacts Management, Gujarat University for providing an opportunity to access the bioinformatics research facilities.
References
- A. Elango, B. Shepherd and T. T. Chen, Effects of endocrine disrupters on the expression of growth hormone and prolactin mRNA in the rainbow trout pituitary, Gen. Comp. Endocrinol., 2006, 145, 116–127 CrossRef CAS PubMed.
- D. G. Skledar and L. P. Mašič, Bisphenol A and its analogs: Do their metabolites have endocrine activity?, Environ. Toxicol. Pharmacol., 2016, 47, 182–199 CrossRef PubMed.
- J. R. Rochester, Bisphenol A and human health: a review of the literature, Reprod. Toxicol., 2013, 42, 132–155 CrossRef CAS PubMed.
- L. N. Vandenberg, R. Hauser, M. Marcus, N. Olea and W. V. Welshons, Human exposure to bisphenol A (BPA), Reprod. Toxicol., 2007, 24, 139–177 CrossRef CAS PubMed.
- N. von Goetz, R. Pirow, A. Hart, E. Bradley, F. Poças, D. Arcella, I. Lillegard and C. Simoneau, J. van Engelen and T. Husoy, Including non-dietary sources into an exposure assessment of the European Food Safety Authority: The challenge of multi-sector chemicals such as Bisphenol A, Regul. Toxicol. Pharmacol., 2017, 85, 70–78 CrossRef CAS PubMed.
- T. Geens, T. Z. Apelbaum, L. Goeyens, H. Neels and A. Covaci, Intake of bisphenol A from canned beverages and foods on the Belgian market, Food Addit. Contam., 2010, 27, 1627–1637 CrossRef CAS PubMed.
- J.-H. Kang, K. Kito and F. Kondo, Factors influencing the migration of bisphenol A from cans, J. Food Prot., 2003, 66, 1444–1447 CrossRef CAS PubMed.
- M. Fernandez, J. Arrebola, J. Taoufiki, A. Navalón, O. Ballesteros, R. Pulgar, J. Vilchez and N. Olea, Bisphenol-A and chlorinated derivatives in adipose tissue of women, Reprod. Toxicol., 2007, 24, 259–264 CrossRef CAS PubMed.
- J. L. Carwile, H. T. Luu, L. S. Bassett, D. A. Driscoll, C. Yuan, J. Y. Chang, X. Ye, A. M. Calafat and K. B. Michels, Polycarbonate bottle use and urinary bisphenol A concentrations, Environ. Health Perspect., 2009, 117, 1368 CrossRef CAS PubMed.
- K. Suzuki, K. Ishikawa, K. Sugiyama, H. Furuta and F. Nishimura, Content and release of bisphenol A from polycarbonate dental products, Dent. Mater. J., 2000, 19, 389–395 CrossRef CAS.
- R. E. Chapin, J. Adams, K. Boekelheide, L. E. Gray, S. W. Hayward, P. S. Lees, B. S. McIntyre, K. M. Portier, T. M. Schnorr and S. G. Selevan, NTP-CERHR expert panel report on the reproductive and developmental toxicity of bisphenol A, Birth Defects Res., Part B, 2008, 83, 157–395 CrossRef CAS PubMed.
- C. Brede, P. Fjeldal, I. Skjevrak and H. Herikstad, Increased migration levels of bisphenol A from polycarbonate baby bottles after dishwashing, boiling and brushing, Food Addit. Contam., 2003, 20, 684–689 CrossRef CAS PubMed.
- J. Sajiki, R. Yanagibori and Y. Kobayashi, Study of experiment on leaching of bisphenol A from infant books to artificial saliva, Nippon Eiseigaku Zasshi, 2010, 65, 467–470 CrossRef CAS PubMed.
- J. M. Braun and R. Hauser, Bisphenol A and children's health, Curr. Opin. Pediatr., 2011, 23, 233–239 CrossRef CAS PubMed.
- V. Bindhumol, K. Chitra and P. Mathur, Bisphenol A induces reactive oxygen species generation in the liver of male rats, Toxicology, 2003, 188, 117–124 CrossRef CAS PubMed.
- A. Nadal, E. Fuentes, C. Ripoll, S. Villar-Pazos, M. Castellano-Muñoz, S. Soriano, J. Martinez-Pinna, I. Quesada and P. Alonso-Magdalena, Extranuclear-initiated estrogenic actions of endocrine disrupting chemicals: Is there toxicology beyond paracelsus?, J. Steroid Biochem. Mol. Biol., 2017 DOI:10.1016/j.jsbmb.2017.01.014.
- N. R. Gassman, Induction of oxidative stress by bisphenol A and its pleiotropic effects, Environ. Mol. Mutagen., 2017 DOI:10.1002/em.22072.
- H. Kabuto, M. Amakawa and T. Shishibori, Exposure to bisphenol A during embryonic/fetal life and infancy increases oxidative injury and causes underdevelopment of the brain and testis in mice, Life Sci., 2004, 74, 2931–2940 CrossRef CAS PubMed.
- J. Asahi, H. Kamo, R. Baba, Y. Doi, A. Yamashita, D. Murakami, A. Hanada and T. Hirano, Bisphenol A induces endoplasmic reticulum stress-associated apoptosis in mouse non-parenchymal hepatocytes, Life Sci., 2010, 87, 431–438 CrossRef CAS PubMed.
- H. Suthar, R. Verma, S. Patel and Y. Jasrai, Green tea potentially ameliorates bisphenol A-induced oxidative stress: an in vitro and in silico study, Biochem. Res. Int., 2014, 2014, 259763 Search PubMed.
- R. W. Tyl, Commentary to the CERHR expert panel report on bisphenol A, Birth Defects Res., Part B, 2008, 83, 152–152 CrossRef CAS PubMed.
- J. Michałowicz, Bisphenol A–sources, toxicity and biotransformation, Environ. Toxicol. Pharmacol., 2014, 37, 738–758 CrossRef PubMed.
- M. Santos, M. Rodriguez-Gomez, G. Justino, N. Charro, M. Florencio and L. Mira, Influence of the metabolic profile on the in vivo antioxidant activity of quercetin under a low dosage oral regimen in rats, Br. J. Pharmacol., 2008, 153, 1750–1761 CrossRef CAS PubMed.
- Y. Hanasaki, S. Ogawa and S. Fukui, The correlation between active oxygens scavenging and antioxidative effects of flavonoids, Free Radical Biol. Med., 1994, 16, 845–850 CrossRef CAS PubMed.
- G. W. Plumb, K. R. Price and G. Williamson, Antioxidant properties of flavonol glycosides from green beans, Redox Rep., 1999, 4, 123–127 CrossRef CAS PubMed.
- F. Feixas, S. Lindert, W. Sinko and J. A. McCammon, Exploring the role of receptor flexibility in structure-based drug discovery, Biophys. Chem., 2014, 186, 31–45 CrossRef CAS PubMed.
- F. Parmar, C. Patel, H. Highland, H. Pandya and L.-B. George, Antiproliferative Efficacy of Kaempferol on Cultured Daudi Cells: An In Silico and In Vitro Study, Adv. Biol., 2016, 2016, 9521756 Search PubMed.
- Y. Duan, C. Wu, S. Chowdhury, M. C. Lee, G. Xiong, W. Zhang, R. Yang, P. Cieplak, R. Luo and T. Lee, A point-charge force field for molecular mechanics simulations of proteins based on condensed-phase quantum mechanical calculations, J. Comput. Chem., 2003, 24, 1999–2012 CrossRef CAS PubMed.
- R. M. Hussein and J. I. Eid, Pathological mechanisms of liver injury caused by oral administration of bisphenol A, Life Sci. J., 2013, 10(1), 1050–1059 Search PubMed.
- N. P. Sangai, R. J. Verma and M. H. Trivedi, Testing the efficacy of quercetin in mitigating bisphenol A toxicity in liver and kidney of mice, Toxicol. Ind. Health, 2014, 30, 581–597 CrossRef PubMed.
- O. H. Lowry, N. J. Rosebrough, A. L. Farr and R. J. Randall, Protein measurement with the Folin phenol reagent, J. Biol. Chem., 1951, 193, 265–275 CAS.
- C. S. Frings, T. W. Fendley, R. T. Dunn and C. A. Queen, Improved determination of total serum lipids by the sulfo-phospho-vanillin reaction, Clin. Chem., 1972, 18, 673–674 CAS.
- A. Zlatkis, B. Zak and A. J. Boyle, A new method for the direct determination of serum cholesterol, J. Lab. Clin. Med., 1953, 41, 486–492 CAS.
- P. Talalay, Hydroxysteroid dehydrogenase.:Colowick, Methods Enzymol., 1962, 512–516 CAS.
- E. Krieger, G. Koraimann and G. Vriend, Increasing the precision of comparative models with YASARA NOVA—a self-parameterizing force field, Proteins: Struct., Funct., Bioinf., 2002, 47, 393–402 CrossRef CAS PubMed.
- E. Krieger, T. Darden, S. B. Nabuurs, A. Finkelstein and G. Vriend, Making optimal use of empirical energy functions: force-field parameterization in crystal space, Proteins: Struct., Funct., Bioinf., 2004, 57, 678–683 CrossRef CAS PubMed.
-
R. Parmar, C. N. Patel, H. Highland, K. Desai and L. B. George, Pesticide Target Protein and Phytochemical Interactions- A Computational Study Mitigating Mosquito-Vectors, (Conference paper) India International Science Festival held at IIT, New Delhi, India on 4-8th December, 2015. DOI:10.13140/RG.2.1.2747.2249.
- K. Balan, P. Pratheebaa, T. Jebastin, N. Sundarabaalaji, X. Liu and T. Palvannan, Beneficial protective effects of 2-allyl amino 4-methyl sulfanyl butyric acid on glucose metabolism and glycoprotein components in streptozotocin induced diabetic rats with molecular modeling, Toxicol. Res., 2016, 5, 399–406 RSC.
- M. A. Borad, M. N. Bhoi, S. K. Rathwa, M. S. Vasava, H. D. Patel, C. N. Patel, H. A. Pandya, E. A. Pithawala and J. J. Georrge, Microwave-Assisted ZrSiO2 Catalysed Synthesis, Characterization and Computational Study of Novel Spiro [Indole-Thiazolidines] Derivatives as Anti-tubercular Agents, Interdiscip. Sci.: Comput. Life Sci., 2016, 1–8 CAS.
- G.-B. Li, M. I. Abboud, J. Brem, H. Someya, C. T. Lohans, S.-Y. Yang, J. Spencer, D. W. Wareham, M. A. McDonough and C. J. Schofield, NMR-filtered virtual screening leads to non-metal chelating metallo-β-lactamase inhibitors, Chem. Sci., 2017, 8, 928–937 RSC.
- O. Trott and A. J. Olson, AutoDock Vina: improving the speed and accuracy of docking with a new scoring function, efficient optimization, and multithreading, J. Comput. Chem., 2010, 31, 455–461 CAS.
- A. W. Kahsai, K. Xiao, S. Rajagopal, S. Ahn, A. K. Shukla, J. Sun, T. G. Oas and R. J. Lefkowitz, Multiple ligand-specific conformations of the β2-adrenergic receptor, Nat. Chem. Biol., 2011, 7, 692 CrossRef CAS PubMed.
- E. L. Tobinick, The value of drug repositioning in the current pharmaceutical market, Drug News Perspect., 2009, 22, 119–125 CrossRef PubMed.
- H. J. Berendsen, J. v. Postma, W. F. van Gunsteren, A. DiNola and J. Haak, Molecular dynamics with coupling to an external bath, J. Chem. Phys., 1984, 81, 3684–3690 CrossRef CAS.
- F. S. Vom Saal, B. T. Akingbemi, S. M. Belcher, L. S. Birnbaum, D. A. Crain, M. Eriksen, F. Farabollini, L. J. Guillette Jr., R. Hauser and J. J. Heindel, Chapel Hill bisphenol A expert panel consensus statement: integration of mechanisms, effects in animals and potential to impact human health at current levels of exposure, Reprod. Toxicol., 2007, 24, 131 CrossRef CAS PubMed.
- M. Ruiz, M. Fernández, Y. Pico, J. Manes, M. Asensi, C. Carda, G. Asensio and J. Estrela, Dietary administration of high doses of pterostilbene and quercetin to mice is not toxic, J. Agric. Food Chem., 2009, 57, 3180–3186 CrossRef CAS PubMed.
- J. Zheng, J. Wu, J. Chen, J. Liu, Y. Lu, C. Huang, G. Hu, X. Wang and Y. Zeng, Therapeutic effects of quercetin on early inflammation in hypertriglyceridemia-related acute pancreatitis and its mechanism, Pancreatology, 2016, 16, 200–210 CrossRef CAS PubMed.
- H. Chen, C. Lu, H. Liu, M. Wang, H. Zhao, Y. Yan and L. Han, Quercetin ameliorates imiquimod-induced psoriasis-like skin inflammation in mice via the NF-κB pathway, Int. Immunopharmacol., 2017, 48, 110–117 CrossRef CAS PubMed.
- M. L. Dufau, A. Khanum, C. A. Winters and T.-M. Chon-Hwa, Multistep regulation of Leydig cell function, J. Steroid Biochem., 1987, 27, 343–350 CrossRef CAS PubMed.
- S. Jahan, Q. U. Ain and H. Ullah, Therapeutic effects of quercetin against bisphenol A induced testicular damage in male Sprague Dawley rats, Syst. Biol. Reprod. Med., 2016, 62, 114–124 CrossRef CAS PubMed.
- J. D. Meeker, A. M. Calafat and R. Hauser, Urinary bisphenol A concentrations in relation to serum
thyroid and reproductive hormone levels in men from an infertility clinic, Environ. Sci. Technol., 2009, 44, 1458–1463 CrossRef PubMed.
- S. Watanabe, W. Rui-Sheng, M. Miyagawa, K. Kobayashi, S. Megumi, S. Sekiguchi and T. Honma, Imbalance of testosterone level in male offspring of rats perinatally exposed to bisphenol A, Ind. Health, 2003, 41, 338–341 CrossRef CAS PubMed.
- J. P. Wiebe, Steroidogenesis in Rat Leydig Cells: Changes in Activity of 5-ane and 5-ene 3β-Hydroxysteroid Dehydrogenases During Sexual Maturation 1, Endocrinology, 1976, 98, 505–513 CrossRef CAS PubMed.
- Y. Toyama and S. Yuasa, Effects of neonatal administration of 17β-estradiol, β-estradiol 3-benzoate, or bisphenol A on mouse and rat spermatogenesis, Reprod. Toxicol., 2004, 19, 181–188 CrossRef CAS PubMed.
- M. Sakaue, S. Ohsako, R. Ishimura, S. Kurosawa, M. Kurohmaru, Y. Hayashi, A. Yasunobu, J. Yonemoto and C. Tohyama, Bisphenol-A affects spermatogenesis in the adult rat even at a low dose, J. Occup. Health, 2001, 43, 185–190 CrossRef CAS.
-
S. Samova, H. Doctor and R. Verma, Spermatotoxic effect of bisphenol a and its amelioration using quercetin, 2016 Search PubMed.
Footnote |
† Electronic supplementary information (ESI) available. See DOI: 10.1039/c7tx00161d |
|
This journal is © The Royal Society of Chemistry 2018 |