Efficient photoelectrochemical water oxidation enabled by an amorphous metal oxide-catalyzed graphene/silicon heterojunction photoanode†
Received
17th October 2017
, Accepted 19th December 2017
First published on 20th December 2017
Abstract
Silicon is a promising photoelectrochemical (PEC) material owing to its earth abundance, high carrier mobility and narrow bandgap. However, a bare Si photoelectrode is prone to photocorrosion and its photovoltage is affected by surface states on the electrode in PEC measurements. In the present work, a simple and inexpensive method for the construction of a Si/graphene heterojunction is presented. The high barrier at the solid/solid junction results in an open-circuit voltage of 490 mV, making it an excellent heterojunction light absorber to generate a substantial photovoltage for PEC water oxidation. A TiO2 thin layer deposited on the Si/graphene structure is effective to protect the heterojunction from the electrolyte while favoring the interfacial charge transport from the buried junction. The introduction of a FeNiCoOx co-catalyst thin film onto the TiO2 protected heterojunction yields a high photocurrent density of ∼19 mA cm−2 at an applied potential of 1.5 V vs. RHE in 1.0 M NaOH solution under one sun simulated solar illumination. In contrast to the unprotected Si/graphene electrode, the activity of the TiO2 protected photoanode is sustained for several hours in an alkaline electrolyte. This work clearly reveals the important role of graphene in the performance improvement of the Si-based photoanodes and sheds light on the introduction of novel materials onto silicon-based electrodes to achieve higher photovoltages for efficient PEC reactions.
Introduction
The increasing global energy demand and associated environmental concerns have prompted tremendous interest in the utilization of renewable energies especially solar energy, to decrease the reliance on fossil fuels. Photoelectrochemical (PEC) water splitting offers a means to convert solar energy to storable chemical fuels and overcome the limitation imposed by the diurnal and intermittent nature of sunlight.1,2 Metal oxides and nitrides, such as TiO2, Fe2O3, WO3, BiVO4, and Ta3N5, have been extensively studied as promising photoanodes for water oxidation owing to their low cost and good chemical stability in appropriate electrolytes.3,4 These semiconducting materials are expected to achieve a high photovoltage due to their large band gaps (>2 eV); however, their efficiencies are limited by their relatively large band gaps and/or modest electronic conductivities. Si is an excellent solar material with a narrow band gap that is capable of capturing a large portion of the solar spectrum. However, Si can be corroded in aqueous alkaline solutions at high oxidation potentials during the water oxidation half-reaction.5,6 In addition, the surface states associated with Fermi-level pinning that may occur at the Si/liquid interface may limit the attainable photovoltage of Si-based photoanodes.7,8 To overcome these limitations, surface deposition of metal oxides (MnO,9 CoOx,10 NiOx,11,12 and TiO2/co-catalyst13–15) and metals (Co16 and Ni17–19) on n-Si has been proposed to improve the performances of Si-based photoanodes. The metal oxides and metals on Si can not only improve the stability of Si photoanodes, but also serve as the oxygen evolution catalyst. Moreover, the overlayer on Si may passivate the interface states responsible for the Fermi level pinning and reduce recombination loss in heterojunctions, so that an improved photovoltage is achieved. However, in many cases, the performance of a photoanode including photovoltage, photocurrent and stability, is sensitive to the thickness of the metal oxides or metals.9,14–19 The trade-off between film thickness, corrosion protection, and electrical conductivity should be taken into consideration carefully for the design of high performance photoanodes. Besides the n-Si/protective overlayer structures, the combination of a p+–n Si substrate and a thin catalytic layer offers a reliable composite photoelectrode that possesses the advantages of a buried junction and a stable water oxidation operation.20–22 Nonetheless, the use of the p+–n junction introduces extra processing steps and complexity, so that the fabrication of the junction becomes more complicated than the growth of a light absorber itself.23 In this regard, developing a low-cost, reproducible and scalable method for the construction of a Si-based buried heterojunction towards high performance water oxidation is highly desirable.
An ideal overlayer on Si to form an efficient heterojunction should be chemically and electrochemically stable in electrolytes, provide high optical transparency, allow for efficient charge transport, and be deposited easily on the Si. Graphene is a two-dimensional material that can be transferred on arbitrary surfaces and has low resistance, high optical transparency, and excellent stability.24 Thus, graphene offers great opportunity in the development of semiconductor/graphene Schottky solar cells due to its unique physical properties and simple processing steps.25–28 In comparison to polycrystalline graphene films, a graphene micro-net exhibits a better mechanical performance and a tunable light transparency and conductivity,26–28 thus making the graphene micro-net a suitable catalyst support and transparent electrode for solar energy conversion applications. An n-Si/graphene structure can be prepared by a simple transfer method and the configuration produces a built-in field across the Si/graphene interface. The maximum achievable barrier height (ΦB) of the structure is defined by the difference between the work functions of n-Si (∼4.3 eV) and graphene (∼4.8 eV),26 which provides a high photovoltage for PEC applications (Fig. 1b). Although Si/graphene structures have been used for ferrocyanide and bromine oxidation,29,30 their direct operation in solar-driven water oxidation was not achieved due to their poor stabilities in electrolyte solutions and the sluggish kinetics of the oxygen evolution reaction (OER).
 |
| Fig. 1 (a) Schematic structure of the Si/graphene/TiO2/FeNiCoOx photoanode. (b) Energy band diagram of the Si/graphene heterojunction. (c) FE-SEM image of the graphene micro-net transferred onto a Si substrate. (d) Transmittance and (e) Raman spectra of a graphene micro-net transferred onto a quartz glass. The inset in (d) is the optical image of the graphene micro-net structure on quartz glass. (f) Height profile along the lines in the inset of (d). (g) Optical images of the Si/graphene/TiO2/FeNiCoOx structure. | |
In the present work, we explored the construction of a buried heterojunction on n-Si with a multilayered graphene micro-net and its application in improving the performance of Si-based photoanodes for the water oxidation half-reaction. We introduced a simple method to enable their applications to the PEC water oxidation in electrolyte solutions. A thin TiO2 layer prepared by atomic layer deposition (ALD) was used to isolate the heterojunction from the electrolyte while maintaining an efficient hole transport. It was found that the graphene micro-net was capable of forming an effective built-in field with Si so that the Si/graphene micro-net solid solar cell produced an open-circuit voltage of 490 mV, providing a large driving force for carrier separation in the OER. In conjunction with a TiO2 thin layer and FeNiCoOx electrocatalyst, the Si-based photoanode exhibited a high photocurrent of ∼19 mA cm−2 at an applied potential of 1.5 V vs. reverse hydrogen electrode (RHE) in 1.0 M NaOH solution using an AM 1.5G solar simulator (100 mW cm−2). It is noted that the decoupling of the catalytic layer from the underlying heterojunction makes it possible to further improve the device performance by optimizing the different components independently.
Results and discussion
Characterization of the Si/graphene micro-net/TiO2/FeNiCoOx structures
Fig. 1a, S1 and S2† illustrate the schematic diagram and fabrication process of the Si/graphene/TiO2/FeNiCoOx photoanode, respectively. Similar to the previous work,31,32 the Si/graphene heterojunction was prepared by transferring a graphene micro-net on a Si substrate (Fig. 1c). The micro-net graphene film was grown by the ambient pressure chemical vapor deposition (APCVD) method using copper meshes as substrates. A freestanding micro-net graphene film was then obtained by detaching the graphene from the copper mesh in 0.5 mol L−1 FeCl3 solution. After the copper mesh dissolved completely, the micro-net graphene was further rinsed in dilute hydrochloric acid (0.1 mol L−1) for 4 h to completely remove the residual ions. The free standing graphene micro-net was directly transferred on the top of a substrate, thus totally covering the Si window by forming a heterojunction with Si. The n-type Si and graphene layers produce a Schottky junction across the Si/graphene interface that enhances carrier separation (Fig. 1b). The charge carriers can move across the junction by tunneling.33 However, the graphene layer was easily detached from the Si substrate in the electrolyte solution. Therefore, a TiO2 thin layer was deposited on the surface of the Si/graphene structure as a protective layer. The surface of the Si/graphene/TiO2 was then modified with a FeNiCoOx thin film, an efficient and highly active oxygen evolution co-catalyst.34,35 The graphene micro-net exhibited an ordered alignment of openings with a width of ∼100 μm for light transmission (the inset of Fig. 1d), through which light transparency up to 80% in the range of 600 to 1200 nm was achieved (Fig. 1d). The ratio of area covered by the graphene micro-net to the Si surface is estimated to be 84 ± 1%, so that a large contact area between Si and graphene is achieved and an efficient Schottky junction for carrier separation can be formed.
The Raman spectra indicated that the polycrystalline graphene micro-net was a multilayered configuration (ID/IG ≈ 0.16, I2D/IG ≈ 0.61) and the thickness of the multilayer graphene was measured to be ∼20–40 nm (Fig. 1e and f). Two conformal thin TiO2 layers of 10 nm and 50 nm formed by ALD were respectively deposited to prevent the photocorrosion of the Si substrate (Fig. S3 and S4†). The amorphous FeNiCoOx co-catalyst film was prepared by spin-coating appropriate metal–organic precursor complexes on the TiO2 protected Si/graphene structure, followed by photolysis under UV light, and annealing at 100 °C for 1 h.34,35 The microscopy and SEM images of the as-prepared amorphous FeNiCoOx co-catalyst film revealed a complete coverage of the substrate with the catalyst and a smooth surface morphology. The thickness of the film was measured to be approximately 450 nm (Fig. S5†). Fig. 1g shows the optical microscope image of the Si-based photoanode after the formation of the heterojunction and deposition of the co-catalyst.
Structural, electrocatalytic and optical properties of FeNiCoOx thin films
The constituents of the co-catalyst film were identified by X-ray spectroscopy (XPS) survey scans to be Fe, Ni, Co, O, and C. Energy dispersive X-ray spectroscopy (EDX) analysis indicated that the elemental composition of the film was in good agreement with the corresponding solution stoichiometry (Fe
:
Ni
:
Co = 1
:
1
:
1). Fig. 2a–c show the high resolution XPS spectra of the Fe-2p, Co-2p, and Ni-2p peaks of the FeNiCoOx film. The XPS survey scans acquired on FeNiCoOx, FeNiOx and FeCoOx are shown in Fig. S6.† The as-prepared amorphous FeNiCoOx film exhibited a main peak of Fe 2p3/2 at the binding energy of 712.0 ± 0.1 eV that was ascribed to its FeOOH phase.36,37 The Ni 2p3/2 peak centered at 856.1 ± 0.1 eV indicates that the nickel was in the form of Ni(OH)2.38,39 The Co 2p region of the XPS spectrum resembles those of Co3O4 and Co(OH)2 phases. These two phases can be distinguished by the shape and energy of the Co 2p3/2 satellite band. The prominent Co 2p satellite ascribed to Co(OH)2, but not Co3O4,35,40,41 suggests that the film mostly consisted of Co(OH)2, rather than Co3O4.
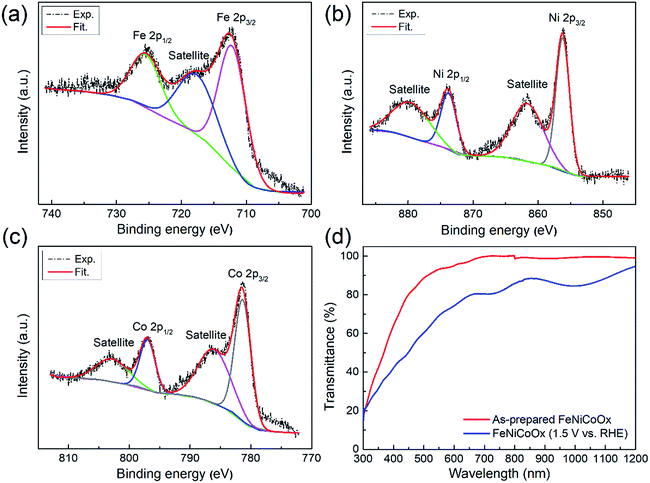 |
| Fig. 2 (a–c) High resolution XPS spectra of the Fe-2p, Co-2p and Ni-2p peaks of the FeNiCoOx film. (d) Transmittance obtained for the FeNiCoOx film. | |
The electrocatalytic performance of the FeNiCoOx film on FTO towards water oxidation was examined by CV in 1.0 M NaOH solution. Moreover, the electrooxidation characteristics of water on the FeCoOx and FeNiOx films were also investigated for comparison purposes (Fig. S7a†). The ternary mixture of the FeNiCoOx film exhibited a precatalytic oxidation wave at an applied potential of 1.38 V vs. RHE and a reductive wave at an applied potential of 1.32 V vs. RHE (Fig. 3). The precatalytic oxidation process of the film can be attributed to the oxidation of Ni(OH)2 to NiOOH and the reductive peak can be assigned to the regeneration of Ni(OH)2.35,42 The FeNiCoOx film exhibits excellent OER activity, as demonstrated by the low Tafel slope of 56 ± 2 mV dec−1 and small overpotential of 290 ± 3 mV to reach a current density of 10 mA cm−2 (without iR correction), as indicated in Fig. 3. The iR-corrected linear scan voltammetry (LSV) curves are shown in Fig. S7c.† For the FeCoOx film, an overpotential of 340 ± 4 mV was needed to reach an OER current of 10 mA cm−2, much higher than that of the FeNiCoOx film. The FeNiOx film exhibited a similar overpotential to FeNiCoOx, but a lower OER activity in a more anodic potential range (Fig. S7a†). The overpotential of the FeNiCoOx film is superior to those of other catalysts, such as Co3O4 (>370 mV),43 CoFe2O4 (378 mV),44 NiCo2O4 (320 mV),45 NiOx (>400 mV) and NiFeOx (∼350 mV),46 suggesting its excellent OER activity. The annealing of the FeNiCoOx film at temperatures higher than 100 °C led to an anodic shift of the precatalytic oxidation wave and a decreased OER activity, suggesting that the higher temperature annealing might reduce the electrochemically active sites for water oxidation (Fig. S7e and Table S1†). The variation of the film thickness is shown in Fig. S7f and Table S2.† Therefore, the FeNiCoOx film can provide a desirable balance between catalytic properties as evidenced by the low overpotential and the small Tafel slope for water oxidation.
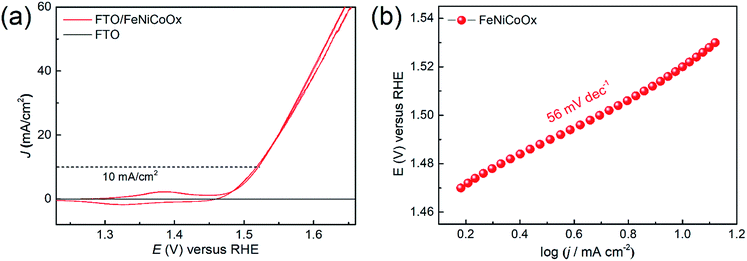 |
| Fig. 3 (a) Cyclic voltammograms (CVs) of the FeNiCoOx film. Data were collected at a scan rate of 10 mV s−1 in 1.0 M NaOH, without iR correction. (e) Tafel plot obtained for the FeNiCoOx film. | |
As shown in Fig. 2d for the transmission spectrum of the FeNiCoOx film, the as-prepared FeNiCoOx film was almost transparent in the range of 500 to 1200 nm. When a potential was applied on the film, a color change occurred due to the redox event. To analyze the change in transmittance of FeNiCoOx film samples caused by the oxidation reaction, the film samples were operated at an applied voltage in NaOH electrolyte for 10 min and then taken out from the cell, rinsed in distilled water and dried in a nitrogen stream. After the cleaning process, the transmittance of the samples was immediately measured. It was found that the film still allowed a large portion of light to be transmitted even after electrochemical operation at a positive potential of 1.5 V vs. RHE (Fig. 2d and S7d†). Therefore, integrating the FeNiCoOx co-catalyst with a high OER activity and good optical transparency on the Si/graphene/TiO2 structure offers an attractive strategy to achieve a high-performance Si-based photoanode for water splitting.
PEC properties of Si/graphene micro-net/TiO2/FeNiCoOx photoanodes
The PEC current density vs. applied potential properties of the FeNiCoOx modified Si-based photoanodes were subsequently characterized in aqueous 1.0 M NaOH (pH 13.6) using an AM 1.5G solar simulator (100 mW cm−2). The Si/FeNiCoOx photoelectrode exhibited a very low photocurrent under illumination (Fig. 4a), which might be attributed to the poor charge separation at the Si/catalyst and catalyst/electrolyte interfaces during the OER. The low photocurrent suggested the existence of deleterious surface states on Si that functioned as recombination centers reducing the open-circuit voltage by pinning the Fermi level to the surface states, and thus limiting the photocurrent.7,8 This hypothesis is supported by the open-circuit potential (OCP) measurement of the photoelectrode. The OCP technique is an effective means to measure the potential that is not affected by kinetic factors.47Fig. 4c shows the photovoltage of the photoanodes, which was obtained by the difference of OCPs in the dark and under illumination (Fig. S8†). The OCP of the Si/FeNiCoOx photoelectrode varied only by 100 mV when irradiation changed from dark to illumination, much less than that of the Si/Ni thin layer photoanode (300 mV) reported in the literature.17 Considering that the layered M(OH)2/MOOH (M = Fe, Ni, Co) structures allow the intercalation of water due to the weak interaction of hydroxide layers,42 the co-catalyst film cannot isolate the Si from the electrolyte and thus the catalyst overlayer is incapable of passivating the surface states on the Si surface, leading to a low photovoltage at the solid/liquid interface.
 |
| Fig. 4 (a) Current–potential curves of Si/FeNiCoOx, Si/graphene and Si/graphene/FeNiCoOx photoanodes in 1 M NaOH under chopped AM 1.5G light illumination. (b) Current–potential curves of Si/TiO2/FeNiCoOx and Si/graphene/TiO2/FeNiCoOx photoanodes in the dark and under AM 1.5G light illumination. Scan rate, 10 mV s−1. (c) Photovoltage and (d) Nyquist plot of Si/FeNiCoOx, Si/graphene/FeNiCoOx and Si/graphene/TiO2/FeNiCoOx photoanodes. Nyquist plot measurements were carried out at 1.23 V vs. RHE by varying the frequency between 100 kHz and 0.1 Hz under AM 1.5G light illumination. | |
To eliminate the dependence of photovoltage on the Si/electrolyte junction, a multilayered graphene film was introduced so that a solid heterojunction with the Si substrate was formed. The difference between the work functions of graphene and Si can generate a constant internal electric field at their interface, which is independent of the thermodynamic and kinetic properties of the semiconductor/liquid junction. To confirm this, the current–voltage (I–V) characteristics of the Si/graphene solid-state cell were tested under AM 1.5G light illumination (100 mW cm−2), as shown in Fig. S9.† The Si/graphene structure exhibited an open-circuit voltage (Voc) of 490 mV, indicating an efficient built-in field formed at the Si/graphene interface and could provide a substantial photovoltage for PEC water oxidation. The I–V characteristics of the Si/graphene structures annealed at different temperatures can be found in Fig. S9.† Compared with the Si/FeNiCoOx photoelectrode, the photocurrent of the Si/graphene electrode (Fig. 4a), even in the absence of co-catalyst, exhibited a moderate improvement, which was attributed to the efficient charge separation at the interface and the defects in the graphene layer that provided electrochemically active sites for water oxidation.48 However, the slowly increasing photocurrent indicates a slow reaction kinetics on the electrode surface due to the low catalytic activity of intrinsic defects on the graphene layer. The introduction of the FeNiCoOx co-catalyst film onto the Si/graphene heterojunction dramatically improved the PEC performance. As shown in Fig. 3a for the dark cyan curve, the Si/graphene/FeNiCoOx photoanode exhibited a precatalytic oxidation wave at 1 V vs. RHE, that was ascribed to the oxidation of the co-catalyst (M(OH)2 → MOOH), and a photocurrent of 17.5 mA cm−2 at 1.5 V vs. RHE. The difference between OCPs of the composite photoanode in the dark and under illumination was ∼400 mV (Fig. S8†), much greater than the photovoltage on the Si/FeNiCoOx photoelectrode (<100 mV). The significant improvements of photovoltage and photocurrent provided unambiguous evidence for the successful construction of a heterojunction on Si and the highly efficient OER reaction in the presence of the FeNiCoOx co-catalyst. However, although the graphene layer is robust against extreme pH values and harsh oxidative environments, the physical contact between the Si and graphene layer is fragile due to the corrosion at the interface, resulting in the detachment of the graphene layer from the Si substrate (Fig. S10†).
TiO2 is a well-known n-type semiconductor that can serve as a protective layer for unstable p-type photocathodes in the hydrogen evolution reaction (HER).49,50 In addition, the amorphous TiO2 with electronic defects has been used as a hole conduction overlayer on n-type semiconductors to achieve a stable OER operation against photocorrosion in alkaline solutions.13 Inspired by this concept, we deposited a thin n-type TiO2 protective layer on the Si/graphene heterojunction to isolate it from the electrolyte, while enabling an efficient hole transfer towards the electrode surface. With a 10 nm TiO2 layer deposited by ALD, the Si/graphene/TiO2/FeNiCoOx photoanode exhibited an onset potential of 1.0 V vs. RHE and a photocurrent of ∼19 mA cm−2 at 1.5 V vs. RHE. The OCP of the Si/graphene/TiO2/FeNiCoOx electrode under dark conditions was 1.21 V vs. RHE, indicating that its Fermi level was aligned with E(H2O/O2) and the charge transfer was due to the OER half reaction.51 Under illumination, the OCP of the electrode shifted to a more negative potential, resulting in a photovoltage of ∼420 mV that was greater than those of n-Si/Ni (300 mV)17 and n-Si/Al2O3/NiOx (247 mV)12 and comparable to those of Si/defective TiO2 (430 mV)13 and n-Si/SiOx/Co/CoOOH photoanodes (450 mV)16 reported in the literature. Furthermore, the photocurrent and stability of this structure show a significant enhancement over the Si/carbon nanotube/graphene photoanode,52 indicating that a rational combination between the intermediate layer, protective layer and catalytic layer is necessary for high performance water oxidation. These results suggest that a 10 nm TiO2 thin layer had no interference to the transfer of photogenerated holes from the heterojunction to the electrode surface, and could slightly improve photovoltage and photocurrent, possibly due to the fully passivated electrode surface. However, the thicker 50 nm TiO2 overlayer caused a moderate decrease in the photocurrent (Fig. S11a†). Therefore, a thin TiO2 layer should be adopted as the protection layer.
For comparison purposes, the PEC performances of Si/TiO2 (10 nm and 50 nm)/FeNiCoOx photoanodes were also determined (Fig. S11b and c†). The negligible photocurrents were achieved on these photoanodes, suggesting that the energy band diagram at the Si/TiO2 interface was unfavorable for hole transfer and the high photocurrent observed on the Si/graphene/TiO2/FeNiCoOx photoanode was produced by bridging the transport pathway of charge carriers through a graphene layer. Therefore, the introduction of graphene layer between Si and TiO2 in this work provided a simple but efficient method to construct a favorable buried junction for notably enhanced charge separation and transfer. The electrochemical impedance spectra (EIS) of Si/FeNiCoOx, Si/graphene/FeNiCoOx and Si/graphene/TiO2/FeNiCoOx photoanodes at 1.23 V vs. RHE under light illumination were also measured (Fig. 4d). It was found that the charge transfer resistance of Si/graphene/FeNiCoOx (∼400 Ω) and Si/graphene/TiO2/FeNiCoOx (∼330 Ω) photoanodes was much lower than that of Si/FeNiCoOx (∼1700 Ω) (Fig. S12 and Table S3†), corroborating the conclusion drawn by the PEC measurement that the introduction of the buried junction enhanced charge transfer.
The proposed schematic diagrams of the energy levels for the Si/FeNiCoOx and Si/graphene/TiO2/FeNiCoOx photoanodes are depicted in Fig. S13† to illustrate the interfacial band-edge energetics under open-circuit conditions. The Fermi levels of the photoanodes equilibrating with the electrolyte under dark conditions caused a band bending at the surface. Under illumination, the quasi-Fermi levels of the photoexcited electrons and holes split and flattened the band, giving rise to a photovoltage. Due to the permeable co-catalyst film and nonideality factors representing effects such as the surface states on the Si surface, the built-in potential of the Si/electrolyte solid/liquid junction is determined by the difference between the work function of Si and the surface state energy level. Therefore, a small band bending in Si was formed, which accounted for the low photovoltage (∼100 mV). In contrast, the introduction of the graphene layer and TiO2 protective layer isolated the Si substrate to form a solid/solid junction, resulting in an effective Si/graphene Schottky barrier. The barrier height is mainly defined by the difference between the work functions of the Si (∼4.3 eV) and graphene layer (∼4.8 eV),26 providing a large driving force for the PEC oxygen evolution. In this study, the measured photovoltage of the Si/graphene solid state solar cell (∼490 mV) is consistent with the expected value (∼500 mV), suggesting that an efficient buried junction can be formed at the Si/graphene interface. The introduction of the Si/graphene junction shifted the OCP of the photoanode to a more positive value that aligned with E(H2O/O2) in the dark, suggesting that a partial passivation of surface states reduced the Fermi level pinning effect, and thus enlarged the band bending formed at the interface. The higher photovoltage of 420 mV observed on the photoanode with Si/graphene heterojunction indicates that an efficient solid/solid junction was formed. The high built-in field across the interface is favorable to the charge separation and transfer toward the Co-catalyst/electrolyte interface.
Stability and IPCE measurements
Fig. 5a shows the stability of the Si/graphene/TiO2/FeNiCoOx photoanode at 1.5 V vs. RHE under AM 1.5G simulator irradiation. The photocurrent on the photoanode was maintained at 71% of the initial photocurrent after a 1 h operation and gradually decreased in a 6 h measurement (Fig. S14†), during which the fluctuation in the photocurrent was caused by the formation and detachment of oxygen bubbles. We analyzed the gaseous products and found that the ratios of the evaluated H2 and O2 were close to 2
:
1 and the faradic efficiency of the photoanode was ∼94% (Fig. S15†). After the stability test, the graphene micro-net and Co-catalyst were still observed on the Si substrate (Fig. S16†). Cracks formed on the catalyst film were attributed to the stress-induced crack formation during the PEC measurement.21 The Raman spectra further confirmed that the graphene layer remained on the Si substrate, and the presence of Ti peaks and the absence of the Si peak in the XPS spectrum revealed that the protected photoanode was highly tolerant to corrosion in the electrolyte (Fig. S16 and S17†). The SEM images of the samples subjected to the stability test indicate that the graphene and TiO2 layers were localized below the Co-catalyst film, as shown in Fig. S18.† The XPS spectra of the FeNiCoOx film after the stability test suggest that the Fe and Ni remained in their oxidation states of Ni(OH)2 and FeOOH (Fig. S19†). However, compared to the as-prepared sample, the Co 2p peaks after the stability test shifted to a lower binding energy and the intensities of satellite peaks significantly decreased, indicating the appearance of the Co3O4 phase during the PEC measurement.35,40,41 The decay of photocurrent on the photoanode might be attributed to the formation of cracks that reduced an effective catalyst coverage area.
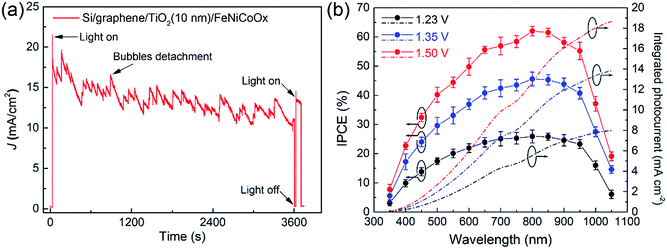 |
| Fig. 5 (a) Current–time curve (held at 1.5 V vs. RHE) of the Si/graphene/TiO2/FeNiCoOx photoanode measured in 1.0 M NaOH solution under AM 1.5G light illumination. (b) Wavelength dependence of IPCE measured at 1.23, 1.35 and 1.5 V vs. RHE for the photoanode (left axis). The integrated photocurrent (right axis) was obtained by integrating the IPCE and AM 1.5G spectrum. | |
Fig. 5b shows the wavelength dependent incident photo-to-current conversion efficiency (IPCE) of the photoanode at 1.23, 1.35 and 1.5 V vs. RHE. The photoanode exhibited IPCEs over 50% in the range of 600–950 nm under the bias of 1.5 V vs. RHE, indicating an efficient photoconversion in this region. Integration of the average IPCE with the solar AM 1.5G spectrum resulted in a photocurrent that matched well with the photocurrent obtained by LSV. Considering that the amorphous metal oxide catalyst films tended to form cracks on the surface and that the film transmittance was affected by the applied voltage during the PEC measurement, a more transparent and robust catalyst layer should improve light absorption and photocurrent. Therefore, a thin and conformal catalytic layer prepared by ALD21 is expected to further improve electrode stability and minimize light absorption loss in this system.
Conclusions
A Si/graphene/TiO2/FeNiCoOx photoanode has been prepared by directly transferring a multilayered graphene micro-net on a Si substrate, followed by the deposition of an ALD-TiO2 protective layer and a catalytic layer. The graphene layer formed a solid heterojunction with Si, which was independent of the thermodynamic and kinetic properties of the Si/liquid junction, affording a high photovoltage of 420 mV for effective charge separation. The ALD-TiO2 layer allowed an efficient and stable interfacial charge transport for hours under alkaline conditions. The integration of a FeNiCoOx co-catalyst film onto the surface of the Si/graphene/TiO2 electrode achieved a large photocurrent of 19 mA cm−2 and a high IPCE of 62.0 ± 1.5% (800 nm) at 1.5 V vs. RHE. The reported photoanode structure could be further improved by independent optimization of the catalytic layer and the underlying photovoltaic substrate. These results demonstrate that Si/graphene is an effective and powerful configuration to achieve a significant built-in field and sheds light on the development of a Si/novel material heterojunction with desired functions for high performance PEC water splitting.
Methods
Fabrication of the graphene micro-net
The graphene micro-net was prepared on a Cu mesh by atmospheric pressure chemical vapor deposition (APCVD).24–27 A typical fabrication process was performed as follows. A Cu mesh (120 mesh) with a size of 10 × 5 cm2 was cleaned sequentially in acetone and ethanol in an ultrasonic bath for 10 min, and placed at the center of a quartz tube. The quartz tube was heated to 1000 °C at 10 °C min−1 under an Ar (300 mL min−1) and a H2 (45 mL min−1) atmosphere. The H2 flow was stopped and the flow rate of Ar was set to 200 mL min−1. CH4 was then introduced into the quartz tube at a flow rate of 20 mL min−1 for 15 min to grow graphene. After the reaction, the Cu mesh was quickly cooled to room temperature, cut into small pieces (1 × 1 cm2), and etched in a FeCl3/HCl solution (FeCl3: 1 mol L−1; HCl: 0.5 mol L−1) to form a graphene micro-net.
Preparation of n-Si substrate and n-Si/graphene structures
Phosphorus-doped n-type monocrystalline Si wafer (ρ = 2–4 Ω cm, 3′′ diameter, 400 μm thickness) was used as the substrate to construct Si/graphene-based photoanodes. To prepare an n-Si substrate with a well-defined exposed area, a SiO2 layer with a thickness of 300 nm was thermally grown on a Si surface. A layer of photoresist was then spin-coated on the Si/SiO2 surface and patterned by irradiating the photoresist layer through a photomask. The Si/SiO2 substrate with the patterned photoresist was etched in acid until the Si surface was exposed. The remaining photoresist was then removed to form a Si substrate with an active area of 0.1 cm2 surrounded by a SiO2 insulating layer. So, all photoelectrodes in this study have the same active surface area of 0.1 cm2. The ohmic contact of the substrate was formed by sputtering 10 nm Ti and 50 nm Au on the backside of the wafer. Prior to the graphene transfer, the Si substrate was dipped in HF (10% v/v in deionized water) for 15 s to remove the native SiO2 layer, rinsed with deionized water and dried by argon gas. The as-prepared graphene was transferred onto the n-Si substrate to achieve a Si/graphene heterojunction.
Fabrication of Si/graphene micro-net solid-state cells
The free standing graphene micro-net was directly transferred on the top of the n-Si substrate, totally covering the Si window and thus forming a Schottky junction with Si. Micrometer-thick Ag paste was applied around graphene to enclose a nearly square window as the device active area (0.1 cm2). After that, the top of graphene (Ag paste) and the bottom of Si (Ti/Au) were wired as positive and negative electrodes to complete the fabrication process. The photovoltaic properties of the solar devices were measured under AM 1.5 illumination (100 mW cm−2). The current–voltage data were recorded with a Keithley 4200-SCS.
Atomic layer deposition of TiO2 thin films
To protect the Si/graphene structure, a TiO2 thin layer was deposited on its surface with an ALD system (Beneq ALD TFS200). The TiO2 layer was prepared at a substrate temperature of 200 °C using titanium tetrachloride and H2O as Ti and O precursors, respectively. TiO2 deposition of 220 and 1160 cycles produced conformal TiO2 thin films of approximately 10 and 50 nm, respectively.
Photochemical deposition of the FeNiCoOx amorphous co-catalyst
Iron(III) 2-ethylhexanoate (50% w/w in Mineral Spirits, Strem Chemicals), cobalt(II) 2-ethylhexanoate (65% w/w in Mineral Spirits, Alfa Aesar) and nickel(II) 2-ethylhexanoate (78% w/w in 2-ethylhexanoic acid, Strem Chemicals) were mixed in a 1
:
1
:
1 ratio, and dissolved in an appropriate amount of hexane to produce a total metal complex concentration of 7.5% w/w. The metal complex solution was spin-coated onto a fluorine-doped tin oxide (FTO) coated glass and the Si-based photoanode at 600 rpm for 10 s and 3000 rpm for 1 min (Easy coater 6, Schwan technology, China). The films were illuminated under UV light for 3 h. The photoelectrodes with the FeNiCoOx coating were annealed in an oven at 100 °C for 1 h and the FTO/FeNiCoOx sample was annealed at higher temperature (200 °C and 300 °C) for comparison purposes. FTO/FeNiOx and FTO/FeCoOx samples were prepared by the same procedure.
Preparation of electrodes
Silver paste was used to connect the ohmic contact on the back side of the Si electrode to a coiled tin-coated Cu wire that was protected by ethylene tetrafluoroethylene. The backside and edges of the Si electrode were insulated with epoxy gel against the electrolyte. The photograph of the insulated electrode can be found in Fig. S2.†
Electrochemical, photoelectrochemical and electrochemical impedance measurements
For the electrochemical tests, a three-electrode configuration was employed with amorphous metal oxides as the anodes, a Pt wire as the counter electrode, and an Ag/AgCl as a reference electrode. Cyclic voltammetry (CV) measurements were performed in a 1 M NaOH (pH 13.6) electrolyte, with a scan rate of 10 mV s−1. Potential corrections for the iR drop were performed using the series resistance obtained from impedance spectroscopy in a frequency range from 10 kHz to 0.01 Hz (amplitude 5 mV). The resistance was extracted from the x-intercept of the Nyquist plot at a high frequency and the resistance was consistent at multiple potential points. A CHI660E potentiostat (CH Instrument Inc.) was used to maintain the desired reported potentials and measure the current on the electrodes both in electrochemical and PEC measurements. All the PEC characterizations were carried out in the same three-electrode configuration and the Si-based photoanodes were used as the working electrode. The electrolyte (1 M NaOH) was stirred and purged with argon gas for 15 min before each measurement and during measurement. The current–potential curves were measured using an AM 1.5G solar simulator (Newport Oriel, 92250A-1000) with the illumination intensity set to 100 mW cm−2. The scan rate for the linear sweep voltammetry (LSV) was set to 10 mV s−1. For the photovoltage measurement, the electrolyte was saturated with O2 gas. The OCP values were collected upon reaching steady-state with constant stirring. The evolved hydrogen and oxygen gas during the solar water splitting was quantified by gas chromatography (GC2014, Shimadzu). The wavelength dependence of the incident photo-to-current conversion efficiency (IPCE) was measured under monochromatic light irradiation using a monochromator (LE-SP-XE Series Light Source Controller) coupled with a 1000 W Xe lamp under ambient conditions at room temperature (∼25 °C). A calibrated Si photodiode (Thorlabs, FDS1010-CAL, wavelength range 350–1100 nm, active area 10 × 10 mm2) with a known spectral response was used to measure the intensity of monochromatic light impinging on the sample. In order to carry out an accurate measurement, both the sample and the reference diode need to be included in the range of the incident light spot. Photocurrent spectra of samples were recorded with a 50 nm step in the range of 350–1050 nm in a three-electrode electrochemical cell with a quartz window. The IPCE at each wavelength was calculated by the equation IPCE% = [Jph(mA cm−2) × 1240]/[P(mW cm−2) × λ(nm)] × 100. The electrochemical impedance was measured under illumination at an applied potential of 1.23 V vs. RHE for the sweeping frequency range from 10 kHz to 0.01 Hz with an amplitude of 5 mV. The error bars for the related data were determined by taking the standard deviation of the data measured from at least five different samples under the same conditions.
Characterization
The morphologies were examined using a field emission scanning electron microscope (MERLIN VP Compact, Carl Zeiss, Germany). The X-ray diffraction (XRD) patterns were recorded on a diffractometer (SmartLab, Rigaku Co. Ltd, Japan) with Cu Kα radiation (1.540598 Å). X-ray photoelectron spectroscopy (XPS) analysis was performed on an ESCALab 250Xi (Thermo Scientific, USA) using an Al Kα X-ray source (energy 1486.6 eV, voltage 15 kV, current 10 mA, vacuum 5 × 10−6 Pa, power 150 W, pass energy 30 eV, energy step size 0.1 eV, and spot size 500 μm). The C 1s peak at 284.8 eV was used to calibrate the binding energy scale. A flood gun was used to overcome the charging effects. Spectra were analyzed using Thermo Scientific Avantage v5.934 software. Deconvolution and curve fitting of the spectra were performed using Xpspeak software (Version 4.1) employing a Gaussian–Lorentzian line shape function. The height of the graphene micro-net was measured with a Stylus profilometer (Dektak XT, Bruker). The transmittance of the catalyst and graphene film was measured with a Cary 5000 UV-vis-NIR spectrophotometer (Agilent Technologies, USA). The Raman spectra of the samples were recorded on a Raman microscope (HORIBA LabRAM HR Evolution) using 532 nm excitation.
Conflicts of interest
There are no conflicts to declare.
Acknowledgements
This work was supported by the National Science Foundation of China (51672150), and the China Postdoctoral Science Foundation (2016M600083 and 2015M571019).
Notes and references
- M. Grätzel, Nature, 2001, 414, 338–344 CrossRef PubMed.
- N. S. Lewis and D. G. Nocera, Proc. Natl. Acad. Sci. U. S. A., 2006, 103, 15729–15735 CrossRef CAS PubMed.
- Z. Li, W. Luo, M. Zhang, J. Feng and Z. Zou, Energy Environ. Sci., 2013, 6, 347–370 CAS.
- K. Sivula and R. Van De Krol, Nat. Rev. Mater., 2016, 1, 15010 CrossRef CAS.
- R. Liu, Z. Zheng, J. Spurgeon and X. Yang, Energy Environ. Sci., 2014, 7, 2504–2517 CAS.
- M. F. Lichterman, K. Sun, S. Hu, X. Zhou, M. T. McDowell, M. R. Shaner, M. H. Richter, E. J. Crumlin, A. I. Carim, F. H. Saadi, B. S. Brunschwig and N. S. Lewis, Catal. Today, 2016, 262, 11–23 CrossRef CAS.
- A. J. Bard, F. R. F. Fan, A. S. Gioda, G. Nagasubramanian and H. S. White, Faraday Discuss. Chem. Soc., 1980, 70, 19–31 RSC.
- T. Skotheim, L. G. Petersson, O. Inganäs and I. Lundström, J. Electrochem. Soc., 1982, 129, 1737–1741 CrossRef CAS.
- N. C. Strandwitz, D. J. Comstock, R. L. Grimm, A. C. Nichols-Nielander, J. Elam and N. S. Lewis, J. Phys. Chem. C, 2013, 117, 4931–4936 CAS.
- X. Zhou, R. Liu, K. Sun, K. M. Papadantonakis, B. S. Brunschwig and N. S. Lewis, Energy Environ. Sci., 2016, 9, 892–897 CAS.
- K. Sun, N. Park, Z. Sun, J. Zhou, J. Wang, X. Pang, S. Shen, S. Y. Noh, Y. Jing, S. Jin, P. K. L. Yu and D. Wang, Energy Environ. Sci., 2012, 5, 7872–7877 CAS.
- M.-J. Park, J.-Y. Jung, S.-M. Shin, J.-W. Song, Y.-H. Nam, D.-H. Kim and J.-H. Lee, Thin Solid Films, 2016, 599, 54–58 CrossRef CAS.
- S. Hu, M. R. Shaner, J. A. Beardslee, M. Lichterman, B. S. Brunschwig and N. S. Lewis, Science, 2014, 344, 1005–1009 CrossRef CAS PubMed.
- A. G. Scheuermann, J. D. Prange, M. Gunji, C. E. Chidsey and P. C. McIntyre, Energy Environ. Sci., 2013, 6, 2487–2496 CAS.
- Y. W. Chen, J. D. Prange, S. Dühnen, Y. Park, M. Gunji, C. E. D. Chidsey and P. C. McIntyre, Nat. Mater., 2011, 10, 539–544 CrossRef CAS PubMed.
- J. C. Hill, A. T. Landers and J. A. Switzer, Nat. Mater., 2015, 14, 1150–1155 CrossRef CAS PubMed.
- M. J. Kenney, M. Gong, Y. Li, J. Z. Wu, J. Feng, M. Lanza and H. Dai, Science, 2013, 342, 836–840 CrossRef CAS PubMed.
- T. Han, Y. Shi, X. Song, A. Mio, L. Valenti, F. Hui, S. Privitera, S. Lombardo and M. Lanza, J. Mater. Chem. A, 2016, 4, 8053–8060 CAS.
- F. A. L. Laskowski, M. R. Nellist, R. Venkatkarthick and S. W. Boettcher, Energy
Environ. Sci., 2017, 10, 570–579 CAS.
- K. Sun, M. T. McDowell, A. C. Nielander, S. Hu, M. R. Shaner, F. Yang, B. S. Brunschwig and N. S. Lewis, J. Phys. Chem. Lett., 2015, 6, 592–598 CrossRef CAS PubMed.
- J. Yang, J. K. Cooper, F. M. Toma, K. A. Walczak, M. Favaro, J. W. Beeman, L. H. Hess, C. Wang, C. Zhu, S. Gul, J. Yano, C. Kisielowski, A. Schwartzberg and I. D. Sharp, Nat. Mater., 2017, 16, 335–341 CrossRef CAS PubMed.
- X. Yu, P. Yang, S. Chen, M. Zhang and G. Shi, Adv. Energy Mater., 2016, 7, 1601805 CrossRef.
- S. Hu, N. S. Lewis, J. W. Ager, J. Yang, J. R. McKone and N. C. Strandwitz, J. Phys. Chem. C, 2015, 119, 24201–24228 CAS.
- D. Deng, K. S. Novoselov, Q. Fu, N. Zheng, Z. Tian and X. Bao, Nat. Nanotechnol., 2016, 11, 218–230 CrossRef CAS PubMed.
- X. Li, H. Zhu, K. Wang, A. Cao, J. Wei, C. Li, Y. Jia, Z. Li, X. Li and D. Wu, Adv. Mater., 2010, 22, 2743–2748 CrossRef CAS PubMed.
- X. Li, P. Sun, L. Fan, M. Zhu, K. Wang, M. Zhong, J. Wei, D. Wu, Y. Cheng and H. Zhu, Sci. Rep., 2012, 2, 395 CrossRef PubMed.
- X. Li, X. B. Zang, X. M. Li, M. Zhu, Q. Chen, K. L. Wang, M. L. Zhong, J. Q. Wei, D. H. Wu and H. W. Zhu, Adv. Energy Mater., 2014, 4, 1400224 CrossRef.
- Z. Kang, X. Tan, X. Li, T. Xiao, L. Zhang, J. Lao, X. Li, S. Cheng, D. Xie and H. Zhu, Phys. Chem. Chem. Phys., 2016, 18, 1992–1997 RSC.
- A. C. Nielander, M. J. Bierman, N. Petrone, N. C. Strandwitz, S. Ardo, F. Yang, J. Hone and N. S. Lewis, J. Am. Chem. Soc., 2013, 135, 17246–17249 CrossRef CAS PubMed.
- A. C. Nielander, A. C. Thompson, C. W. Roske, J. A. Maslyn, Y. F. Hao, N. T. Plymale, J. Hone and N. S. Lewis, Nano Lett., 2016, 16, 4082–4086 CrossRef CAS PubMed.
- C. C. Chen, M. Aykol, C. C. Chang, A. F. J. Levi and S. B. Cronin, Nano Lett., 2011, 11, 1863–1867 CrossRef CAS PubMed.
- Y. Song, X. M. Li, C. Mackin, X. Zhang, W. J. Fang, T. Palacios, H. W. Zhu and J. Kong, Nano Lett., 2015, 15, 2104–2110 CrossRef CAS PubMed.
- M. M. Furchi, A. A. Zechmeister, F. Hoeller, S. Wachter, A. Pospischil and T. Mueller, IEEE J. Sel. Top. Quantum Electron., 2017, 23, 4100111 CrossRef.
- R. D. L. Smith, M. S. Prévot, R. D. Fagan, Z. Zhang, P. A. Sedach, M. K. J. Siu, S. Trudel and C. P. Berlinguette, Science, 2013, 340, 60–63 CrossRef CAS PubMed.
- R. D. L. Smith, M. S. Prévot, R. D. Fagan, S. Trudel and C. P. Berlinguette, J. Am. Chem. Soc., 2013, 135, 11580–11586 CrossRef CAS PubMed.
- N. S. McIntyre and D. G. Zetaruk, Anal. Chem., 1977, 49, 1521–1529 CrossRef CAS.
- L. Freire, X. R. Nóvoa, M. F. Montemor and M. J. Carmezim, Mater. Chem. Phys., 2009, 114, 962–972 CrossRef CAS.
- N. S. McIntyre and M. G. Cook, Anal. Chem., 1975, 47, 2208–2213 CrossRef CAS.
- D. B. Mitton, J. Walton and G. E. Thompson, Surf. Interface Anal., 1993, 20, 36–42 CrossRef CAS.
- M. C. Biesinger, B. P. Payne, A. P. Grosvenor, L. W. M. Lau, A. R. Gerson and R. S. C. Smart, Appl. Surf. Sci., 2011, 257, 2717–2730 CrossRef CAS.
- S. C. Riha, B. M. Klahr, E. C. Tyo, S. Seifert, S. Vajda, M. J. Pellin, T. W. Hamann and A. B. F. Martinson, ACS Nano, 2013, 7, 2396–2405 CrossRef CAS PubMed.
- L. Trotochaud, J. K. Ranney, K. N. Williams and S. W. Boettcher, J. Am. Chem. Soc., 2012, 134, 17253–17261 CrossRef CAS PubMed.
- T. Y. Ma, S. Dai, M. Jaroniec and S. Z. Qiao, J. Am. Chem. Soc., 2014, 136, 13925–13931 CrossRef CAS PubMed.
- A. Kargar, S. Yavuz, T. K. Kim, C. H. Liu, C. Kuru, C. S. Rustomji, S. Jin and P. R. Bandaru, ACS Appl. Mater. Interfaces, 2015, 7, 17851–17856 CAS.
- R. Chen, H.-Y. Wang, J. Miao, H. Yang and B. Liu, Nano Energy, 2015, 11, 333–340 CrossRef CAS.
- C. C. McCrory, S. Jung, J. C. Peters and T. F. Jaramillo, J. Am. Chem. Soc., 2013, 135, 16977–16987 CrossRef CAS PubMed.
- J.-W. Jang, C. Du, Y. Ye, Y. Lin, X. Yao, J. Thorne, E. Liu, G. McMahon, J. Zhu, A. Javey, J. Guo and D. Wang, Nat. Commun., 2015, 6, 7447 CrossRef PubMed.
- Y. Jia, L. Zhang, A. Du, G. Gao, J. Chen, X. Yan, C. L. Brown and X. Yao, Adv. Mater., 2016, 28, 9532–9538 CrossRef CAS PubMed.
- B. Seger, T. Pedersen, A. B. Laursen, P. C. K. Vesborg, O. Hansen and I. Chorkendorff, J. Am. Chem. Soc., 2013, 135, 1057–1064 CrossRef CAS PubMed.
- C. L. Li, T. Hisatomi, O. Watanabe, M. Nakabayashi, N. Shibata, K. Domen and J.-J. Delaunay, Energy Environ. Sci., 2015, 8, 1493–1500 CAS.
- S. Fan, I. Shih and Z. Mi, Adv. Energy Mater., 2017, 7, 1600952 CrossRef.
- K. Yoon, J.-H. Lee, J. Kang, J. Kang, M. J. Moody, M. C. Hersam and L. J. Lauhon, Nano Lett., 2016, 16, 7370–7375 CrossRef CAS PubMed.
Footnote |
† Electronic supplementary information (ESI) available. See DOI: 10.1039/c7se00504k |
|
This journal is © The Royal Society of Chemistry 2018 |