A highly rigid and gas selective methanopentacene-based polymer of intrinsic microporosity derived from Tröger's base polymerization†
Received
16th January 2018
, Accepted 27th February 2018
First published on 13th March 2018
Abstract
Polymers of intrinsic microporosity (PIMs) have been identified as potential next generation membrane materials for the separation of gas mixtures of industrial and environmental relevance. Based on the exceptionally rigid methanopentacene (MP) structural unit, a Polymer of Intrinsic Microporosity (PIM-MP-TB) was designed to demonstrate high selectivity for gas separations. PIM-MP-TB was prepared using a polymerisation reaction involving the formation of Tröger's base linking groups and demonstrated an apparent BET surface area of 743 m2 g−1 as a powder. The microporosity of PIM-MP-TB was also characterized by chain packing simulations. PIM-MP-TB proved soluble in chlorinated solvents and was cast as a robust, free-standing film suitable for gas permeation measurements. Despite lower gas permeability as compared to previously reported PIMs, high selectivities for industrially relevant gas pairs were obtained, surpassing the 2008 Robeson upper bound for H2/CH4 and O2/N2, (e.g., PO2 = 999 Barrer; αO2/N2 = 5.0) and demonstrating a clear link between polymer rigidity and selectivity. Upon aging, the permeability data move parallel to the Robeson upper bounds with a decrease of permeability, compensated by a related increase in selectivity. Mixed gas permeation measurement for CO2/CH4 and CO2/N2 mixtures confirmed the excellent selectivity of PIM-MP-TB for potentially relevant separations such as biogas upgrading and CO2 capture from flue gas. Importantly, unlike other high performing PIMs, PIM-MP-TB is prepared in four simple steps from a cheap starting material.
1. Introduction
Since Polymers of Intrinsic Microporosity (PIMs) were first reported in 2004,1 a range of potential applications have been identified including catalysis,2–5 chiral separations,6 and as the active material in sensors7,8 or ionic diodes.9,10 In particular, PIMs with the ability to form flexible, robust, self-standing films have potential as membrane materials for anionic transport,11 the separation of gases, vapours12 or for nanofiltration.13,14 Their exceptional potential for gas separation15,16 is based on the high free volume generated from the inability of their contorted polymer chains to pack efficiently, which provides high permeability, while the lack of conformational freedom and rigidity, due to their fused-ring structures, ensures good permselectivity. The design concept of PIMs has produced polymers whose gas permeability data have redefined the Robeson upper bounds,17–20 which quantify the state-of-the-art trade-off between the desirable properties of permeability and selectivity. Hence, PIMs have potential for various industrial gas separation applications such as the recovery of hydrogen (e.g., H2/N2), oxygen- or nitrogen-enrichment of air (O2/N2), biomethane and natural gas purification (CO2/CH4) and carbon capture (CO2/N2). Previously, we and others21,22 have demonstrated the benefit of using bridged bicyclic units in place of the less rigid spirobisindane and benzodioxin structures of conventional PIMs (e.g. PIM-1).23 For example, PIM-EA-TB (Fig. 1a),23 which is composed of ethanoanthracene (EA) structural units linked by Tröger's base (TB i.e. methanodiazocene) demonstrates exceptional selectivity and subsequent work showed that the replacement of EA with triptycene to give PIM-Trip-TB24 (Fig. 1b) or benzotriptycene to give PIM-BTrip-TB25 (Fig. 1c) improves further both gas selectivity and permeability.
 |
| Fig. 1 PIMs based on TB linkages between (a) ethanoanthracene (b) triptycene or (c) benzotriptycene structural units.23–25 | |
Evaluation of a range of potential structural building units for PIMs, assisted by molecular modeling, suggested two adjustments that could be made to the bridged bicyclic structural unit that might enhance further the rigidity of a TB-based PIM. Firstly, the use of a shorter methylene bridge and secondly, the incorporation of a greater proportion of aromatic rings within the polymer chain.26 The readily prepared 6,13-dihydro-6,13-methanopentacene (MP)27 hydrocarbon satisfied both of these design criteria (Fig. 2).28
 |
| Fig. 2 (a) The structure of methanopentacene (MP); (b) XRD solid state crystal structure of MP.27 | |
2. Results and discussion
2.1. Polymer synthesis and characterization
Unsubstituted methanopentacene is easily prepared by the double Diels–Alder addition of the diene, dibromoquinodimethane, to norbornadiene (Scheme 1).28 The required diene precursor to dinitromethanopentacene, 1,2-bis(dibromomethyl)-4-nitrobenzene (2), was synthesised by a convenient literature procedure in which a dilute solution of bromine is added slowly to the cheaply available 4-nitro-o-xylene (1) in chlorobenzene, whilst irradiating the reaction with a tungsten lamp.29 This method avoids the use of highly toxic solvents such as carbon tetrachloride, and the high atom-inefficiency associated with brominating agents such as N-bromosuccinimide, reported in other procedures.30,31 Treatment of 2 with sodium iodide eliminates bromine to produce the reactive intermediate dibromoquinodimethane, which combines with the commercial norbornadiene 3, to give an isomeric mixture of 2,9(10)-dinitro-6,13-dihydro-6,13-methanopentacene (4) in 50% yield. This was reduced in the presence of hydrazine and RANEY® nickel, to obtain the required 2,9(10)-diamino-6,13-dihydro-6,13-methanopentacene monomer (5) as a mixture of isomers. The standard TB polymerisation procedure23 was followed to afford PIM-MP-TB in 50% yield after purification by reprecipitation, which is similar to other TB-based PIMs.
 |
| Scheme 1 Synthetic route to PIM-MP-TB (DMM = dimethoxymethane; TFA = trifluoroacetic acid). | |
It is well established that TB formation using 2-aminonaphthalene takes place exclusively at the 1-position of the naphthyl ring32 so that the expected ideal structure of PIM-MP-TB is that shown in Scheme 1, albeit with no control over regiochemistry due to the mixture of two isomers of the monomer and the relative orientations of methylene bridges of the MP building unit and TB linking group (Fig. 3).
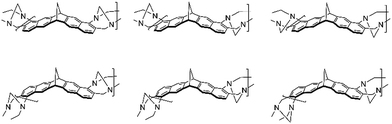 |
| Fig. 3 The regiochemistry within the repeat unit of PIM-MP-TB due to the isomeric mixture of the monomer and the relative orientations of methylene bridges of the MP building unit and TB linking group. | |
Isothermal nitrogen adsorption confirmed the polymer to be significantly microporous with a BET surface area (SABET) of 743 m2 g−1. This is somewhat lower than the values obtained for PIM-EA-TB (1030 m2 g−1)23 and other TB PIMs.24–26,33 Thermogravimetric analysis (Fig. ESI3†) shows that the polymer is stable up to 400 °C (<3% loss), much higher than the EA–TB analogue, which undergoes a reverse Diels–Alder reaction23 to release an ethylene fragment at around 260 °C.
2.2. Simulation of molecular structure and chain packing
To assess the relative flexibility of the PIM-MP-TB, the mechanical deformation of the MP structural unit was compared with that of triptycene,24 ethanoanthracene23 and TB. Fig. 4a shows the comparison of the energy cost as the dihedral angles in these units angle deviate from their most stable configuration, corresponding to the energy minimum as calculated using the Spartan 10.0 modelling package (Fig. 4b). The energy profiles for the framework distortion about dihedral angle 1 (Fig. 4a) are very similar for each structural unit (Fig. 4c). In contrast, those for dihedral angles 2 and 3 are sharper for MP than for the other units, confirming its greater rigidity (Table ESI1†). Simulation of chain packing for PIM-MP-TB was performed as described previously for PIM-EA-TB (ESI Section 6†) and optimized to a polymer density of 1.095 g cm−3 (Fig. 5a). This is only a small deviation from the experimental density of 1.13 g cm−3 for a freshly prepared film is 3.7% and hence is in reasonable agreement. The experimental density is higher than that reported for PIM-1 (1.092 g cm−3)34 and of PIM-EA-TB (1.08 g cm−3).35 Free volume as determined by Bondi's method was calculated to be 25.8 ± 0.5% which is slightly smaller than that of PIM-EA-TB (27.6%)35 and of PIM-1 (28.8%).36 The calculated fractional free volume confirms the poor polymer packing that is typical for all PIMs. The dihedral angle distribution for the MP structural unit observed within the packing simulation correlate well with the energy potential of deformation calculated by molecular dynamics (Fig. 5). The “bite” angle of the MP units in the optimized chains of PIM-MP-TB (Fig. 5b), shows a very wide distribution (Fig. 5c). The average angle is close to the value found for the XRD solid state crystal structure in Fig. 2, but the wide distribution shows that many monomeric units are either stretched or squeezed into a configuration far from their thermodynamic equilibrium. Similar deformations of structural units within packing models of PIMs have been observed previously for PIM-EA-TB and PIM-1.34,37,38
 |
| Fig. 4 (a) The defined dihedral angles in methanopentacene (MP), triptycene (Trip), ethanoanthracene (EA) and TB units; (b) potential energy surfaces of dihedral angles (DA) 1, 2 and 3 for MP, EA, triptycene and TB. (c) Dihedral angle distribution for the MP (red) and TB (black) units derived from packing simulations model. | |
 |
| Fig. 5 (a) Molecular model of the polymer structure with a calculated density of 1.095 g cm−3. The free volume elements are indicated as blue bubbly shapes. Length of the box edges is about 32 Å. (b, c) Structure and “bite” angle distribution of the MP unit in the optimized polymer chains. | |
Fig. 5a shows a 3D representation of the free volume elements and their interconnectivity. Modelling of the pore size distribution by the method proposed by Hofmann and Heuchel39 shows the presence of a majority of pores with an equivalent sphere radius in the range of 1–5 Å (i.e. pore diameter = 2–10 Å) (Fig. 6). The free volume element size distribution was quantified by the Rmax and Vconnect approach, setting the probe diameter to the size of an o-positronium particle (r = 1.1 Å), carbon dioxide (r = 1.65 Å), oxygen (r = 1.73 Å) and nitrogen (r = 1.82 Å), and using a grid spacing of 0.5 Å. The maximum equivalent sphere radius in PIM-MP-TB is approximately 7 Å, which is smaller than for other other PIMs, such as PIM-1 (16.5–17 Å)34 and PIM-EA-TB (15.5 Å).35 This suggests that pores in PIM-MP-TB are both smaller and less interconnected relative to those in PIM-1 or PIM-EA-TB but similar to, for example, Hyflon®AD.40
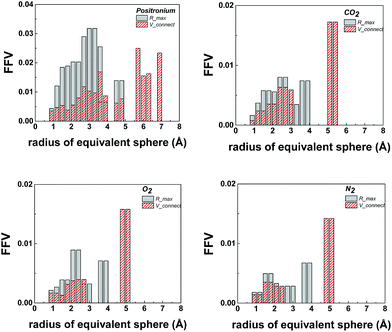 |
| Fig. 6 Averaged size distribution functions using O-positronium (r = 1.1 Å), carbon dioxide (r = 1.65 Å), oxygen (r = 1.73 Å) and nitrogen (r = 1.82 Å) as probes using the Hofmann–Heuchel method.39 | |
The pore size distribution was calculated via NLDFT and H–K analysis of the CO2 uptake at 273 K (Fig. ESI1†). Fig. 6 shows the pore size distribution of PIM-MP-TB. Qualitatively, PIM-MP-TB has a lower total pore volume and fewer pores over 5 Å, compared to PIM-EA-TB,35 which correlates with its lower BET surface area and pore volume measured by gas adsorption.
2.3. Pure gas transport
Before permeability measurements, the films were soaked in methanol and dried to remove traces of residual casting solvent and to reverse the effect of physical aging.37,38,41 The gas permeability, diffusivity and solubility coefficients, with the respective ideal selectivity, for the methanol-treated, aged and thermally treated films of PIM-MP-TB are given in Table 1. The precise sample history is given in Table ESI4.† The data are compared with those of the archetypal PIM-1 and PIM-EA-TB.
Table 1 Gas permeabilities Px, diffusion coefficients Dx, solubility coefficients Sx, and ideal selectivities α (Px/Py), α (Dx/Dy) and α (Sx/Sy) at 25 °C for a methanol-treated film of PIM-MP-TB, PIM-EA-TB and PIM-1 with comparable thickness (94 μm, 95 μm and 93 μm, respectively) and for the aged and thermally treated samples of PIM-MP-TB
Polymer (statea) |
Transport parameter |
Permeability, diffusivity and solubility |
Selectivity α (Px/Py) α (Dx/Dy) α (Sx/Sy) |
N2 |
O2 |
CO2 |
CH4 |
H2 |
He |
H2/N2 |
H2/CH4 |
CO2/N2 |
O2/N2 |
CO2/CH4 |
State: (a) methanol treated; (b) day 1 after thermal conditioning under vacuum at 140 °C; (c) after aging of 118 days and intermediate mixed gas permeation; (d) after 370 days of aging and intermediate mixed gas permeation. See ESI Table 4 for the complete sample history.
|
PIM-MP-TB (a) |
P
x
[Barrer] |
200 |
999 |
3500 |
264 |
4050 |
1310 |
20.3 |
15.3 |
17.5 |
5.0 |
13.3 |
D
x
[10−12 m2 s−1] |
18.3 |
106 |
26 |
6 |
2870 |
4640 |
157 |
478 |
1.42 |
5.8 |
4.33 |
S
x
[cm3(STP) cm−3 bar−1] |
8.21 |
7.08 |
101 |
31 |
1.06 |
0.21 |
0.13 |
0.03 |
12.3 |
0.86 |
3.26 |
PIM-MP-TB (b) |
P
x
[Barrer] |
125 |
648 |
2340 |
158 |
2790 |
956 |
22.3 |
17.7 |
18.7 |
5.18 |
14.8 |
PIM-MP-TB (c) |
P
x
[Barrer] |
21.4 |
134 |
633 |
26 |
1120 |
492 |
52.3 |
43 |
29.6 |
6.26 |
24.4 |
PIM-MP-TB (d) |
P
x
[Barrer] |
13.3 |
88.4 |
378 |
14.7 |
812 |
368 |
61 |
55.3 |
28.4 |
6.65 |
25.7 |
PIM-EA-TB (a) |
P
x
[Barrer] |
380 |
1630 |
5100 |
572 |
7310 |
2720 |
19.2 |
12.8 |
13.4 |
4.29 |
8.92 |
D
x
[10−12 m2 s−1] |
40.5 |
177 |
41 |
12 |
5000 |
6000 |
123 |
419 |
1.01 |
4.37 |
3.42 |
S
x
[cm3(STP) cm−3 bar−1] |
7.04 |
6.91 |
93.3 |
35.75 |
1.10 |
0.34 |
0.16 |
0.03 |
13.2 |
0.98 |
2.61 |
PIM-1 (a) |
P
x
[Barrer] |
773 |
2140 |
12 800 |
1280 |
4710 |
1830 |
6.1 |
3.68 |
16.6 |
2.77 |
10 |
D
x
[10−12 m2 s−1] |
165 |
452 |
199 |
70 |
5760 |
7120 |
35 |
82 |
1.21 |
2.74 |
2.84 |
S
x
[cm3(STP) cm−3 bar−1] |
3.52 |
3.54 |
48 |
13.7 |
0.61 |
0.19 |
0.17 |
0.04 |
13.6 |
1 |
3.5 |
The order of permeability for the PIM-MP-TB is H2 > CO2 > He > O2 > CH4 > N2, similar to that of PIM-EA-TB12 but different to that of more permeable PIMs (PIM-Trip-TB and PIM-BTrip-TB),24,25 in which CO2 has a higher permeability than H2, and O2 has a higher permeability than He. This behavior indicates greater size selectivity for PIM-MP-TB as demonstrated by very high diffusivity selectivity (Dx/Dy) with respect to PIM-1, PIM-EA-TB and PIM-Trip-TB (Table 1). The high size selectivity is best seen from the very steep correlation between the diffusion coefficient and the effective diameter of the gas (Fig. 8). The excellent performance of PIM-MP-TB can be attributed to the superior rigidity of the MP bridged bicyclic unit, as discussed above. The high permselectivity, compared with that of many other PIMs, combined with moderately high permeabilities, places it close to the upper bound for the CO2/CH4 and CO2/N2 gas pair (Fig. 7a and c) and well above the 2008 upper bound for gas pairs involving H2 (PH2 = 4050 Barrer) with H2/CH4; α = 15.3 (Fig. 7b) and H2/N2 (α = 20.3). Furthermore, PIM-MP-TB demonstrate a high oxygen permeability (PO2 = 999 Barrer) showing higher O2/N2 selectivity (α = 5.0) with respect to PIM-EA-TB (α = 4.3), which is close to the revised 2015 upper bound19 (Fig. 7d). The high O2/N2 selectivity confirms that the separation is strongly dependent on a molecular sieving mechanism, as O2 has a smaller kinetic diameter (3.46 Å for O2 and 3.64 Å for N2) whereas both gases have a similar solubility in glassy polymers. Fig. 7 also demonstrates the effect of physical aging of PIM-MP-TB after different treatments. Upon ageing, the data points move almost parallel to the Robeson upper bound, in the direction of higher selectivity and lower permeability compared to the methanol treated membrane. The thermal conditioning at 140 °C for 24 h just after methanol treatment (1 day aged), produces a similar effect suggesting that accelerated aging was achieved. After one year of ageing, with intermediate mixed gas permeation measurements, the performance remains still close to the 2008 Robeson upper bound for the CO2/CH4 and CO2/N2 separation, and above the upper bound for the H2/CH4, H2/N2 and O2/N2 gas pairs.
 |
| Fig. 7 Robeson plots of PIM-MP-TB for the CO2/CH4, H2/CH4, CO2/N2 and O2/N2 gas pairs after methanol treatment ( ), aged for 1 ( ), 118 ( ), 370 ( ) days after the thermal treatment at 140 °C under vacuum. Mixed gas data after 110 days aged ( ). The data for PIM-1 ( ) and recent TB-PIMs like PIM-EA-TB ( ) and PIM-Trip-TB ( ) after MeOH are given for comparison. Feed pressure 1 bar and temperature 25 °C. The total aging time in days for each point is indicated as “xxx d”. | |
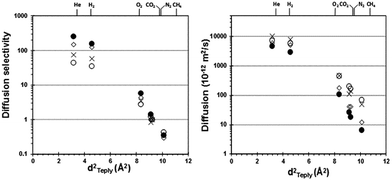 |
| Fig. 8 Diffusion coefficients and corresponding selectivities vs. nitrogen as function of the squared effective diameter of the penetrant42 gases for PIM-MP-TB (●), PIM-1 (○), PIM-EA-TB (◊) and PIM-Trip-TB (×).25,35,37 Feed pressure 1 bar and temperature 25 °C. | |
2.4. Mixed gas permeation
PIM-MP-TB also demonstrates promising permselectivity for mixed gas separation. Mixed gas permeability measurements were carried out using a sample aged for 110 days. Two different binary gas mixtures were used: CO2/CH4 (52.1/47.9 mol%) and CO2/N2 (10/90 mol%), simulating biogas with high CO2 content and CO2 poor flue gas, respectively. During the measurement, the pressure was increased stepwise from 1 to 6 bar (a) at 25 °C. Fig. 9 shows that for the CO2/CH4 mixture the CO2 permeability is virtually independent from the pressure, whereas the CH4 permeability slightly increases and, subsequently, the CO2/CH4 selectivity decreases with pressure. This suggests that the CO2 induces matrix swelling, thus, facilitating CH4 permeation. For the CO2/N2 mixture, both CO2 and N2 permeability slightly decrease as a function of the pressure and the selectivity remains nearly constant. Thus, PIM-MP-TB maintains excellent gas transport properties under mixed gas conditions for the CO2/N2 gas pair, close to the ideal selectivity (α = 29.6).
 |
| Fig. 9 (a) Pressure dependence of the CO2 and CH4 permeabilities with the corresponding CO2/CH4 selectivity, using the binary mixture CO2/CH4 (52.1 : 47.9 vol%); (b) pressure dependence of the CO2 and N2 permeabilities with the corresponding CO2/N2 selectivity, using the binary mixture CO2/N2 (10 : 90 vol%) 110 days aged PIM-MP-TB. Measurement temperature 25 °C. | |
3. Conclusions
The MP structural unit proved useful for making a high performing PIM. PIM-MP-TB demonstrated high selectivities for several important gas pairs such as O2/N2, H2/N2 and H2/CH4, well above the 2008 Robeson upper bounds and approach commercially useful separation factors for the aged film. The CO2/N2 ideal selectivity (α = 17.5) is maintained under mixed gas conditions (10% CO2 in N2), up to 6 bar, which offers good potential for the application of PIM-MP-TB as a high flux first pass membrane in a two membrane system for CO2 capture from flue gas. These results provide further evidence that molecular rigidity is a major determinant of intrinsic microporosity and high size selectivity. The molecular sieving performance, combined with the pore size distribution, suggests that an optimum distribution of small uniform micropores is achieved by maximising chain rigidity and internal free volume of the constituent monomer units. Molecular modelling confirms the high chain rigidity and shows that PIM-MP-TB has fewer interconnected pores than other common PIMs, which explains its relatively low permeability in combination with a strongly size sieving nature. Most importantly, gas permeability data of PIM-MP-TB approach those obtained from the triptycene-based PIMs KAUST-PI-1,22 TPIM-1
21 and PIM-Trip-TB,24 which have been used to define the 2015 upper bounds for the H2/CH4, H2/N2 and O2/N2 gas pairs.19 However, in contrast to these high performing PIMs, the synthesis of PIM-MP-TB is achieved in only four simple steps from a cheap starting material.
Conflicts of interest
There are no conflicts to declare.
Acknowledgements
The work leading to these results has received funding from the European Union's Seventh Framework Program (FP7/2007–2013) under grant agreement no. 608490, project M4CO2. Alessio Fuoco and Marcello Monteleone are gratefully acknowledged for their help in some of the gas permeation experiments.
Notes and references
- P. M. Budd, B. S. Ghanem, S. Makhseed, N. B. McKeown, K. J. Msayib and C. E. Tattershall, Chem. Commun., 2004, 230–231 RSC
.
- F. Xia, M. Pan, S. Mu, R. Malpass-Evans, M. Carta, N. B. McKeown, G. A. Attard, A. Brew, D. J. Morgan and F. Marken, Electrochim. Acta, 2014, 128, 3–9 CrossRef CAS
.
- X. Du, Y. Sun, B. Tan, Q. Teng, X. Yao, C. Su and W. Wang, Chem. Commun., 2010, 46, 970–972 RSC
.
- P. Kaur, J. T. Hupp and S. T. Nguyen, ACS Catal., 2011, 1, 819–835 CrossRef CAS
.
- M. Carta, M. Croad, K. Bugler, K. J. Msayib and N. B. McKeown, Polym. Chem., 2014, 5, 5262–5266 RSC
.
- X. Weng, J. E. Baez, M. Khiterer, M. Y. Hoe, Z. Bao and K. J. Shea, Angew. Chem., Int. Ed., 2015, 54, 11214–11218 CrossRef CAS PubMed
.
- Y. Wang, N. B. McKeown, K. J. Msayib, G. A. Turnbull and I. D. W. Samuel, Sensors, 2011, 11, 2478–2487 CrossRef CAS PubMed
.
- N. A. Rakow, M. S. Wendland, J. E. Trend, R. J. Poirier, D. M. Paolucci, S. P. Maki, C. S. Lyons and M. J. Swierczek, Langmuir, 2010, 26, 3767–3770 CrossRef CAS PubMed
.
- E. Madrid, Y. Rong, M. Carta, N. B. McKeown, R. Malpass-Evans, G. A. Attard, T. J. Clarke, S. H. Taylor, Y. T. Long and F. Marken, Angew. Chem., Int. Ed., 2014, 53, 10751–10754 CrossRef CAS PubMed
.
- Y. Rong, A. Kolodziej, E. Madrid, M. Carta, R. Malpass-Evans, N. B. McKeown and F. Marken, J. Electroanal. Chem., 2016, 779, 241–249 CrossRef CAS
.
- Z. Yang, R. Guo, R. Malpass-Evans, M. Carta, N. B. McKeown, M. D. Guiver, L. Wu and T. Xu, Angew. Chem., 2016, 128, 11671–11674 CrossRef
.
- M. Žák, M. Klepic, L. Č. Štastná, Z. Sedláková, H. Vychodilová, Š. Hovorka, K. Friess, A. Randová, L. Brožová and J. C. Jansen, Sep. Purif. Technol., 2015, 151, 108–114 CrossRef
.
- T. S. Anokhina, A. A. Yushkin, P. M. Budd and A. V. Volkov, Sep. Purif. Technol., 2015, 156, 683–690 CrossRef CAS
.
- D. Fritsch, P. Merten, K. Heinrich, M. Lazar and M. Priske, J. Membr. Sci., 2012, 401–402, 222–231 CrossRef CAS
.
- N. B. McKeown and P. M. Budd, Macromolecules, 2010, 43, 5163–5176 CrossRef CAS
.
- P. M. Budd and N. B. McKeown, Polym. Chem., 2010, 1, 63–68 RSC
.
- L. M. Robeson, J. Membr. Sci., 1991, 62, 165–185 CrossRef CAS
.
- L. M. Robeson, J. Membr. Sci., 2008, 320, 390–400 CrossRef CAS
.
- R. Swaidan, B. Ghanem and I. Pinnau, ACS Macro Lett., 2015, 4, 947–951 CrossRef CAS
.
- L. M. Robeson, Q. Liu, B. D. Freeman and D. R. Paul, J. Membr. Sci., 2015, 476, 421–431 CrossRef CAS
.
- B. S. Ghanem, R. Swaidan, X. Ma, E. Litwiller and I. Pinnau, Adv. Mater., 2014, 26, 6696–6700 CrossRef CAS PubMed
.
- B. S. Ghanem, R. Swaidan, E. Litwiller and I. Pinnau, Adv. Mater., 2014, 26, 3688–3692 CrossRef CAS PubMed
.
- M. Carta, R. Malpass-Evans, M. Croad, Y. Rogan, J. C. Jansen, P. Bernardo, F. Bazzarelli and N. B. McKeown, Science, 2013, 339, 303–307 CrossRef CAS PubMed
.
- M. Carta, M. Croad, R. Malpass-Evans, J. C. Jansen, P. Bernardo, G. Clarizia, K. Friess, M. Lanč and N. B. McKeown, Adv. Mater., 2014, 26, 3526–3531 CrossRef CAS PubMed
.
- I. Rose, M. Carta, R. Malpass-Evans, M.-C. Ferrari, P. Bernardo, G. Clarizia, J. C. Jansen and N. B. McKeown, ACS Macro Lett., 2015, 4, 912–915 CrossRef CAS
.
- I. Rose, C. G. Bezzu, M. Carta, B. Comesana-Gandara, E. Lasseuguette, M. C. Ferrari, P. Bernardo, G. Clarizia, A. Fuoco, J. C. Jansen, K. E. Hart, T. P. Liyana-Arachchi, C. M. Colina and N. B. McKeown, Nat. Mater., 2017, 16, 932–937 CrossRef CAS PubMed
.
- H.-Y. Tsai, M.-H. Luo, T.-C. Fang, M.-J. Chang and K.-Y. Chen, J. Chin. Chem. Soc., 2013, 60, 166–170 CrossRef CAS
.
- M. N. Paddon-Row and H. K. Patney, Synthesis, 1986, 1986, 328–330 CrossRef
.
- K. Baathulaa, Y. Xu and X. Qian, Nat. Protoc., 2011, 6, 1990–1997 CrossRef CAS PubMed
.
- F.-G. Klaerner, S. Madenci, M. C. Kuchenbrandt, D. Blaeser, R. Boese, G. Fukuhara and Y. Inoue, Eur. J. Org. Chem., 2012, 2012, 3385–3395 CrossRef CAS
.
- Y. Shu, Y.-F. Lim, Z. Li, B. Purushothaman, R. Hallani, J. E. Kim, S. R. Parkin, G. G. Malliaras and J. E. Anthony, Chem. Sci., 2011, 2, 363–368 RSC
.
- R. G. Kostyanovsky, V. R. Kostyanovsky, G. n. K. Kadorkina and K. A. Lyssenko, Mendeleev Commun., 2003, 13, 111–113 CrossRef
.
- M. Carta, R. Malpass-Evans, M. Croad, Y. Rogan, M. Lee, I. Rose and N. B. McKeown, Polym. Chem., 2014, 5, 5267–5272 RSC
.
- M. Heuchel, D. Fritsch, P. M. Budd, N. B. McKeown and D. Hofmann, J. Membr. Sci., 2008, 318, 84–99 CrossRef CAS
.
- E. Tocci, L. De Lorenzo, P. Bernardo, G. Clarizia, F. Bazzarelli, N. B. McKeown, M. Carta, R. Malpass-Evans, K. Friess, K. Pilnacek, M. Lanc, Y. P. Yampolskii, L. Strarannikova, V. Shantarovich, M. Mauri and J. C. Jansen, Macromolecules, 2014, 47, 7900–7916 CrossRef CAS
.
- W. Fang, L. Zhang and J. Jiang, J. Phys. Chem. C, 2011, 115, 14123–14130 CAS
.
- P. M. Budd, N. B. McKeown, B. S. Ghanem, K. J. Msayib, D. Fritsch, L. Starannikova, N. Belov, O. Sanfirova, Y. Yampolskii and V. Shantarovich, J. Membr. Sci., 2008, 325, 851–860 CrossRef CAS
.
- P. Bernardo, F. Bazzarelli, F. Tasselli, G. Clarizia, C. R. Mason, L. Maynard-Atem, P. M. Budd, M. Lanč, K. Pilnáček and O. Vopička, Polymer, 2017, 113, 283–294 CrossRef CAS
.
- D. Hofmann, M. Heuchel, Y. Yampolskii, V. Khotimskii and V. Shantarovich, Macromolecules, 2002, 35, 2129–2140 CrossRef CAS
.
- J. C. Jansen, M. Macchione, E. Tocci, L. De Lorenzo, Y. P. Yampolskii, O. Sanfirova, V. P. Shantarovich, M. Heuchel, D. Hofmann and E. Drioli, Macromolecules, 2009, 42, 7589–7604 CrossRef CAS
.
- K. Nagai, A. Higuchi and T. Nakagawa, J. Polym. Sci., Part B: Polym. Phys., 1995, 33, 289–298 CrossRef CAS
.
- V. Teplyakov and P. Meares, Gas Sep. Purif., 1990, 4, 66–74 CrossRef CAS
.
Footnote |
† Electronic supplementary information (ESI) available. See DOI: 10.1039/c8ta00509e |
|
This journal is © The Royal Society of Chemistry 2018 |