Fluoroalkyl ether-diluted dimethyl carbonate-based electrolyte solutions for high-voltage operation of LiNi0.5Co0.2Mn0.3O2 electrodes in lithium ion batteries†
Received
21st January 2018
, Accepted 22nd March 2018
First published on 29th March 2018
Abstract
The energy density of lithium-ion batteries can be increased by improving the battery voltage and/or specific capacity. However, conventional electrolyte solutions decompose oxidatively at high voltages. The stability against oxidation of electrolyte solutions could be enhanced by increasing the concentration of lithium salts. The nearly saturated 8.67 mol kg−1 LiBF4/dimethyl carbonate (DMC) electrolyte solution enabled high-voltage operation of LiNi0.5Co0.2Mn0.3O2 positive-electrodes at 4.6 V, and extended the discharge capacity to ca. 200 mA h g−1. BF4− anions, as well as DMC solvents, were stabilized at the high concentration. To reduce the viscosity and Li concentration without losing the high stability against oxidation toward practical use, the highly concentrated electrolyte solution was diluted with fluorinated co-solvents having a low donor ability. Raman spectroscopy revealed that the solvation structure of LiBF4/DMC was maintained after dilution with a specific fluoroalkyl ether. The resultant low-viscosity and -concentration electrolyte solution was highly stable against oxidation at the LiNi0.5Co0.2Mn0.3O2 electrodes as long as the DMC/LiBF4 molar ratio was kept low. The charge/discharge performance was much better than that for a conventional 1 M LiPF6/ethylene carbonate-based electrolyte solution and the energy density far exceeded that of 5 V class LiNi0.5Mn1.5O4 electrodes.
Introduction
Lithium-ion batteries (LIBs) have greatly expanded their use since 1991 due to their high energy densities. Their performance still needs to be improved with regard to energy density, battery cycle life, rate capability, and safety to realize a smart community with highly efficient energy utilization. The energy density of LIBs can be increased by improving the battery voltage and/or specific capacity. Conventional positive-electrode materials used for LIBs, such as LiMn2O4 and LiCoO2, work at potentials of around 4.0 V vs. Li/Li+, and deliver a specific capacity of about 140 mA h g−1.1,2 On the other hand, the working potentials of a graphite negative-electrode are already very low, close to those of lithium metal, and the specific capacity is more than 2.5 times higher than that of conventional positive-electrodes.3,4 Considering these facts, the improvement of positive electrodes, in terms of both working potentials and specific capacity, is particularly required for the performance improvement of LIBs. LiNixMnyCo(1−x−y)O2, having a layered rock salt crystal structure, is one of the most promising active materials because it has considerable potential for delivering a high reversible capacity over 200 mA h g−1 by charging to high potentials above ca. 4.4 V.5–12 However, the reversible capacity noticeably decreased upon repeated charge/discharge cycling in conventional electrolyte solutions used in commercial LIBs, i.e., ca. 1 mol dm−3 (=M) LiPF6 dissolved in a mixture of ethylene carbonate (EC) and low-viscosity linear carbonates, such as dimethyl carbonate (DMC). The capacity fading is caused by cracks in LiNixMnyCo(1−x−y)O2 particles,13,14 the phase transition of LiNixMnyCo(1−x−y)O2 to a spinel and/or rock salt structure,15,16 and the oxidative decomposition of electrolyte solution.17,18 The stability against oxidation of electrolyte solutions would be enhanced by controlling the solvation properties. Highly concentrated electrolyte solutions including solvent-in-salt electrolyte systems are known to offer unique physicochemical properties such as a wide potential window owing to the enhanced stability against reduction and oxidation.19–23 The enhancement of oxidative stability has been particularly noted for ether-24,25 and carbonate ester-based23 concentrated electrolyte solutions so far. A conventional carbonate ester-based 1 M LiPF6/EC + DMC electrolyte solution contains ca. 12 solvent molecules per lithium ion. Since a lithium ion is solvated with 4 solvent molecules in the first solvation shell, the residual 8 molecules are relatively free in the electrolyte solution.26–30 The HOMO energy of a solvating EC molecule is lower than that of a free one,31 and hence the former is expected to be more stable against oxidation than the latter. Therefore, if all EC molecules are bound to lithium ions, i.e., if the EC/Li molar ratio is less than 4, the stability of the electrolyte solution against oxidation should be enhanced. Unfortunately, however, the solubility of LiPF6 in EC is not so high, while propylene carbonate (PC) and γ-butyrolactone (GBL) dissolved a large amount of lithium salts;32 LiPF6/PC, LiBF4/PC and LiBF4/GBL were nearly saturated at 4.45, 7.25 and 4.65 mol kg−1 at room temperature, which have a solvent/Li molar ratio of 2.20, 1.35 and 2.50, respectively.33–36 We demonstrated that the highly concentrated PC-33,34 and GBL-35 based electrolyte solution exhibited high stability against oxidation at LiNi0.5Mn1.5O4 electrodes. However, increasing the concentration resulted in a high viscosity and a cost increase through the use of a large quantity of Li salts. These problems could be solved by diluting the highly concentrated electrolyte solution with appropriate solvents;37–39 we previously reported that 33.3 vol% 1,1,2,2-tetrafluoroethyl-2,2,3,3-tetrafluoropropyl ether (HFE, H(CF2)2CH2OCF2CF2H)-diluted solution, i.e., 2.50 mol kg−1 LiBF4/PC + HFE (2
:
1 by vol), was a reasonable compromise to attain a low viscosity (51.7 mPa s) and a low PC/Li molar ratio (2.39).40 In fact, LiNi0.5Mn1.5O4 positive-electrodes showed a good charge/discharge performance with low irreversible capacities. However, the viscosity and electrolyte concentration are still high, compared to those for the conventional electrolyte solution, and further dilution was not allowed due to the low miscibility of HFE with LiBF4/PC.
In the present work, DMC, which is less viscous than PC, was used as a main solvent for the preparation of highly concentrated electrolyte solutions.23,41 The highly concentrated DMC-based electrolyte solution is resistant not to 5 V class LiNi0.5Mn1.5O4 electrodes,40 but to LiNi0.5Co0.2Mn0.3O2 electrodes which work at lower potentials. Then, two kinds of fluoroalkyl ether were employed as a diluent to reduce the high viscosity and concentration of the LiBF4/DMC. The solvation structure of Li+–DMC in the diluted electrolyte solutions was studied by Raman spectroscopy. The charge and discharge properties of LiNi0.5Co0.2Mn0.3O2 positive-electrodes were measured in the resultant electrolyte solution.
Experimental
Reagents and chemicals
LiBF4 (Kishida Chemical), DMC (Kishida Chemical), HFE (Daikin Industries), and 1,1,2,2-tetrafluoroethyl-2,2,2-trifluoroethyl ether (TFEE, HCF2CF2OCH2CF3, Daikin Industries) were used without further purification. A given amount of LiBF4 was dissolved in DMC to obtain LiBF4/DMC with different concentrations ranging from 0.93 (ca. 1 M) to 8.67 mol kg−1 (nearly saturated), which correspond to DMC/Li molar ratios of 11.8 to 1.28. Solvent mixtures of DMC and HFE or TFEE were prepared at a given volume ratio, and then LiBF4 was dissolved in them at a DMC/Li molar ratio of 3.00. As a result, 1.52 mol kg−1 LiBF4/DMC + HFE (1
:
1), 0.73 mol kg−1 LiBF4/DMC + HFE (1
:
2), and 1.55 mol kg−1 LiBF4/DMC + TFEE (1
:
1) were obtained, which correspond to DMC/Li molar ratios of 3.00, 3.96 and 3.00, respectively. The entire preparation of electrolyte solutions was conducted in an Ar filled glovebox (DBO-1.5KP-DID, Miwa Mfg). 1 mol dm−3 LiPF6/ethylene carbonate (EC) + diethyl carbonate (DEC) (1
:
1 by volume, Kishida Chemical) was used as a reference.
Characterization of electrolyte solution
The viscosities of the electrolyte solutions were measured with an Ubbelohde viscometer at room temperature in the Ar filled glovebox. Raman spectra of the electrolyte solutions were recorded using a spectrometer (LabRAM HR Evolution, Horiba) equipped with a multichannel charge coupled device detector. The spectra were obtained using a 785 nm laser (>100 mW) through an objective lens. The scattered light was collected in a backscattering (180°) geometry for an integration time of 10 s × 30 times at room temperature.
Electrochemical measurements
The charge and discharge properties of LiNi0.5Co0.2Mn0.3O2-composite electrodes (NCM523) were investigated using two-electrode coin-type cells. The active material of LiNi0.5Co0.2Mn0.3O2 powder (Tanaka Chemical) was mixed with a conductor (10 wt%) and poly[(vinylidenefluoride)-co-chlorotrifluoroethylene] (P(VdF-CtFE)) (10 wt%) as a binder in 1-methyl-2-pyrrolidone to form a slurry. The conductor used was acetylene black (Denka) or graphitized Ketjenblack (FD-7001D, Lion Specialty Chemicals). The slurry was spread out onto Al foil, and then dried at 80 °C for more than 18 h under vacuum. The NCM523 sheet was used as a working electrode. The counter electrode was Li foil. Microporous polypropylene film (Celgard® 2400) and a glass filter (GF/D, Whatman®) were used as a separator for EC + DEC- and DMC-based electrolyte solution, respectively. All the test cells were assembled in the Ar filled glovebox with a dew point below −80 °C. Charge and discharge tests (TOSCAT-3100, Toyo System) were performed at 30 °C between 3.0 and 4.4, 4.6 or 4.8 V. A slow charge/discharge rate of C/10, so that each charge and discharge process should theoretically be completed in 10 h, was used in this study to emphasize the difference in the stability of the electrolyte solutions; the current density corresponds to ca. 120 μA cm−2. At the intermissions between charging and discharging, the cells were rested under open circuit conditions for 1 h. The morphological changes of the NCM523 were observed by scanning electron microscopy (SEM) combined with energy dispersive X-ray spectrometry (EDX).
Results and discussion
Characterization of concentrated LiBF4/DMC electrolyte solution
DMC was almost saturated at room temperature with 8.67 mol kg−1 LiBF4, where the DMC/Li molar ratio is 1.28. This value is lower than those for nearly saturated PC-based electrolyte solutions; the PC/Li molar ratios of 4.45 mol kg−1 LiPF6/PC and 7.25 mol kg−1 LiBF4/PC are 2.20 and 1.35, respectively. Thus, a more highly concentrated electrolyte solution was obtained with the use of DMC solvent. In addition, the viscosity of the nearly saturated 8.67 mol kg−1 LiBF4/DMC (310 mPa s) was much lower than those for the nearly saturated 4.3 mol kg−1 LiPF6/PC (706.5 mPa s) and 7.25 mol kg−1 LiBF4/PC (3497 mPa s).33,34
Fig. 1a shows the Raman spectra of LiBF4/DMC electrolyte solutions of different concentrations ranging from 1.11 to 8.67 mol kg−1. A major peak at about 916 cm−1 was seen in the Raman spectrum of 1.11 mol kg−1 LiBF4/DMC (DMC/Li+ molar ratio = 10.0), which is assigned to the O–CH3 stretching vibration of free DMC molecules.42–44 A weak shoulder assigned to DMC molecules solvating lithium-ions was also observed at around 934 cm−1. As the concentration of LiBF4 increased, free DMC (ca. 916 cm−1) gradually decreased while solvating DMC (ca. 933 cm−1) increased instead. The nearly saturated 8.67 mol kg−1 LiBF4/DMC (DMC/Li+ = 1.28) gave a major peak at around 936 cm−1, suggesting that almost all DMC molecules (96%) are bound to lithium ions. The relative area ratios of ca. 916 and 934 cm−1 peaks are summarized in Fig. 1b, together with those of ca. 710 (free PC) and 720 (solvating PC) cm−1 peaks for LiBF4/PC for comparison.34,45 The fraction of free DMC molecules was smaller than that for free PC at a given solvent/Li molar ratio, which indicates that free solvent molecules can be effectively reduced in LiBF4/DMC. Another thing to note here is that the peak assigned to the B–F symmetric stretching vibration band (ca. 766 cm−1) gradually shifted toward higher wavenumbers with an increase in the electrolyte concentration (Fig. 1c); bands at 766, 774, and 783 cm−1 were observed for LiBF4/DMC, indicating an increase in the ionic association of LiBF4 with increasing concentration.46 These peaks were located at somewhat higher wavenumbers than those for the nearly saturated LiBF4/PC and LiBF4/acetonitrile (AN) solutions.34,46 These results suggest that the ionic association of LiBF4 should be further promoted in DMC, compared to PC and AN. DMC (εr ≈ 3) has a much lower relative dielectric constant than PC (εr ≈ 65) and AN (εr ≈ 36), and hence Li+, BF4− and DMC molecules are more likely to form aggregates, which should result in increasing the stability of BF4−, as well as DMC, against oxidation.
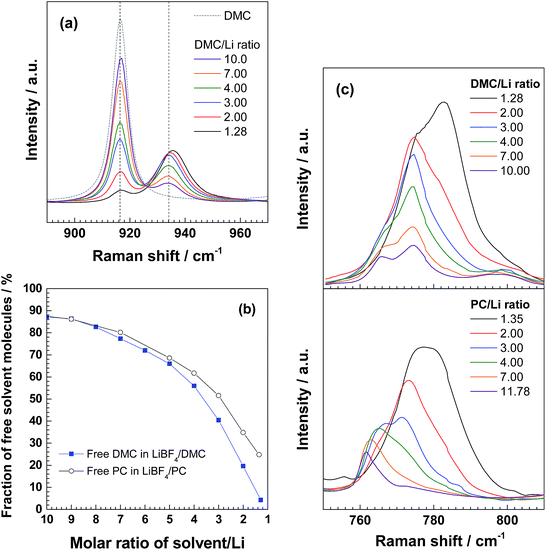 |
| Fig. 1 (a) Raman spectra of DMC and LiBF4/DMC electrolyte solutions with different DMC/Li molar ratios. (b) Relative area ratios (%) of Raman peaks assigned to free DMC at ca. 916 cm−1 and to solvating DMC at ca. 934 cm−1 in (a), and of those assigned to free PC at ca. 710 cm−1 and to solvating PC at ca. 720 cm−1 in Fig. 1 of ref. 34. (c) Raman spectra of LiBF4/DMC and LiBF4/PC with different solvent/Li molar ratios. | |
Effect of the LiBF4 concentration in DMC on the stability against oxidation at NCM523
The charge/discharge properties of NCM523 were preliminarily investigated using a conventional electrolyte solution of 1 M LiPF6/EC + DEC (1
:
1 by volume) in different voltage ranges to determine the targeted voltage range in this study.8 The initial charge/discharge capacity of NCM523 increased as the charging cut-off voltage increased from 4.4 to 4.8 V (Fig. S1a–c in the ESI†), the values of which are summarized in Table S1.† The capacity gradually faded with repeated charge/discharge cycling, and the capacity fading was more severe at higher cut-off voltages.8 As a result, the energy density at the 50th cycle, which was evaluated by integrating the discharge curve, was the highest when charge/discharge cycling was conducted between 3.0 and 4.6 V (Table S1d†); the discharge capacity at the 50th cycle corresponded to 86.9% of the initial capacity. Hence, hereafter, we set the voltage range for charge/discharge measurements to 3.0–4.6 V.
Fig. 2a, b and c show the charge and discharge curves of Li|NCM523 cells using 0.93, 3.70 and 8.67 mol kg−1 LiBF4/DMC, respectively, and the values are summarized in Table 1. The initial discharge capacity of the NCM523 electrode reached 199 mA h g−1 in 0.93 mol kg−1 LiBF4/DMC (DMC/Li+ molar ratio = 11.8). However, the initial irreversible capacity was very high, which resulted in a poor coulombic efficiency (82.6%). These results suggest that irreversible reactions, such as the oxidative decomposition of the electrolyte solution, occurred at high potentials. Increasing the LiBF4 concentration in DMC resulted in a significant reduction of irreversible capacity and an improvement of coulombic efficiency in the 1st cycle (Fig. 2b and c, and Table 1). These results clearly indicate that the oxidative decomposition of electrolyte solution was effectively suppressed by employing the highly concentrated LiBF4/DMC. The high coulombic efficiency in the highly concentrated LiBF4/DMC was maintained over 50 charge/discharge cycles (Fig. 2f). In 3.70 (DMC/Li+ = 3.00) and 8.67 mol kg−1 LiBF4/DMC (DMC/Li+ = 1.28), high discharge capacities greater than 180 mA h g−1 were maintained even at the 50th cycle, which corresponded to ≥90% of the initial discharge capacity. These values were much higher than those obtained for 0.93 mol kg−1 LiBF4/DMC (Table 1) and 1 M LiPF6/EC + DEC (Table S1†), indicating that the cycling performance of NCM523 was greatly improved in the nearly saturated 8.67 mol kg−1 LiBF4/DMC. Fig. 3 shows the SEM/EDX images of NCM523 electrodes after the 50th cycle in 0.93 mol kg−1 and 8.67 mol kg−1 LiBF4/DMC. A number of precipitates containing F were seen on the NCM523 electrode after charge/discharge cycling in 0.93 mol kg−1 LiBF4/DMC (Fig. 3a and b), indicating the oxidative decomposition of BF4− anions. The precipitates would interfere with the lithium ion transfer reaction between NCM523 and electrolyte solution to degrade the charge/discharge performance. On the other hand, the formation of such F-containing precipitates was significantly suppressed in 8.67 mol kg−1 LiBF4/DMC (Fig. 3c and d). These results clearly indicate that BF4− anions are more stable against oxidation in the highly concentrated electrolyte solution, which is consistent with the Raman spectra in Fig. 1c.
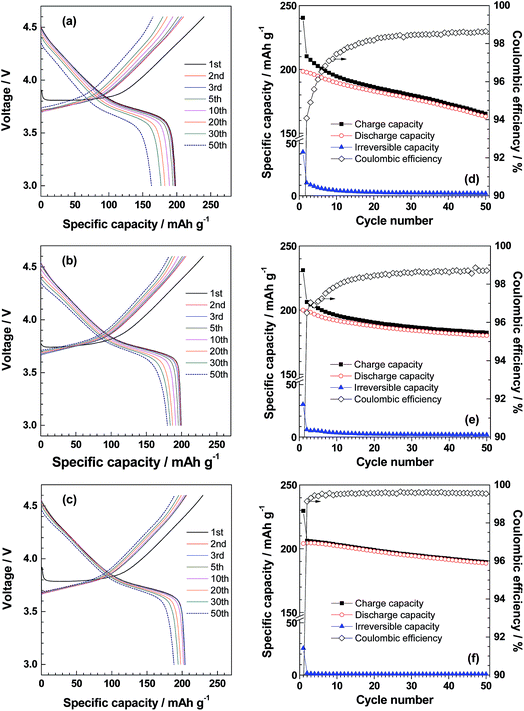 |
| Fig. 2 (a–c) Charge and discharge curves and (d–f) variations of capacities and coulombic efficiency of Li|LiNi0.5Co0.2Mn0.3O2 cells with cycle number using (a and d) 0.93 mol kg−1 (ca. 1 M), (b and e) 3.70 mol kg−1, and (c and f) nearly saturated 8.67 mol kg−1 LiBF4/DMC. | |
Table 1 Charge, discharge, and irreversible capacities and coulombic efficiency in the 1st cycle, and capacity retention at the 50th cycle of Li|LiNi0.5Co0.2Mn0.3O2 cells using 0.93, 3.70 and 8.67 mol kg−1 LiBF4/DMC. The charging cut off voltage was 4.6 V
LiBF4/DMC |
1st cycle |
50th cycle |
Concentration (mol kg−1) |
DMC/Li molar ratio |
Charge capacity (mA h g−1) |
Discharge capacity (mA h g−1) |
Irreversible capacity (mA h g−1) |
Coulombic efficiency (%) |
Capacity retentiona (%) |
Defined as percent ratios of discharge capacity in the 50th cycle to that in the 1st cycle.
|
0.93 |
11.8 |
240 |
199 |
41 |
82.6 |
82.2 |
3.70 |
3.00 |
231 |
200 |
31 |
86.5 |
90.0 |
8.67 |
1.28 |
230 |
204 |
26 |
88.9 |
92.2 |
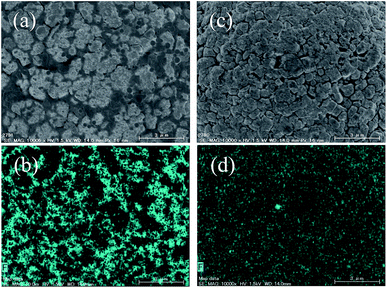 |
| Fig. 3 SEM/EDX images of LiNi0.5Co0.2Mn0.3O2 electrodes after 50 charge/discharge cycles between 3.0 and 4.6 V in (a and b) 0.93 mol kg−1 (ca. 1 M) and (c and d) nearly saturated 8.67 mol kg−1 LiBF4/DMC. | |
Dilution of highly concentrated LiBF4/DMC with fluoroalkyl ether
The viscosity of 8.67 mol kg−1 LiBF4/DMC (310 mPa s) was still high for practical application. We previously reported that the highly concentrated LiBF4/PC system can be diluted with fluoroalkyl ether to reduce the viscosity;40 the HFE is hardly involved in the solvation of Li+, and serves just as a diluting solvent. As a result, we obtained 33.3 vol% HFE-diluted solution (2.50 mol kg−1 LiBF4/PC + HFE (2
:
1)) as a compromise of an acceptably low PC/Li molar ratio (2.39) and a low viscosity (51.7 mPa s).40 A Li| LiNi0.5Mn0.2Co0.3O2 cell with 2.50 mol kg−1 LiBF4/PC + HFE (2
:
1) gave almost the same initial discharge capacity (198 mA h g−1) as that for nearly concentrated 7.25 mol kg−1 LiBF4/PC, and exhibited higher rate-capability than it (Fig. S2†). However, the ionic conductivity of 2.50 mol kg−1 LiBF4/PC + HFE (2
:
1) remained at 0.58 mS cm−1 at 25 °C, which was about 10 times as low as that of conventional 1 M LiPF6/EC + DMC electrolyte solution (Fig. S3†). The viscosity of a conventional 1 M LiPF6/EC + DMC electrolyte solution is ca. 3 mPa s, and hence a further decrease in viscosity, as well as electrolyte concentration, is required for practical application. However, further HFE-dilution resulted in an increase in the PC/Li molar ratio due to the low miscibility between HFE and LiBF4/PC, which impairs the stability against oxidation. In this work, we used two kinds of fluoroalkyl ether, including HFE, as a diluent for a highly concentrated LiBF4/DMC system. To obtain an electrolyte solution with acceptably low viscosity, concentration and DMC/Li molar ratio, 3.70 mol kg−1 LiBF4/DMC (DMC/Li molar ratio = 3.00) was diluted with HFE. As a result, 50 vol% HFE-diluted solution, i.e., 1.52 mol kg−1 LiBF4/DMC + HFE (1
:
1 by vol), maintained a low DMC/Li ratio (3.00), and showed a low viscosity of 3.6 mPa s. Further dilution resulted in a decrease in the solubility and an increase in the DMC/Li molar ratio because HFE can hardly dissolve LiBF4, and exhibits low miscibility with highly concentrated LiBF4/DMC. In fact, 67 vol% HFE-diluted solution (DMC
:
HFE = 1
:
2) dissolved only 0.73 mol kg−1 LiBF4, where the DMC/Li molar ratio rose to 3.96. Another fluoroalkyl ether, TFEE (−15.26 eV), has a much lower HOMO energy than HFE (−13.91 eV), and hence the stability against oxidation is expected to be higher than HFE. As with HFE, TFEE can hardly dissolve LiBF4. 1.55 mol kg−1 LiBF4 was dissolved in a solvent mixture of DMC + TFEE (1
:
1), which has the same DMC/Li ratio of 3.00 as 1.52 mol kg−1 LiBF4/DMC + HFE (1
:
1 by vol). The viscosity of the resultant solutions was low at around 3 mPa s.
Charge/discharge performance of LiNi0.5Mn0.2Co0.3O2 electrodes in fluoroalkyl ether-diluted LiBF4/DMC electrolyte solution
Fig. 4 shows the Raman spectra of 1.52 mol kg−1 LiBF4/DMC + HFE (1
:
1) and 1.55 mol kg−1 LiBF4/DMC + TFEE (1
:
1), together with 3.70 mol kg−1 LiBF4/DMC, all of which have an equal DMC/Li molar ratio of 3.00. The relative area ratios of ca. 916 and 934 cm−1 peaks are summarized in Table 2. The fraction of free DMC molecules remained low in the HFE-diluted solution, while the dilution with TFEE resulted in a slight increase by about 10%. These results indicate that TFEE is more involved in the solvation of Li+ than HFE, and therefore seem to be less suitable as a diluent. In fact, the capacity fading of NCM523 in the TFEE-diluted electrolyte solution (Fig. 5a) was more noticeable than that for the HFE-diluted one (Fig. 5b). The low charge/discharge performance would be associated with the large fraction of free DMC molecules and/or TFEE vulnerable to oxidation at more than 4.5 V (Fig. S4†). On the other hand, in the HFE-diluted electrolyte solution, the initial discharge capacity and coulombic efficiency were almost equal in both 1.52 mol kg−1 LiBF4/DMC + HFE (1
:
1 by volume, DMC/Li molar ratio = 3.00) and 0.73 mol kg−1 LiBF4/DMC + HFE (1
:
2 by volume, DMC/Li ratio = 3.96), as indicated in Fig. 5b and c, respectively. A major difference was seen upon repeated charge/discharge cycling; the charge/discharge capacities were considerably reduced in 0.73 mol kg−1 LiBF4/DMC + HFE (1
:
2), while a relatively high discharge capacity (186 mA h g−1), which corresponded to 93.4% of the initial discharge capacity, was maintained even after 50 cycles in 1.52 mol kg−1 LiBF4/DMC + HFE (1
:
1) (Fig. 5b). The present results clearly show that the highly concentrated LiBF4/DMC system can be diluted with HFE without impairing the charge/discharge performance as long as the DMC/Li ratio is kept low (less than 3). Thus, a low-viscosity and -concentration electrolyte solution with high stability against oxidation for NCM523 was developed. Finally, the discharge curves in Fig. 5b were integrated by evaluating the energy density of NCM523. The energy density reached a maximum of 784 W h kg−1, which was comparable to that obtained for the nearly saturated 8.67 mol kg−1 LiBF4/DMC, and even higher than that for a conventional 1 M LiPF6/EC + DEC. Moreover, 5 V class LiNi0.5Mn1.5O4 electrodes could work between 3.0 and 5.0 V by substituting PC for DMC in 1.52 mol kg−1 LiBF4/DMC + HFE (1
:
1). However, the energy density of the LiNi0.5Mn1.5O4 electrode remained below 613 W h kg−1, as shown in Fig. 5d. Thus, NCM523 far exceeded LiNi0.5Mn1.5O4 in energy density.
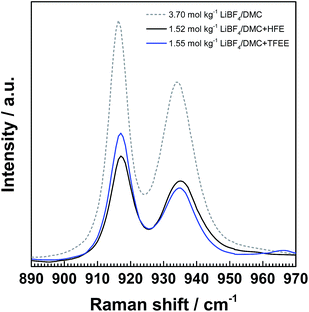 |
| Fig. 4 Raman spectra of 3.70 mol kg−1 LiBF4/DMC, 1.52 mol kg−1 LiBF4DMC + HFE (1 : 1) and 1.55 mol kg−1 LiBF4/DMC + TFEE (1 : 1). | |
Table 2 Relative area ratios (%) of Raman peaks assigned to free DMC at ca. 916 cm−1 and to solvating DMC at ca. 934 cm−1 in Fig. 4
|
DMC/Li molar ratio |
Free DMC |
Solvating DMC |
3.70 mol kg−1 LiBF4/DMC |
3.00 |
43.8 |
56.2 |
1.52 mol kg−1 LiBF4/DMC + HFE |
3.00 |
44.6 |
55.4 |
1.55 mol kg−1 LiBF4/DMC + TFEE |
3.00 |
55.8 |
44.2 |
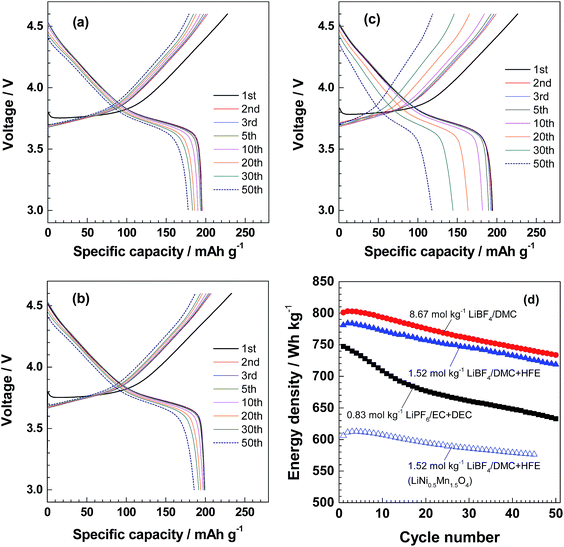 |
| Fig. 5 (a) Charge and discharge curves of Li|LiNi0.5Co0.2Mn0.3O2 cells using (a) 1.55 mol kg−1 LiBF4/DMC + TFEE (1 : 1 by volume, DMC/Li ratio = 3.00), (b) 1.52 mol kg−1 LiBF4/DMC + HFE (1 : 1 by volume, DMC/Li ratio = 3.00), and (c) 0.73 mol kg−1 LiBF4/DMC + HFE (1 : 2 by volume, DMC/Li ratio = 3.96). (d) Variation of energy density of LiNi0.5Co0.2Mn0.3O2 electrodes with cycle number, together with that of a LiNi0.5Mn1.5O4 electrode in 1.52 mol kg−1 LiBF4/DMC + HFE (1 : 1 by volume, DMC/Li ratio = 3.00) for comparison. | |
Conclusions
DMC was almost saturated at room temperature with LiBF4 at a very high concentration of 8.67 mol kg−1. The BF4− anions, as well as DMC, were stabilized against oxidation in the highly concentrated LiBF4/DMC. As a result, 8.67 mol kg−1 LiBF4/DMC enabled high-voltage operation of NCM523 electrodes, and greatly improved the charge/discharge performance of NCM523. The highly concentrated LiBF4/DMC could be diluted with a specific fluoalkyl ether (HFE) without impairing the charge/discharge performance; 50 vol% HFE-diluted solution, i.e., 1.52 mol kg−1 LiBF4/DMC + HFE (1
:
1), satisfied the requirements of low viscosity, concentration, and DMC/Li molar ratio, and elicited a high discharge capacity of ca. 200 mA h g−1 by charging the NCM523 electrodes to 4.6 V. The improved charge/discharge performance was maintained even after 50 charge/discharge cycles, which was comparable to that obtained for the nearly saturated 8.67 mol kg−1 LiBF4/DMC, and even better than that for a conventional 1 M LiPF6/EC + DEC electrolyte solution. The energy density of NCM523 reached a maximum of 784 Wh kg−1, and far exceeded that of 5 V class LiNi0.5Mn1.5O4. Thus, the electrolyte solution developed in this study possessed high stability against oxidation, low viscosity, and relatively low concentration. However, it is volatile (Fig. S5†) and the Li concentration of 1.52 mol kg−1 LiBF4 is still a little high, as compared with the conventional electrolyte solution. Diluents with low volatility, higher miscibility and stability against oxidation are required for realizing a LiBF4/DMC-based electrolyte solution for use in practical LIBs.
Conflicts of interest
There are no conflicts to declare.
Acknowledgements
The authors thank Dr Katsuyuki Takahashi, Ms. Hiroe Nakagawa, Mr Shuji Hitomi, and Dr Tokuo Inamasu of GS Yuasa International Ltd. for their helpful discussion. This research was partially supported by “the Super Cluster Program” from MEXT and JST and “Kyoto Regional Scientific Innovation Hub” from MEXT.
References
- J. M. Tarascon, E. Wang, F. K. Shokoohi, W. R. McKinnon and S. Colson, The Spinel Phase of LiMn2O4 as a Cathode in Secondary Lithium Cells, J. Electrochem. Soc., 1991, 138, 2859–2864 CrossRef CAS.
- T. Ohzuku, A. Ueda, M. Nagayama, Y. Iwakoshi and H. Komori, Comparative study of LiCoO2, LiNi12Co12O2 and LiNiO2 for 4 volt secondary lithium cells, Electrochim. Acta, 1993, 38, 1159–1167 CrossRef CAS.
- R. Fong, U. von Sacken and J. R. Dahn, Studies of lithium intercalation into carbons using nonaqueous electrochemical cells, J. Electrochem. Soc., 1990, 137, 2009–2013 CrossRef CAS.
- S. Flandrois and B. Simon, Carbon materials for lithium-ion rechargeable batteries, Carbon, 1999, 37, 165–180 CrossRef CAS.
- Z. Liu, A. Yu and J. Y. Lee, Synthesis and characterization of LiNi1−x−yCoxMnyO2 as the cathode materials of secondary lithium batteries, J. Power Sources, 1999, 81–82, 416–419 CrossRef CAS.
- T. Ohzuku and Y. Makimura, Layered lithium insertion material of LiCo1/3Ni1/3Mn1/3O2 for lithium-ion batteries, Chem. Lett., 2001, 30, 642–643 CrossRef.
- H. Zheng, Q. Sun, G. Liu, X. Song and V. S. Battaglia, Correlation between dissolution behavior and electrochemical cycling performance for LiNi1/3Co1/3Mn1/3O2-based cells, J. Power Sources, 2012, 207, 134–140 CrossRef CAS.
- S.-K. Jung, H. Gwon, J. Hong, K.-Y. Park, D.-H. Seo, H. Kim, J. Hyun, W. Yang and K. Kang, Understanding the degradation mechanisms of LiNi0.5Co0.2Mn0.3O2 cathode material in lithium ion batteries, Adv. Energy Mater., 2014, 4, 1300787 CrossRef.
- W. Liu, P. Oh, X. Liu, M.-J. Lee, W. Cho, S. Chae, Y. Kim and J. Cho, Nickel-rich layered lithium transition-metal oxide for high-energy lithium-ion batteries, Angew. Chem., Int. Ed., 2015, 54, 4440–4457 CrossRef CAS PubMed.
- J. Zheng, W. H. Kan and A. Manthiram, Role of Mn content on the electrochemical properties of nickel-rich layered LiNi0.8−xCo0.1Mn0.1+xO2 (0.0 ≤ x ≤ 0.08) cathodes for lithium-ion batteries, ACS Appl. Mater. Interfaces, 2015, 7, 6926–6934 CAS.
- J. W. Choi and D. Aurbach, Promise and reality of post-lithium-ion batteries with high energy densities, Nat. Rev. Mater., 2016, 1, 16013 CrossRef CAS.
- W. Li, B. Song and A. Manthiram, High-voltage positive electrode materials for lithium-ion batteries, Chem. Soc. Rev., 2017, 46, 3006–3059 RSC.
- E.-J. Lee, Z. Chen, H.-J. Noh, S. C. Nam, S. Kang, D. H. Kim, K. Amine and Y.-K. Sun, Development of microstrain in aged lithium transition metal oxides, Nano Lett., 2014, 14, 4873–4880 CrossRef CAS PubMed.
- K. Meng, Z. Wang, H. Guo, X. Li and D. Wang, Improving the cycling performance of LiNi0.8Co0.1Mn0.1O2 by surface coating with Li2TiO3, Electrochim. Acta, 2016, 211, 822–831 CrossRef CAS.
- S.-U. Woo, C. S. Yoon, K. Amine, I. Belharouak and Y.-K. Sun, Significant improvement of electrochemical performance of AlF3-coated LiNi0.8Co0.1Mn0.1O2 cathode materials, J. Electrochem. Soc., 2007, 154, A1005–A1009 CrossRef CAS.
- D. P. Abraham, R. D. Twesten, M. Balasubramanian, I. Petrov, J. McBreen and K. Amine, Surface changes on LiNi0.8Co0.2O2 particles during testing of high-power lithium-ion cells, Electrochem. Commun., 2002, 4, 620–625 CrossRef CAS.
- M. Ue, M. Takeda, M. Takehara and S. Mori, Electrochemical properties of quaternary ammonium salts for electrochemical capacitors, J. Electrochem. Soc., 1997, 144, 2684–2688 CrossRef CAS.
- K. Xu, Nonaqueous liquid electrolytes for lithium-based rechargeable batteries, Chem. Rev., 2004, 104, 4303–4418 CrossRef CAS PubMed.
- Y. Hu, H. Li, X. Huang and L. Chen, Novel room temperature molten salt electrolyte based on LiTFSI and acetamide for lithium batteries, Electrochem. Commun., 2004, 6, 28–32 CrossRef CAS.
- W. A. Henderson, F. McKenna, M. A. Khan, N. R. Brooks, V. G. Young and R. Frech, Glyme–lithium bis(trifluoromethanesulfonyl)imide and glyme–lithium bis(perfluoroethanesulfonyl)imide phase behavior and solvate structures, Chem. Mater., 2005, 17, 2284–2289 CrossRef CAS.
- L. Suo, Y.-S. Hu, H. Li, M. Armand and L. Chen, A new class of solvent-in-salt electrolyte for high-energy rechargeable metallic lithium batteries, Nat. Commun., 2013, 4, 1481 CrossRef PubMed.
- Y. Yamada and A. Yamada, Review-superconcentrated electrolytes for lithium batteries, J. Electrochem. Soc., 2015, 162, A2406–A2423 CrossRef CAS.
- J. Wang, Y. Yamada, K. Sodeyama, C. H. Chiang, Y. Tateyama and A. Yamada, Superconcentrated electrolytes for a high-voltage lithium-ion battery, Nat. Commun., 2016, 7, 12032 CrossRef CAS PubMed.
- K. Yoshida, M. Nakamura, Y. Kazue, N. Tachikawa, S. Tsuzuki, S. Seki, K. Dokko and M. Watanabe, Oxidative-stability enhancement and charge transport mechanism in glyme–lithium salt equimolar complexes, J. Am. Chem. Soc., 2011, 133, 13121–13129 CrossRef CAS PubMed.
- C. Zhang, A. Yamazaki, J. Murai, J.-W. Park, T. Mandai, K. Ueno, K. Dokko and M. Watanabe, Chelate effects in glyme/lithium bis(trifluoromethanesulfonyl)amide solvate ionic liquids, part 2: importance of solvate-structure stability for electrolytes of lithium batteries, J. Phys. Chem. C, 2014, 118, 17362–17373 CAS.
- Y. Kameda, Y. Umebayashi, M. Takeuchi, M. A. Wahab, S. Fukuda, S.-I. Ishiguro, M. Sasaki, Y. Amo and T. Usuki, Solvation structure of Li+ in concentrated LiPF6–propylene carbonate solutions, J. Phys. Chem. B, 2007, 111, 6104–6109 CrossRef CAS PubMed.
- M. D. Bhatt, M. Cho and K. Cho, Conduction of Li+ cations in ethylene carbonate (EC) and propylene carbonate (PC): comparative studies using density functional theory, J. Solid State Electrochem., 2012, 16, 435–441 CrossRef CAS.
- X. Bogle, R. Vazquez, S. Greenbaum, A. V. W. Cresce and K. Xu, Understanding Li+–solvent interaction in nonaqueous carbonate electrolytes with 17O NMR, J. Phys. Chem. Lett., 2013, 4, 1664–1668 CrossRef CAS PubMed.
- J. L. Allen, O. Borodin, D. M. Seo and W. A. Henderson, Combined quantum chemical/Raman spectroscopic analyses of Li+ cation solvation: cyclic carbonate solvents – ethylene carbonate and propylene carbonate, J. Power Sources, 2014, 267, 821–830 CrossRef CAS.
- I. Skarmoutsos, V. Ponnuchamy, V. Vetere and S. Mossa, Li+ solvation in pure, binary, and ternary mixtures of organic carbonate electrolytes, J. Phys. Chem. C, 2015, 119, 4502–4515 CAS.
- M. D. Bhatt, M. Cho and K. Cho, Interaction of Li+ ions with ethylene carbonate (EC): density functional theory calculations, Appl. Surf. Sci., 2010, 257, 1463–1468 CrossRef CAS.
- M. Nie, D. P. Abraham, D. M. Seo, Y. Chen, A. Bose and B. L. Lucht, Role of Solution Structure in Solid Electrolyte Interphase Formation on Graphite with LiPF6 in Propylene Carbonate, J. Phys. Chem. C, 2013, 117, 25381–25389 CAS.
- T. Doi, R. Masuhara, M. Hashinokuchi, Y. Shimizu and M. Inaba, Concentrated LiPF6/PC electrolyte solutions for 5-V LiNi0.5Mn1.5O4 positive electrode in lithium-ion batteries, Electrochim. Acta, 2016, 209, 219–224 CrossRef CAS.
- T. Doi, Y. Shimizu, M. Hashinokuchi and M. Inaba, LiBF4-based concentrated electrolyte solutions for suppression of electrolyte decomposition and rapid lithium-ion transfer at LiNi0.5Mn1.5O4/electrolyte interface, J. Electrochem. Soc., 2016, 163, A2211–A2215 CrossRef CAS.
- T. Doi, Y. Shimizu, M. Hashinokuchi and M. Inaba, Low-viscosity γ-butyrolactone-based concentrated electrolyte solutions for LiNi0.5Mn1.5O4 positive electrodes in lithium-ion batteries, ChemElectroChem, 2017, 4, 2398–2403 CrossRef CAS.
- T. Doi, Y. Shimizu, R. Matsumoto, M. Hashinokuchi and M. Inaba, Suppression of Mn-ion-dissolution of LiNi0.5Mn1.5O4 electrodes in a highly concentrated electrolyte solution at elevated temperatures, ChemistrySelect, 2017, 2, 8824–8827 CrossRef CAS.
- K. Dokko, N. Tachikawa, K. Yamauchi, M. Tsuchiya, A. Yamazaki, E. Takashima, J.-W. Park, K. Ueno, M. Watanabe, S. Seki and N. Serizawa, Solvate ionic liquid electrolyte for Li–S batteries, J. Electrochem. Soc., 2013, 160, A1304–A1310 CrossRef CAS.
- N. Azimi, W. Weng, C. Takoudis and Z. Zhang, Improved performance of lithium–sulfur battery with fluorinated electrolyte, Electrochem. Commun., 2013, 37, 96–99 CrossRef CAS.
- M. Watanabe, M. L. Thomas, S. Zhang, K. Ueno, T. Yasuda and K. Dokko, Application of ionic liquids to energy storage and conversion materials and devices, Chem. Rev., 2017, 117, 7190–7239 CrossRef CAS PubMed.
- T. Doi, Y. Shimizu, M. Hashinokuchi and M. Inaba, Dilution of highly concentrated LiBF4/propylene carbonate electrolyte solution with fluoroalkyl ethers for 5-V LiNi0.5Mn1.5O4 positive electrodes, J. Electrochem. Soc., 2017, 164, A6412–A6416 CrossRef CAS.
- L. Doucey, M. Revault, A. Lautié, A. Chaussé and R. Messina, A study of the Li/Li+ couple in DMC and PC solvents : Part 1: characterization of LiAsF6/DMC and LiAsF6/PC solutions, Electrochim. Acta, 1999, 44, 2371–2377 CrossRef CAS.
- J. E. Katon and M. D. Cohen, The vibrational spectra and structure of dimethyl carbonate and its conformational behavior, Can. J. Chem., 1975, 53, 1378–1386 CrossRef CAS.
- M. Morita, Y. Asai, N. Yoshimoto and M. Ishikawa, A Raman spectroscopic study of organic electrolyte solutions based on binary solvent systems of ethylene carbonate with low viscosity solvents which dissolve different lithium salts, J. Chem. Soc., Faraday Trans., 1998, 94, 3451–3456 RSC.
- H. Bohets and B. J. Van Der Veken, On the conformational behavior of dimethyl carbonate, Phys. Chem. Chem. Phys., 1999, 1, 1817–1826 RSC.
- H. Tsunekawa, A. Narumi, M. Sano, A. Hiwara, M. Fujita and H. Yokoyama, Solvation and ion association studies of LiBF4-propylenecarbonate and LiBF4-propylenecarbonate-trimethyl phosphate solutions, J. Phys. Chem. B, 2003, 107, 10962–10966 CrossRef CAS.
- D. M. Seo, O. Borodin, S.-D. Han, Q. Ly, P. D. Boyle and W. A. Henderson, Electrolyte solvation and ionic association I. Acetonitrile–lithium salt mixtures: intermediate and highly associated salts, J. Electrochem. Soc., 2012, 159, A553–A565 CrossRef CAS.
Footnote |
† Electronic supplementary information (ESI) available. See DOI: 10.1039/c8se00036k |
|
This journal is © The Royal Society of Chemistry 2018 |