Understanding the slowdown of whole slurry hydrolysis of steam pretreated lignocellulosic woody biomass catalyzed by an up-to-date enzyme cocktail
Received
25th November 2017
, Accepted 18th February 2018
First published on 19th February 2018
Abstract
Although steam pretreatment could efficiently pretreat various lignocellulosic biomass, it generates water-soluble inhibitory components which significantly slow down cellulose hydrolysis. Most of the previous studies have only evaluated the effect of the inhibitors on the traditional cellulase cocktails while the detailed mechanisms of the pretreatment derived inhibitors on the recently developed enzyme cocktails remain unclear. In addition, the previous studies either focused on the inhibition of cellulose hydrolysis or the inhibition of the activities of cellulases, while it is not elucidated how the cellulose hydrolysis performance is associated with the inhibition of major enzyme activities in the presence of pretreatment derived inhibitors. This study looked at how the water-soluble compounds derived from two typical steam pretreated woody biomasses (lodgepole pine and poplar) might affect the cellulose hydrolysis of pretreated biomass by using the state-of-the-art enzyme preparation (CTec3) and also evaluated their influence on the major enzyme activities. The result showed that both enzyme inhibition and deactivation contribute to the overall decrease in the cellulose hydrolysis. Among the water-soluble compounds, the activities of cellobiohydrolases (CBHs) and β-glucosidases (BGs) were strongly inhibited by sugars in the water-soluble fraction while CBH and xylanase activities were deactivated by phenolics, resulting in the overall decrease in cellulose hydrolysis. Although the major enzymes were inhibited/deactivated significantly, it appeared that the initial cellulose hydrolysis rate was only slightly affected. Instead, the extent of cellulose hydrolysis correlated closely with the inhibition/deactivation of the major enzyme activities.
Introduction
The replacement of traditional hydrocarbon based oil refineries with carbohydrate based refineries has been considered as one of the most promising strategies to solve many increasingly serious global issues such as energy insecurity and climate changes.1,2 The bioconversion of lignocellulosic biomass to fuels and chemicals has many advantages in this biorefinery concept.3 In the biomass bioconversion process, a physicochemical pretreatment step is firstly needed to open up the cell wall structure and facilitate the following enzymatic hydrolysis so as to produce fermentable sugars.3 Among all the pretreatment strategies, steam pretreatment has been widely used at the demonstration and commercialization scales because it can efficiently pretreat a wide range of biomass with low/no demand of catalysts and solvents.4,5 However, one of the drawbacks of steam pretreatment is that the various biomass degradation products derived from the pretreatment process (e.g. sugars, phenolics, furans and organic acids) could significantly inhibit the following enzymatic hydrolysis and fermentation processes.6–10
Previous cellulase inhibition studies have mainly assessed the inhibitory effects of pretreatment derived inhibitors on either the purified cellulase components11 or the traditional cellulase preparations such as Celluclast and Spezyme CP.12 As enzyme cocktails have evolved to be more tolerant toward the pretreatment derived inhibitors, it is desirable to further understand the inhibition mechanism of cellulose hydrolysis catalyzed by the newly developed enzyme cocktails such as CTec3. In addition, the newly developed enzyme cocktails contain not only cellulases but also various so-called “accessory enzymes” such as hemicellulases.13,14 As these accessory enzymes play an essential role in hydrolyzing the pretreated lignocellulosic substrates, it is essential to also assess how they may be affected by the pretreatment derived inhibitors. Therefore, in order to better understand the inhibition mechanisms and develop more efficient inhibition mitigation strategies, it would be desirable to further evaluate the influence of the pretreatment derived soluble inhibitors on the major enzyme activities present in the state-of-the-art enzyme preparation such as CTec3.
The inhibitory effects of soluble compounds on cellulose hydrolysis by using traditional enzyme cocktails have been reported widely in recent years. Regarding the major cause for the decrease of cellulose hydrolysis, some study evaluated the inhibition of enzyme activities and reported that glucose and phenolics selectively inhibited and deactivated β-glucosidase (BG).15,16 Some studies assessed the inhibition of purified cellobiohydrolases (CBHs) and found that it was strongly inhibited by the phenolics. In addition, some studies also suggested that endoglucanase (EG) was sensitive to the presence of tannic acid.16 Although these studies generally agree that the pretreatment-derived inhibitors reduce the cellulase activities, it seems the major enzyme components such as CBH, BG, EG could all be inhibited by various water-soluble inhibitors. Thus, it is hard to compare these observations and draw a clear picture about how the inhibition of cellulase might lead to the decrease in cellulose hydrolysis, as these studies used different enzyme mixtures and different types of inhibitors.
This study systematically assessed how these pretreatment-derived inhibitors from two typical woody biomass (lodgepole pine and poplar) could affect the time course of cellulose hydrolysis as well as the activities of the major group of enzymes such as CBH, EG, and BG, and the activities of the major “accessory” enzyme xylanase of the CTec3 cellulase preparation. By using the same type of inhibitors and enzyme mixture, we could identify the enzyme components most sensitive towards the inhibitors and the enzyme components responsible for the decrease of cellulose hydrolysis. In addition, this work has also identified the major inhibitors/deactivators that are responsible for the slowdown of the rate and extent of cellulose hydrolysis.
Materials and methods
Lignocellulosic materials and steam pretreatment
Lodgepole pine killed by mountain pine beetles (Pinus contorta) and hybrid poplar were obtained from the British Columbia Forest service and FP Innovations, respectively. Steam pretreatment of the two types of woody biomass (lodgepole pine and poplar) was carried out in equipment consisting of a 2 L pressure vessel and a flash tank to collect the pretreated biomass slurry. After pretreatment, the biomass slurry was filtered into solid and liquid fractions (water-soluble fraction, WS). The composition of the solid fractions was 52.8% glucan, 2.0% mannan, and 42.3% Klason lignin for steam pretreated lodgepole pine (SPLP), while it was 58.7% glucan, 2.2% xylan, 1.4% mannan, 35.1% Klason lignin, and 1.7% acid-soluble lignin for steam pretreated poplar (SPP) (all values are calculated as % total dry weight (w/w) and measured using the modified Tappi T-222 method17,18).
The compositions of SPLP-WS and SPP-WS were the same as those published previously.19 The sugar composition was measured by using a high performance liquid chromatography (HPLC) system and the phenolics were measured by using the Folin–Ciocalteu method and expressed as the equivalent vanillin amount according to a previous study.19 The major composition of the WS fractions of steam pretreated lodgepole pine (SPLP) was 4.8 g L−1 arabinose, 8.5 g L−1 galactose, 19.8 g L−1 glucose, 15.4 g L−1 xylose, 27.2 g L−1 mannose, and 4.5 g L−1 phenolics, whereas the composition of WS fractions of poplar (SPP) was 1.3 g L−1 arabinose, 2.7 g L−1 galactose, 22.9 g L−1 glucose, 52.1 g L−1 xylose, 3.9 g L−1 mannose, and 9.8 g L−1 phenolics. In order to identify the effect of phenolics on the major enzyme activities, partial phenolics were removed by using activated carbon treatment. The phenolics composition of the WS fraction after activated carbon treatment is 0.3 g L−1 for SPLP-WS and 3.1 g L−1 for SPP-WS, while other components were only slightly affected.
Enzyme preparations and enzyme assay procedures
Commercial cellulase (Cellic CTec3) was obtained from Novozyme, North America, Inc. (Franklinton, NC). The total protein content of CTec3 (245 mg protein per mL enzyme cocktail) was determined according to the ninhydrin method with bovine serum albumin as the protein standard.20 β-Glucosidase activity was measured using p-nitrophenyl-β-D-glucoside (Wood and Bhat, 1988).21 Briefly, 50 μL of β-D-glucopyranoside (PNPG) substrate solution (10 mM) was mixed with 50 μL of diluted enzyme preparation and the reaction mixture was incubated at 50 °C for 30 min. The reaction was stopped by the addition of 100 μL of NaOH glycine buffer solution (100 mM). The concentration of p-nitrophenol produced was measured by the absorbance at 410 nm. Cellobiohydrolase (CBH) activity was measured under similar conditions using p-nithrophenyl-β-D-cellobioside (PNPC) as the substrate in the presence of D-glucono-1,5-δ-lactone to inhibit β-glucosidase activity.22 Although endoglucanase and Cel6A can also hydrolyse the substrate, previous studies have shown that they have considerably lower activity than Cel7A. Thus, the CBH activity was mainly due to the Cel7A component. Enzyme activity was expressed as international units (U), where one U releases one μmol p-nitrophenol per min. Experiments were performed in triplicate and the mean values were calculated. CBH and BG activities corresponded to 2 and 15 U per mg protein, respectively. Endoglucanase (EG) activity was determined as described previously.23 The total reaction mixture (100 μL) contained a suitably diluted enzyme and 0.7% (w/v) of carboxymethyl cellulose (CMC, Sigma-Aldrich) solution in 50 mM acetate buffer. Xylanase activity was determined using a 1% xylan solution (birchwood, Sigma-Aldrich) as the substrate under similar conditions.14,24 The enzyme reaction was stopped by adding 200 μL of 3,5-dinitrosalicylic acid (DNS) reagent after 10, 20, and 30 min of incubation. The microplates were then placed in an oven at 105 °C for 30 min and the reducing sugar content of the samples was analyzed by measuring the absorbance at 540 nm.23 Xylose and glucose were used as standards for calibration. The EG and xylanase activities were expressed in international units (U) defined as 1 μmol of glycoside bonds of the substrate hydrolysed per minute. Experiments were performed in triplicate and the mean values were calculated. EG and xylanase activities corresponded to 6 and 14 U per mg protein, respectively.
It is difficult (and sometimes impossible) to quantify a small amount of product formed using the DNS method against a high background of reducing sugars added as possible inhibitors.25 Therefore, when enzyme inhibition/deactivation was studied, EG activity and xylanase activity in the presence of hemicellulose sugar rich water-soluble fractions (WSs) were estimated using relatively indirect assays based on the release of dyes labelled in the matrix of CMC or birchwood xylan. Both substrates (CMC and xylan) were labelled with Remazol Brilliant Blue (RBB). The Remazol Brilliant Blue labelled CMC and birchwood xylan were prepared according to a modified method of Biely et al.26
Enzyme inhibition
The water-soluble (WS) fractions derived from steam pretreated lodgepole pine and poplar were referred to as SPP-WS and SPLP-WS, respectively. These water-soluble (WS) fractions were first neutralized with NaOH to pH 4.8. A series of volumes of water-soluble fractions (WSs) were then mixed with a substrate solution and a diluted enzyme solution. Activities were then determined in the presence of the WS fractions, as mentioned in the previous section (Enzyme preparations and enzyme assay procedures). The activity assayed in the absence of pretreatment liquors was defined as the control activity of 100%. All reactions were carried out in triplicate and the residual enzyme activity was expressed as the mean.
Enzyme deactivation
Pretreatment-derived soluble compounds have been reported to inhibit not only the initial hydrolysis rate but also the extent of hydrolysis. The enzyme activity assays only evaluated the inhibitory effect of pretreatment-derived water-soluble fractions on the catalytic action of enzymes over the initial stages of hydrolysis. To investigate the possible deactivation effects of pretreatment-derived soluble compounds on the major groups of enzyme activities during the time course of hydrolysis, the extent of enzyme activity loss was examined using the same enzyme activity assay procedure as described previously.12 The deactivation effects of pretreatment liquors on specific activities were determined by pre-incubating the enzyme with the WS fractions (pH 4.8 and 50 °C) for 0–72 h. The enzyme mixtures were combined with each WS fraction to achieve a final concentration of 2.8 mg mL−1. This was within the range of enzyme loading normally used for high-solid cellulose hydrolysis.27,28 After an appropriate period of time (0–72 h), samples of the incubation mixture were withdrawn, diluted 100–1000 fold and then added to the standard assay mixture (PNPG, PNPC, CMC and xylan). The residual activities of BG, CBH, EG and xylanase were determined.
Results and discussion
Inhibition of cellulose hydrolysis of steam pretreated biomass by pretreatment-derived water-soluble compounds
The possible inhibitory effects of the pretreatment-derived water-soluble compounds on cellulose hydrolysis of SPLP and SPP were evaluated by using CTec3 at optimized enzyme loadings. It is clear that the water-soluble fraction derived from both types of biomass after steam pretreatment strongly inhibited the overall cellulose hydrolysis (Fig. 1). When the whole slurry was used as substrates, stronger inhibition of cellulose hydrolysis was observed, with cellulose hydrolysis decreased by 20–40% (Fig. 1). Although the concentrations of the major soluble compounds of SPP-WS were similar to that of SPLP-WS, it is clear that the enzyme inhibition caused by SPP-WS was more significant than that by SPLP-WS. This suggests that the composition of WSs has a great impact on their inhibitory effect on the cellulose hydrolysis. Interestingly, it was also found that the inhibition of the extent of cellulose hydrolysis was more significant than the inhibition of hydrolysis rate. This might indicate that the cellulases in the enzyme preparations might be affected through different inhibition mechanisms.
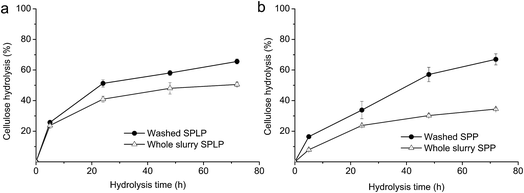 |
| Fig. 1 The influence of pretreatment derived soluble compounds on the cellulose hydrolysis of (a) steam pretreated lodgepole pine (SPLP) and (b) steam pretreated poplar (SPP). The cellulose hydrolysis of SPLP and SPP was catalyzed by CTec3 at an enzyme loading of 40 mg and 8 mg protein per g cellulose, respectively. The hydrolysis experiments were performed in triplicate and the error bars represent the standard deviation. | |
Inhibition of specific enzyme activities of CTec3 by pretreatment-derived water-soluble fractions (WSs)
As the water-soluble compound in the pretreated whole slurry could strongly inhibit the overall cellulose hydrolysis, even by using the more recent enzyme preparation CTec3, a more detailed understanding of the inhibition mechanism is still needed to further improve the tolerance of enzyme cocktails. As mentioned previously, cellobiohydrolases (CBHs), endoglucanases (EGs), beta-glucosidases (BGs) and xylanases are the major types of enzymes that govern the overall hydrolytic potential of cellulase preparations on various pretreated lignocellulosic substrates.29,30 Since these major enzyme components (CBHs, EGs, BGs and xylanases) contain quite different amino acid sequences and protein tertiary structures,31–33 it was anticipated that they might be inhibited through different mechanisms such as inhibition and/or deactivation by the inhibitors present within the WS fractions. As discussed previously, enzyme inhibition typically happens immediately after adding the inhibitors and the extent of inhibition follows a concentration-dependent manner, which means the enzyme activity can be recovered once these inhibitors are removed.34 In contrast, enzyme deactivation takes place after incubation of the enzymes with inhibitors and the enzyme activity cannot be recovered since the inhibitors have bound to the enzyme active sites irreversibly.34 Therefore, the enzyme inhibition effect has commonly been assessed by measuring the specific enzyme activities in the presence of inhibitory compounds at varied concentrations, since these inhibitors decrease enzyme activities in a very fast manner and the level of inhibition is positively correlated with inhibitor concentration.34
To assess the potential inhibitory effect of the water-soluble (WS) fractions, the major enzyme activities (EG, CBH, BG, xylanase activities) of CTec3 were measured with and without the supplementation of varied concentrations (0–100%) of the WS fractions by using dye labelled carboxymethyl cellulose (CMC), p-nitrophenyl-β-D-cellobioside (PNPC), p-nitrophenyl-β-D-glucopyranoside (PNPG) and dye labelled birchwood xylan, respectively. It was apparent that the WS fractions derived from both the pretreated softwood lodgepole pine (SPLP-WS) and the hardwood poplar (SPP-WS) exhibited similar inhibitory effects towards each of the enzyme activities (Fig. 2). Among these specific enzyme activities, it seemed that the BG and CBH activities were inhibited to a greater extent, especially at higher amounts of WS fractions (Fig. 2). However, it seems that the initial cellulose hydrolysis rate was only slightly decreased by these soluble compounds (Fig. 1a and b). It is possible that the higher substrate concentration at the initial stage of cellulose hydrolysis might provide sufficient substrates for the enzyme to bind and thus inhibit the binding of the enzyme to inhibitors.
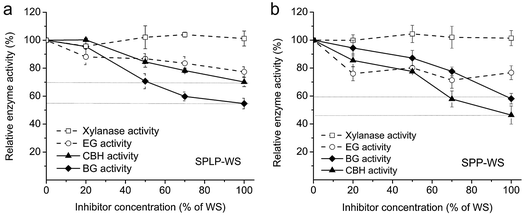 |
| Fig. 2 The influence of the water-soluble compounds on the xylanase, endoglucanase (EG), β-glucosidase (BG), and cellobiohydrolase (CBH) activities within CTec3 at various concentrations of (a) steam pretreated lodgepole pine derived water-soluble fraction (SPLP-WS) and (b) steam pretreated poplar derived water-soluble fraction (SPP-WS). The water-soluble (WS) fractions were added at the start of the assay. The inhibitor concentration was expressed as percentage of the concentration of WSs. 100% EG, CBH, BG, and xylanase activities corresponded to 6, 2, 15 and 14 U per mg protein, respectively. These values, measured in the absence of inhibitors, were used as a reference to calculate the loss of enzyme activities due to the presence of inhibitors. | |
Identifying the major inhibitors (sugars and phenolics) that account for the inhibitory effect of the water-soluble fractions (WSs) on the major enzyme activities
As sugars (especially monomeric sugars) and phenolics were the two major inhibitory compounds within the WS fractions from steam pretreated woody biomass19 and the CBH and BG activities were the main activities that were inhibited by these WS fractions (Fig. 2), we next assessed the potential inhibitory effects of sugars and phenolics on the CBH and BG activities respectively. The effects of monomeric sugars were evaluated by using the “synthetic sugar mixture” (the mixture of sugars present in the same sugar concentration/proportion as detected in the WS fraction). Generally, the BG activity was more strongly inhibited than the CBH activity by the monomeric sugar mixture (Fig. 2). At lower concentration (<70% of the WS fraction), the monomeric sugars had no influence on the CBH activities, but reduced more than 20% of BG activities. And even at the high concentration (100% of the WS fraction), CBH activities were only slightly inhibited (∼10%), while the BG activities were almost inhibited to the same level as the original WS fractions (Fig. 2). This phenomenon indicated that the monomeric sugars within the WS fractions were the main inhibitors towards the BG activities within the CTec3 preparation.
The observed extensive inhibition of BG activity by the “synthetic sugar mixture” was expected, since previous studies (by using purified cellulase monocomponents) have already shown that the monomeric sugars especially glucose strongly inhibit BG in a competitive manner, while inhibiting CBH activity to a much lesser extent in a non-competitive or uncompetitive manner.35,36 As a result of the strong inhibition of BG activity by glucose, cellobiose accumulates during cellulose hydrolysis, which could in turn strongly inhibit CBH activities and then lead to a slowdown of the enzymatic hydrolysis of cellulose.36,37 Although the hemicellulose sugar rich fraction also contains high concentration of mannose or xylose, after evaluating their effect on the major enzyme activities, it was observed that these sugars did not inhibit the major specific enzyme activities in the commercial enzyme cocktails (data not shown).
Aside from sugars, the effects of phenolics within the WS fractions on the BG and CBH activities were next evaluated. Since the steam pretreatment derived phenolic components have very complex and unknown structures, it has been very challenging to purify and/or synthesize these phenolic components.19 Therefore, to try to better understand the possible inhibition effects of these phenolics on the individual BG and CBH activities, we next used activated carbon (AC) to investigate how partial phenolics removal might alleviate their enzyme inhibition.19 It appeared that the selective removal of partial phenolics from the SPLP-WS and SPP-WS fractions significantly alleviated inhibition of CBH activity while not reducing the inhibition of BG activity, in a concentration dependent manner (Fig. 3). In addition, the SPP-WS derived phenolics showed a stronger inhibitory effect on CBH activity than the SPLP-WS derived phenolics (Fig. 3a and b).
 |
| Fig. 3 The influence of monomeric sugars and partial phenolics removal, using activated carbon treatment, from the water-soluble fractions (WSs) of steam pretreated lodgepole pine and poplar (SPLP/SPP-WS) on the activities of CBH (a and b) and BG (c and d). Phenolics were removed from SPLP/SPP-WS by using 5% (w/w) activated carbon treatment in order to obtain WS fractions with lower concentration of phenolics. These are referred to as SPLP/SPP-WS-5% AC. Inhibitor concentration is expressed as the percentage of original WSs. Inhibitors were added at the start of the assay. 100% CBH and BG activities corresponded to 2 and 15 U per mg protein, respectively. These values, measured in the absence of inhibitors were used as a reference to calculate the loss of enzyme activities due to the presence of inhibitors. | |
One of the potential reasons for the significant inhibition of CBH activity by phenolics is the possible strong interaction between CBH and phenolics since both the catalytic domain and the carbohydrate binding module (CBM) of CBHs contain several amino-acid residuals with aromatic side chains to interact with the cellulose during its processive hydrolysis process.38 Thus, it is possible that the pretreatment derived phenolics interacted with aromatic phenolics through hydrophobic interactions, and therefore significantly inhibited CBH activity (Fig. 3). In addition, the linkers of CBHs are rich in proline and also highly glycosylated.39 It has been shown that prolines in the protein structure could result in the formation of a loose and open structure which greatly facilitates the enzyme–phenolics interactions.40 Since this glycosylated linker is essential for both the binding of CBH to cellulose and the processivity of CBH during the hydrolysis,41 it is also possible that the phenolics decrease CBH activity by binding to their linker regions, consequently interrupting the interaction of the CBH with the cellulose. As BG does not have a similar structure to that of CBH for promoting enzyme–cellulose interaction,32,42 its activity showed much higher tolerance to phenolics than CBH activity (Fig. 3). It also appeared that phenolics-mediated inhibition was also highly dependent on the source of the original biomass feedstocks (Fig. 3). This might be related to different phenolic characteristics such as degree of polymerization,43 functional groups24,44 and the overall hydrophobicity43,45 of the phenolics. However, further studies are needed to clarify the detailed mechanisms behind this phenomenon.
Deactivation of specific enzyme activities of CTec3 by pretreatment-derived water-soluble fractions (WSs)
As mentioned previously, compared to enzyme inhibition, enzyme deactivation is usually indicated by the time-dependent loss of enzyme activities.46 These deactivators could interact with different functional groups on the enzyme/protein surface through covalent and/or exceptionally strong noncovalent bonds which sometimes remain even after complete protein breakdown.44,45,47 Since these steam pretreatment-derived WS fractions not only inhibited the initial rate of cellulose hydrolysis but also the extent of cellulose hydrolysis (cellulose conversion at 72 h) (Fig. 1), it was anticipated that certain enzymes were also deactivated by the inhibitory components during cellulose hydrolysis.
To try to determine if enzyme deactivation was occurring, the CTec3 preparation was first incubated with either the WS fractions or hydrolysis buffer for various times before its major enzyme activities were evaluated. As expected, the enzyme activities of CBH, EG, BG, and xylanase decreased gradually in a time-dependent manner after incubating with the WS fractions, which indicated that these WS fractions also contained soluble compounds that deactivated the major enzyme activities during the hydrolysis process (Fig. 4). Briefly, the SPP-WS fraction decreased the CBH activity dramatically after 72 h incubation (Fig. 4a and b). Although xylanase activity dropped gradually during the time course of incubation even without WS fractions, presumably due to thermal or shear inactivation, both the SPP-WS and the SPLP-WS fractions significantly accelerated the deactivation of xylanase activity (Fig. 4c and d). The WS fractions also deactivated EG and BG activities by 20–30% after 72 h incubation (Fig. 4e and f). In addition, it was apparent that the deactivating effect of the WS fractions was highly dependent on the type of biomass feedstocks. For example, unlike the effect of the SPP-WS fraction, the SPLP-WS fraction only slightly decreased the CBH activity even after 72 h incubation (Fig. 4a). These results indicated that the major enzyme components (such as CBH, xylanase, EG and BG) were indeed deactivated, to a certain extent, when incubated with the WS fractions derived from pretreatment.
 |
| Fig. 4 Effect of SPLP/SPP-WS fractions on the residual enzyme activities of (a, b) CBH, (c, d) xylanase, (e, f) EG and (g, h) BG after 72 h incubation at 50 °C. Controls were performed by incubating enzymes with buffer under the same conditions. The enzyme was incubated with the inhibitors for a certain time at 50 °C and 200 rpm. 100% EG, CBH, BG, and xylanase activities corresponded to 6, 2, 15 and 14 U per mg protein respectively. These values measured in the absence of inhibitors were used as a reference to calculate the loss of enzyme activities due to the presence of the possible inhibitors. | |
It is worth noting that the CBH activity (the major enzyme activities in most cellulase preparations) was particularly inhibited by the pretreatment derived WS fractions, and the extent of deactivation was also correlated well with the extent of WS inhibition of overall cellulose hydrolysis observed in our previous study.19 Thus, the deactivation of CBH activity was likely to be one of the major reasons for the slowdown of cellulose hydrolysis at the later stages of enzymatic hydrolysis. The xylanase deactivation might cause the significant decrease in the cellulose hydrolysis of pretreated substrates which contain higher amounts of xylan (Fig. 1b). Interestingly, the enzyme deactivation was not in line with the initial cellulose hydrolysis. It is clear that at 24 h, the CBH activity was decreased by around 40% (Fig. 4d) while the initial cellulose hydrolysis at 24 h in the presence of WS was only slightly inhibited compared with the control (Fig. 1b). This is likely to be related to the concentration of hydrolyzed cellulosic substrates: higher substrate concentration could alleviate the inhibitory effect of soluble compounds on cellulases when catalyzing cellulose. With increase of hydrolysis time, as less substrates are available for cellulases, the cellulases tend to bind to inhibitors more preferably.
Identifying the major inhibitors (sugars and phenolics) that account for the deactivating effect of the water-soluble fractions (WSs) on the major enzyme activities
Since the WS fractions derived from the pretreated biomass greatly deactivated the major enzyme activities within CTec3 preparation (Fig. 4), we next want to assess which of the major soluble compounds (sugars and phenolics) within WS fractions contributed to such an enzyme deactivation. When the deactivating effects of sugars and phenolic components on the major enzyme activities were assessed, it appeared that the pretreatment-derived phenolics were the main reasons for deactivating the major enzyme activities after 72 hours of cellulose hydrolysis (Fig. 5). Briefly, the activated carbon treated WS fractions (SPLP/SPP-WS-5% AC) showed almost no deactivating effect as compared to the original SPP-WS and SPLP-WS fractions, which indicated that the selective removal of phenolics from these WS fractions could alleviate their enzyme deactivation effects, while on the other hand, the monomeric sugars within both WS fractions showed no effects on enzyme deactivation even after 72 hours incubation (Fig. 5a and b). Once again, the enzyme deactivation effect was also highly dependent on the nature of the phenolics, where the phenolics within the SPP-WS fraction had much stronger deactivation effects than the phenolics within the SPLP-WS fraction (Fig. 5). This could be due to the higher concentration of phenolics present in the SPP-WS fraction.
 |
| Fig. 5 Influence of monomeric sugars and activated carbon (AC) treated water-soluble fractions from (a) steam pretreated lodgepole pine (SPLP-WS) and (b) poplar (SPP-WS) on enzyme deactivation within 72 h incubation. 5% activated carbon (AC) was used to treat the water-soluble fractions to selectively remove phenolics from the WS fractions (SPLP/SPP-WS-5% AC). The compositions of monomeric sugars were same as those of the corresponding WS fractions. 100% EG, CBH, BG, and xylanase activities corresponded to 6, 2, 15 and 14 U per mg protein respectively. These values, measured in the absence of possible inhibitors were used as a reference to calculate the loss of enzyme activities due to the presence of possible inhibitors. | |
Since the activated carbon treatment could selectively remove the more hydrophobic phenolics48 and their removal significantly alleviated the enzyme deactivation effects of WS fractions (Fig. 5), it appeared that these more hydrophobic phenolics in the WS fraction are the major inhibitory compounds, which cause severe enzyme deactivation during cellulose hydrolysis. Although the specific inhibition mechanism of the phenolics was not determined, the work reported here suggested that there might be a possibility of mitigating the inhibitory effect of the phenolics by modifying the upstream pretreatment process to produce less inhibitory (less hydrophobic) phenolics.
Conclusions
In the work reported here, we found that the pretreatment-derived inhibitors restricted the efficient enzymatic hydrolysis of various pretreated woody biomasses, during whole slurry hydrolysis where the water-soluble and water-insoluble fractions derived from pretreatment have to be combined together. The inhibition of the main enzyme components within the latest commercial cellulase preparations was the major cause for the strong inhibition of cellulose hydrolysis. The inhibition of the major enzyme activities by WSs contributed more significantly to the extent of hydrolysis than to the initial rate of hydrolysis. It appeared that BG and CBH activities were mainly inhibited by sugars (glucose) and phenolics respectively. Phenolics were the major enzyme deactivators that resulted in a significant irreversible loss of CBH and xylanase activities, in a time-dependent manner. The deactivation effects of phenolics were also highly dependent on the origin of the biomass and the type/concentration of the phenolic components.
Conflicts of interest
There are no conflicts to declare.
Acknowledgements
We would like to thank Novozymes (Sarah Teter) for their generous gift of cellulase enzyme mixtures and Natural Science Foundation of Jiangsu Province (No. BK20170832) and NSERC for funding support.
References
- S. Brethauer and M. H. Studer, Chim. Int. J. Chem., 2015, 69, 572–581 CrossRef CAS PubMed.
- R. Parajuli, T. Dalgaard, U. Jørgensen, A. P. S. Adamsen, M. T. Knudsen, M. Birkved, M. Gylling and J. K. Schjørring, Renewable Sustainable Energy Rev., 2015, 43, 244–263 CrossRef CAS.
- G. Fiorentino, M. Ripa and S. Ulgiati, Biofuels, Bioprod. Biorefin., 2017, 11, 195–214 CrossRef CAS.
- S. Sun, S. Sun, X. Cao and R. Sun, Bioresour. Technol., 2016, 199, 49–58 CrossRef CAS PubMed.
- L. J. Jönsson and C. Martín, Bioresour. Technol., 2016, 199, 103–112 CrossRef PubMed.
- L. J. Jönsson, B. Alriksson and N.-O. Nilvebrant, Biotechnol. Biofuels, 2013, 6, 16 CrossRef PubMed.
- H. Rasmussen, D. Tanner, H. R. Sørensen and A. S. Meyer, Green Chem., 2017, 19, 464–473 RSC.
- M. Kellock, J. Rahikainen, K. Marjamaa and K. Kruus, Bioresour. Technol., 2017, 232, 183–191 CrossRef CAS PubMed.
- A. Djioleu and D. J. Carrier, ACS Sustainable Chem. Eng., 2016, 4, 4124–4130 CrossRef CAS.
- K. Rajan and D. J. Carrier, ACS Sustainable Chem. Eng., 2016, 4, 3627–3633 CrossRef CAS.
- L. Murphy, C. Bohlin, M. J. Baumann, S. N. Olsen, T. H. Sørensen, L. Anderson, K. Borch and P. Westh, Enzyme Microb. Technol., 2013, 52, 163–169 CrossRef CAS PubMed.
- E. Ximenes, Y. Kim, N. Mosier, B. Dien and M. Ladisch, Enzyme Microb. Technol., 2011, 48, 54–60 CrossRef CAS PubMed.
- J. Shi, M. A. Ebrik, B. Yang, R. J. Garlock, V. Balan, B. E. Dale, V. Ramesh Pallapolu, Y. Y. Lee, Y. Kim, N. S. Mosier, M. R. Ladisch, M. T. Holtzapple, M. Falls, R. Sierra-Ramirez, B. S. Donohoe, T. B. Vinzant, R. T. Elander, B. Hames, S. Thomas, R. E. Warner and C. E. Wyman, Bioresour. Technol., 2011, 102, 11080–11088 CrossRef CAS PubMed.
- J. Hu, V. Arantes, A. Pribowo and J. N. Saddler, Biotechnol. Biofuels, 2013, 6, 112 CrossRef CAS PubMed.
- Y. Kim, E. Ximenes, N. S. Mosier and M. R. Ladisch, Enzyme Microb. Technol., 2011, 48, 408–415 CrossRef CAS PubMed.
- A. Tejirian and F. Xu, Enzyme Microb. Technol., 2011, 48, 239–247 CrossRef CAS PubMed.
- R. Bura, R. J. Bothast, S. D. Mansfield and J. N. Saddler, Appl. Biochem. Biotechnol., 2003, 106, 319–335 CrossRef.
- A. Boussaid, J. Robinson, Y. Cai, D. J. Gregg and J. N. Saddler, Biotechnol. Bioeng., 1999, 64, 284–289 CrossRef CAS PubMed.
- R. Zhai, J. Hu and J. N. Saddler, ACS Sustainable Chem. Eng., 2016, 4, 3429–3436 CrossRef CAS.
- B. Starcher, Anal. Biochem., 2001, 292, 125–129 CrossRef CAS PubMed.
- T. M. Wood and K. M. Bhat, Methods Enzymol., 1988, 160, 87–112 CAS.
- M. V. Deshpande, K.-E. Eriksson and L. Göran Pettersson, Anal. Biochem., 1984, 138, 481–487 CrossRef CAS PubMed.
- G. L. Miller, Anal. Chem., 1959, 31, 426–428 CrossRef CAS.
- A. Berlin, M. Balakshin, N. Gilkes, J. Kadla, V. Maximenko, S. Kubo and J. Saddler, J. Biotechnol., 2006, 125, 198–209 CrossRef CAS PubMed.
- Y.-H. Percival Zhang, M. E. Himmel, J. R. Mielenz, Y. P. Zhang, M. E. Himmel and J. R. Mielenz, Biotechnol. Adv., 2006, 24, 452–481 CrossRef CAS PubMed.
- P. Biely, D. Mislovičová and R. Toman, Anal. Biochem., 1985, 144, 142–146 CrossRef CAS PubMed.
- F. Shen, J. Hu, Y. Zhong, M. L. Y. Liu, J. N. Saddler and R. Liu, Biomass Bioenergy, 2012, 41, 157–164 CrossRef CAS.
- J. B. Kristensen, C. Felby and H. Jørgensen, Appl. Biochem. Biotechnol., 2009, 156, 127–132 CrossRef PubMed.
- M.-J. Yu, S.-H. Yoon and Y.-W. Kim, Enzyme Microb. Technol., 2016, 93, 150–156 CrossRef PubMed.
- H. Jørgensen and M. Pinelo, Biofuels, Bioprod. Biorefin., 2017, 11, 150–167 CrossRef.
- J. M. D. Paye, A. Guseva, S. K. Hammer, E. Gjersing, M. F. Davis, B. H. Davison, J. Olstad, B. S. Donohoe, T. Y. Nguyen, C. E. Wyman, S. Pattathil, M. G. Hahn and L. R. Lynd, Biotechnol. Biofuels, 2016, 1–13 Search PubMed.
- N. Cruys-Bagger, K. Alasepp, M. Andersen, J. Ottesen, K. Borch and P. Westh, J. Phys. Chem. B, 2016, 120, 5591–5600 CrossRef CAS PubMed.
- N. K. Morgan, A. Wallace, M. R. Bedford and M. Choct, Carbohydr. Polym., 2017, 167, 290–296 CrossRef CAS PubMed.
-
H. J. Smith and C. Simons, Enzymes and Their Inhibition Drug Development, CRC Press, New York, 2005 Search PubMed.
- M. E. Atreya, K. L. Strobel and D. S. Clark, Biotechnol. Bioeng., 2016, 113, 330–338 CrossRef CAS PubMed.
- J. P. Olsen, K. Alasepp, J. Kari, N. Cruys-Bagger, K. Borch and P. Westh, Biotechnol. Bioeng., 2016, 113, 1178–1186 CrossRef CAS PubMed.
- S. Kuusk and P. Väljamäe, Biotechnol. Biofuels, 2017, 10, 7 CrossRef PubMed.
- A. Nakamura, T. Tsukada, S. Auer, T. Furuta, M. Wada, A. Koivula, K. Igarashi and M. Samejima, J. Biol. Chem., 2013, 288, 13503–13510 CrossRef CAS PubMed.
- M. Srisodsuk, T. Reinikainen, M. Penttilä and T. T. Teeri, J. Biol. Chem., 1993, 268, 20756–20761 CAS.
- C. Pascal, F. Paté, V. Cheynier and M. Delsuc, Biopolymers, 2009, 91, 745–756 CrossRef CAS PubMed.
- C. M. Payne, M. G. Resch, L. Chen, M. F. Crowley, M. E. Himmel, L. E. Taylor, M. Sandgren, J. Ståhlberg, I. Stals and Z. Tan, Proc. Natl. Acad. Sci., 2013, 110, 14646–14651 CrossRef CAS PubMed.
- R. Kont, J. Kari, K. Borch, P. Westh and P. Väljamäe, J. Biol. Chem., 2016, 291, 26013–26023 CrossRef CAS PubMed.
- S. Nakagame, R. P. Chandra, J. F. Kadla and J. N. Saddler, Biotechnol. Bioeng., 2011, 108, 538–548 CrossRef CAS PubMed.
- L. Qin, W.-C. Li, L. Liu, J.-Q. Zhu, X. Li, B.-Z. Li and Y.-J. Yuan, Biotechnol. Biofuels, 2016, 9, 70 CrossRef PubMed.
- Y. Wang, A. P. Singh, W. J. Hurst, J. A. Glinski, H. Koo and N. Vorsa, J. Agric. Food Chem., 2016, 64, 2190–2199 CrossRef CAS PubMed.
- T. S. Maurer and H.-L. L. Fung, AAPS PharmSci, 2000, 2, 68–77 CrossRef.
-
R. B. Silverman and M. W. Holladay, Org. Chem. Drug Des. Drug Action, 2014, pp. 207–274 Search PubMed.
- A. Dąbrowski, P. Podkościelny, Z. Hubicki and M. Barczak, Chemosphere, 2005, 58, 1049–1070 CrossRef PubMed.
|
This journal is © The Royal Society of Chemistry 2018 |