DOI:
10.1039/C7SC04032F
(Edge Article)
Chem. Sci., 2018,
9, 356-361
Selective electrochemical generation of benzylic radicals enabled by ferrocene-based electron-transfer mediators†
Received
14th September 2017
, Accepted 25th October 2017
First published on 6th November 2017
Abstract
The generation and intermolecular functionalisation of carbon-centred radicals has broad potential synthetic utility. Herein, we show that benzylic radicals may be generated electrochemically from benzylboronate derivatives at low electrode potentials (ca. −0.3 V vs. Cp2Fe0/+) via single electron oxidation. Use of a catalytic quantity of a ferrocene-based electron-transfer mediator is crucial to achieve successful radical functionalisation and avoid undesirable side reactions arising from direct electrochemical oxidation or from the use of stoichiometric ferrocenium-based oxidants.
Carbon-centred radicals are versatile reaction intermediates,1,2 and recent studies have led to numerous methods to exploit these species in unique synthetic transformations.3 Radical pathways can be lower in energy and provide different selectivity relative to those based on other reactive carbonaceous species, such as carbanions or carbocations. The growing interest in accessing radical-based pathways for organic synthesis motivates efforts toward the development of new methods to generate these species.
Electrochemistry provides a unique opportunity to generate and manipulate radicals due to its reagent-free and tunable control over redox processes, and it continues to expand as a powerful technology for organic synthesis.4 The oxidative generation and functionalisation of radicals is often intimately linked to the nature of the chemical oxidant employed.5 Electrochemical oxidation of radical precursors, however, is not linked to the subsequent radical functionalisation step, thus potentially providing the basis for a wider variety of intermolecular functionalisation strategies. There are myriad examples of electrochemical oxidation to access net two-electron reactivity,6 but far fewer electrochemical methods exist that rely on single-electron pathways to selectively generate and functionalise neutral radicals (Fig. 1A). Most precedents feature trapping of an electrochemically generated radical by O2,7 while those undergoing anaerobic functionalisation are scarce.8 The limited number of precedents may be attributed, in part, to the proclivity of carbon-centred radicals to undergo side reactions when generated in close proximity to an electrode surface. Common side reactions include direct reaction with the electrode, further oxidation of the radical to afford carbocation species, and homocoupling of the radicals to afford dimeric (Kolbe-type) products (Fig. 1B). Intramolecular functionalisation of radicals can circumvent some of these problems, and a number of demonstrations of such reactivity have been recently described.9 Ultimately, however, it would be desirable to control the intermolecular reactivity of electrochemically generated radicals. Herein, we show that significantly improved control of radical reactivity is possible through the use of ferrocene-based electron-transfer mediators. The mediator shuttles redox equivalents into the bulk solution, away from the electrode surface where the radical is susceptible to degradation pathways, and it enables productive intermolecular reactivity through an electrochemical-chemical (EC′) mechanism (Fig. 1C). The results herein illustrate the utility of a mediated electrolysis strategy for radical generation,10,11 with potentially broader implications for the growing field of electro-organic chemistry.
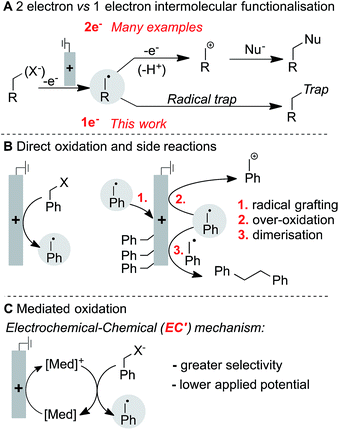 |
| Fig. 1 Aspects of electrochemical radical generation. | |
Benzylboronic esters were selected as appealing entry-points for electrochemical generation of carbon-centred radicals. The oxidative liberation of radicals from organoboron compounds has been demonstrated under photochemical12 conditions and with stoichiometric oxidants,13 but electrochemical oxidation of organoboron reagents to generate radical intermediates has yet to be fully explored.14
Benzylpinacol boronic ester (1a) was analysed by cyclic voltammetry, and was found not to undergo oxidation within the examined potential window (Fig. 2A). This observation is consistent with the need to use strong chemical oxidants (ca. 2 V vs. NHE)15 to oxidise neutral boronic acids.13a,b Addition of NaOH to 1a generates the anionic boronate (11B NMR), which is readily oxidised at lower potentials. The cyclic voltammogram (CV) exhibits an irreversible redox wave,16 suggesting single electron-transfer (SET) at the anode forms an unstable neutral radical that rapidly homolyses via C–B bond cleavage to give the benzylic radical (Fig. 2B). Variation of the boron substituents led to significant changes in the boronate oxidation potential, with an observed potential range of nearly 1 V (Fig. 2C). The potential is affected by both the ancillary ligation (e.g., diolate, diamide, trifluoro) and the identity of the anionic activator (X−), which appears to include both electronic (cf. TBAF vs. NaOH for 1a) and steric effects (cf. KOt-Bu vs. KOMe). The resulting benzylboronate species exhibit redox potentials that are more than 0.5 V lower than many functional groups commonly assumed to be easily oxidised, (e.g., enamines (0.0–0.2 V vs. Fc/Fc+), trialkylamines (0.45–0.55 V) or anilines (0.1–0.6 V)).17
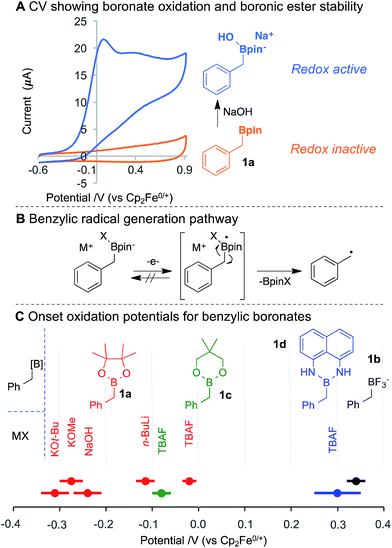 |
| Fig. 2 (A) CVs showing no oxidation of 1a without added base. (B) Single electron oxidation of benzylboronate with homolytic C–B bond cleavage to reveal radical. (C) Onset oxidation potentials measured for a variety of boronic esters (1a, 1c, 1d) with bases (colour-coded for their use with each boronic ester) and boronate 1b. Lines through points indicate a range of uncertainty. | |
Benzyltrifluoroborate 1b oxidises at the highest potential, consistent with the anionic stabilisation from three electronegative fluorides. The fluoride adduct of boronic ester 1c (derived from addition of TBAF) is oxidised more readily than the fluoride adduct of 1a, suggesting the 6-membered ring of 1c engenders a less stable tetrahedral boronate. In the absence of an anionic activator, aniline oxidation was observed in diaminoboron reagent 1d at approximately 0.1 V vs. Fc/Fc+. Addition of TBAF, however, increases the oxidation potential and leads to an irreversible CV trace,18 which indicates boronate oxidation and C–B bond cleavage. No electrochemical activity was observed for benzyl MIDA boronates and ill-defined redox activity was observed for a benzyl cyclic triol boronate.18 The significant influence of anion ligation to boronic ester derivatives offers a flexible strategy to adjust the oxidation potential for radical generation, and may find useful synthetic and materials applications beyond those presented herein.
During the voltammetric studies, cycling the applied potential multiple times led to a decrease in the magnitude of the response current (Fig. 3). This effect was observed for all tested boronates, with both glassy-carbon (GC) and Pt disk electrodes,18 and it was not attenuated by the presence of an exogenous radical trap, which could plausibly compete for reaction with the benzylic radical. The activity could only be restored after polishing the electrodes. SEM analysis of the electrode surface before and after fouling did not reveal bulk changes,18 suggesting that electrical insulation arises from molecular scale modification of the electrode surface.19 This conclusion is consistent with precedents for intentional derivatisation of electrode surfaces via oxidation of benzylcarboxylates19 or reduction of diazonium reagents.20
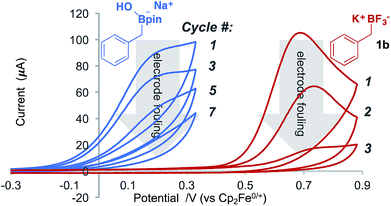 |
| Fig. 3 Cycled CVs reveal a current loss due to benzylic radical grafting and subsequent electrode fouling. CVs of 1a + NaOH in MeCN and TBAP (0.1 M) and oxo-TEMPO (5 mM) (blue) and 1b (5 mM) with dihydroanthracene (5 mM) in MeCN and TBAP (0.1 M) (red). | |
The electrode fouling observed by voltammetry was also manifested in the oxidation of boronates via bulk electrolysis (Scheme 1). The electrolysis was performed with RVC in the presence of 4 equivalents of TEMPO to trap the benzylic radical. The TEMPO-functionalised product was observed, but only in moderate yields and with a relatively poor mass balance (MB).21 This outcome, which could not be improved by altering the identity of the boronate, is attributed to non-productive substrate consumption and electrode fouling.
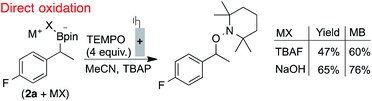 |
| Scheme 1 Inefficient radical generation and functionalisation with direct oxidation. Reactions performed in divided cells under N2 atmosphere with RVC:Pt electrodes (0.1 mmol scale), under constant potential (0.0 V, TBAF) or current (0.4 mA, NaOH), NMR yields shown. 2a used in bulk electrolyses for 19F NMR probe. Secondary benzylboronate oxidation potentials are between 60–90 mV lower than the primary benzylboronates shown in Fig. 2.18 | |
These observations prompted us to consider the use of an electrochemical mediator. Triarylamines22 and imidazoliums23 have been reported as electrochemical single-electron redox mediators, however, they operate at much higher oxidation potentials (ca. 0.5–1.5 V) that are poorly matched to the low potential benzylboronates (Fig. 2). On the other hand, ferrocene (Fc) derivatives display redox potentials in the appropriate range. Ferrocene itself was recently demonstrated by Xu and co-workers as an electrochemical mediator in radical generation for intramolecular functionalisation,24 but other ferrocene derivatives have yet to be explored in this role. The redox states of all tested ferrocene derivatives show stable and reversible activity (CV), and thus we decided to investigate their use as catalytic mediators for boronate oxidation.
Voltammetric analysis of two ferrocene derivatives, octamethyl–ferrocene (FcMe8) and dibromo-ferrocene (FcBr2), displayed an increased oxidation current in the presence of a boronate substrate (Fig. 4). This current increase is typical of an electrochemical-chemical (EC′) mechanism (Fig. 1C), in which the mediator is regenerated on the timescale of the CV scan, and is proportional to catalyst activity.25
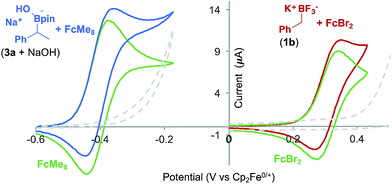 |
| Fig. 4 CVs showing ferrocene derivative mediated boronate oxidation. The increase in the current of the ferrocene derivative oxidation is due to catalytic boronate oxidation. Left: CVs (average of 3 runs) of FcMe8 (1.5 mM) in MeCN and TBAP (0.1 M), 10 mV s−1 (green) and, added to that, (1-phenethyl)pinacol boronic ester (50 mM) and NaOH (50 mM) (blue). Any current due to background substrate (3 + NaOH (50 mM)) oxidation (grey dashed) has been removed from the blue catalysis trace. Right: CVs (average of 3 runs) of FcBr2 (1 mM) in MeCN:THF (1 : 1) and TBAP (0.1 M) 10 mV s−1 (green) and, added to that, 1b (5 mM) (red). Current due to background substrate (1b (5 mM)) oxidation (grey dashed) has been removed from the red catalysis trace. | |
The onset redox potentials of these two ferrocene derivatives are approximately 200 mV lower than the onset potential of the respective boronates (3a + NaOH and 1b, respectively). This feature is designed to attenuate direct substrate oxidation at the electrode and ensure that the majority of the substrate is oxidised in the bulk solution by the mediator. The thermodynamically uphill electron transfer (200 mV = 4.6 kcal mol−1) is driven by rapid and irreversible C–B bond homolysis from the oxidised boronate derivative. Employing less oxidising ferrocene derivatives, in which the energy difference is larger, led to a decrease in the magnitude of the catalytic current, as evident by CV.18
The utility of ferrocenium mediators for boronate oxidation was then probed under bulk electrolysis conditions (Scheme 2).26 Use of a catalytic quantity of FcMe8 (10 mol%) led to a significantly improved yield of the benzylic TEMPO adduct. With constant current electrolysis, the oxidation proceeds at a lower potential (ca. 200 mV) than in the absence of the mediator, which attenuates electrode fouling processes that otherwise consume substrate (Fig. 1B). A lower concentration of TEMPO could also be tolerated under these conditions.
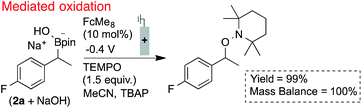 |
| Scheme 2 Efficient radical generation and productive functionalisation facilitated by the inclusion of a catalytic electron mediator. Reactions performed in divided cells under N2 atmosphere with RVC:Pt electrodes (0.1 mmol scale), NMR yields shown. | |
Electrochemically regenerating a catalytic ferrocenium derivative proved to be more effective than employing a stoichiometric quantity of the oxidant. The pairing of FcMe8+ or FcBr2+ with low and high potential boronates (2a + NaOH and 2b), respectively, only afforded low yields of the desired coupled products (Scheme 3). The increased concentration of the Fc+-based oxidants led to over-oxidation byproducts and boronate decomposition.18,27,28 These observations show that controlled electrochemical regeneration of a catalytic mediator can have advantages over the use of a stoichiometric chemical oxidant. The effectiveness of the mediated electrochemical oxidation strategy proved successful with other low-potential benzylboronates, exhibiting high yields and mass balances in each case (Scheme 4). This product class is useful29 as, for example, cation precursors30 or as initiators for controlled nitroxide-mediated polymerisation reactions.31
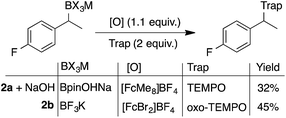 |
| Scheme 3 Inefficient radical generation and functionalisation with the use of stoichiometric quantities of oxidant. The more oxidatively resilient oxo-TEMPO was required when used in combination with the more oxidising FcBr2+. | |
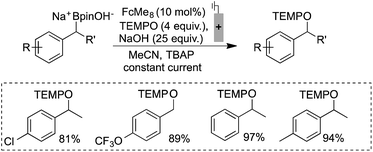 |
| Scheme 4 Efficient electrochemical mediated single electron oxidation and trapping of benzylic radical demonstrated. Reactions performed in divided cells under N2 atmosphere with RVC:Pt electrodes (0.1 mmol scale, 0.4 mA), NMR yields shown. | |
In summary, this study demonstrates the benefits of catalytic redox mediators in the electrochemical oxidative conversion of benzyl boronates to benzylic radicals. Mediated electrolysis avoids electrode fouling and side-product formation, which occur during direct electrochemical oxidation. Mediated electrolysis also offers several advantages over the use of stoichiometric ferrocenium-based oxidants, which lead to over-oxidation and substrate decomposition. These insights should aid the development of electrochemical methods for the generation and intermolecular functionalisation of carbon-centred radicals, a potentially transformative strategy in synthetic chemistry.
Conflicts of interest
There are no conflicts to declare.
Acknowledgements
The authors would like to acknowledge Zhenyang Jia and Garrett Wheeler for assistance with benzylboronic ester syntheses and SEM imaging, respectively. Financial support was provided by the DOE (DE-FG02-05ER15690, S. S. S.), and an NSF predoctoral Fellowship (DGE-1747503, JEN). Spectroscopic instrumentation was partially supported by the NIH (1S10 OD020022-1) and the NSF (CHE-1048642).
Notes and references
-
(a) C. P. Jasperse, D. P. Curran and T. L. Fevig, Chem. Rev., 1991, 91, 1237–1286 CrossRef CAS;
(b) M. Yan, J. C. Lo, J. T. Edwards and P. S. Baran, J. Am. Chem. Soc., 2016, 138, 12692–12714 CrossRef CAS PubMed.
-
(a) C. R. J. Stephenson, A. Studer and D. P. Curran, Beilstein J. Org. Chem., 2013, 9, 2778–2780 CrossRef PubMed;
(b) A. Studer and D. P. Curran, Angew. Chem., Int. Ed., 2016, 55, 58–102 CrossRef CAS PubMed.
-
(a) C. K. Prier, D. A. Rankic and D. W. C. MacMillan, Chem. Rev., 2013, 113, 5322–5363 CrossRef CAS PubMed;
(b) J. Hu, J. Wang, T. H. Nguyen and N. Zheng, Beilstein J. Org. Chem., 2013, 9, 1977–2001 CrossRef PubMed;
(c) N. A. Romero and D. A. Nicewicz, Chem. Rev., 2016, 116, 10075–10166 CrossRef CAS PubMed;
(d) A. Gansäuer and H. Bluhm, Chem. Rev., 2000, 100, 2771–2788 CrossRef;
(e) M. P. Sibi, S. Manyem and J. Zimmerman, Chem. Rev., 2003, 103, 3263–3296 CrossRef CAS PubMed;
(f) R. A. Rossi, A. B. Pierini and A. B. Peñéñory, Chem. Rev., 2003, 103, 71–167 CrossRef CAS PubMed;
(g) H. Yi, G. Zhang, H. Wang, Z. Huang, J. Wang, A. K. Singh and A. Lei, Chem. Rev., 2017, 117, 9016–9085 CrossRef CAS PubMed;
(h) D. Ravelli, S. Protti and M. Fagnoni, Chem. Rev., 2016, 116, 9850–9913 CrossRef CAS PubMed.
-
(a) E. J. Horn, B. R. Rosen and P. S. Baran, ACS Cent. Sci., 2016, 2, 302–308 CrossRef CAS PubMed;
(b) J. I. Yoshida, K. Kataoka, R. Horcajada and A. Nagaki, Chem. Rev., 2008, 108, 2265–2299 CrossRef CAS PubMed;
(c) D. S. P. Cardoso, B. Šljukić, D. M. F. Santos and C. A. C. Sequeira, Org. Process Res. Dev., 2017, 21, 1213–1226 CrossRef CAS.
- Selected examples:
(a) S. Bloom, C. R. Pitts, D. C. Miller, N. Haselton, M. G. Holl, E. Urheim and T. Lectka, Angew. Chem., Int. Ed., 2012, 51, 10580–10583 CrossRef CAS PubMed;
(b) Y. Amaoka, M. Nagatomo and M. Inoue, Org. Lett., 2013, 15, 2160–2163 CrossRef CAS PubMed;
(c) X. Huang, W. Liu, H. Ren, R. Neelamegam, J. M. Hooker and J. T. Groves, J. Am. Chem. Soc., 2014, 136, 6842–6845 CrossRef CAS PubMed;
(d) X. Huang and J. T. Groves, ACS Catal., 2016, 6, 751–759 CrossRef CAS;
(e) X. Huang, T. M. Bergsten and J. T. Groves, J. Am. Chem. Soc., 2015, 137, 5300–5303 CrossRef CAS PubMed;
(f) Y. Amaoka, S. Kamijo, T. Hoshikawa and M. Inoue, J. Org. Chem., 2012, 77, 9959–9969 CrossRef CAS PubMed.
- Selected examples:
(a) R. Hayashi, A. Shimizu and J. I. Yoshida, J. Am. Chem. Soc., 2016, 138, 8400–8403 CrossRef CAS PubMed;
(b) S. Kim, K. Hayashi, Y. Kitano, M. Tada and K. Chiba, Org. Lett., 2002, 4, 3735–3737 CrossRef CAS PubMed;
(c) K. J. Frankowski, R. Liu, G. L. Milligan, K. D. Moeller and J. Aubé, Angew. Chem., Int.
Ed., 2015, 54, 10555–10558 CAS;
(d) C. Zeng, N. Zhang, C. M. Lam and R. D. Little, Org. Lett., 2012, 14, 1314–1317 CrossRef CAS PubMed;
(e) E. Salahifar, D. Nematollahi, M. Bayat, A. Mahyari and H. Amiri Rudbari, Org. Lett., 2015, 17, 4666–4669 CrossRef CAS PubMed;
(f) B. Yin, S. Inagi and T. Fuchigami, Beilstein J. Org. Chem., 2015, 11, 85–91 CrossRef PubMed;
(g) J. I. Yoshida, S. Suga, S. Suzuki, N. Kinomura, A. Yamamoto and K. Fujiwara, J. Am. Chem. Soc., 1999, 121, 9546–9549 CrossRef CAS;
(h) S. Kim, T. Shoji, Y. Kitano and K. Chiba, Chem. Commun., 2013, 49, 6525–6527 RSC.
-
(a) M. Masui, S. Hara, T. Ueshima, T. Kawaguchi and S. Ozaki, Chem. Pharm. Bull., 1983, 31, 4209–4211 CrossRef CAS;
(b) E. J. Horn, B. R. Rosen, Y. Chen, J. Tang, K. Chen, M. D. Eastgate and P. S. Baran, Nature, 2016, 533, 77–81 CrossRef CAS PubMed;
(c) Y. Kawamata, M. Yan, Z. Liu, D.-H. Bao, J. Chen, J. T. Starr and P. S. Baran, J. Am. Chem. Soc., 2017, 139, 7448–7451 CrossRef CAS PubMed;
(d) D. P. Hruszkewycz, K. C. Miles, O. R. Thiel and S. S. Stahl, Chem. Sci., 2017, 8, 1282–1287 RSC.
- For example:
(a) A. G. O'Brien, A. Maruyama, Y. Inokuma, M. Fujita, P. S. Baran and D. G. Blackmond, Angew. Chem., Int. Ed., 2014, 53, 11868–11871 CrossRef PubMed;
(b) N. Fu, G. S. Sauer, A. Saha, A. Loo and S. Lin, Science, 2017, 357, 575–579 CrossRef CAS PubMed.
-
(a) P. Xiong, H.-H. Xu and H.-C. Xu, J. Am. Chem. Soc., 2017, 139, 2956–2959 CrossRef CAS PubMed;
(b) H.-B. Zhao, Z.-W. Hou, Z.-J. Liu, Z.-F. Zhou, J. Song and H.-C. Xu, Angew. Chem., Int. Ed., 2017, 56, 587–590 CrossRef CAS PubMed;
(c) A. Redden, R. J. Perkins and K. D. Moeller, Angew. Chem., Int. Ed., 2013, 52, 12865–12868 CrossRef CAS PubMed;
(d) J. A. Smith and K. D. Moeller, Org. Lett., 2013, 15, 5818–5821 CrossRef CAS PubMed;
(e) H.-C. Xu, J. M. Campbell and K. D. Moeller, J. Org. Chem., 2014, 79, 379–391 CrossRef CAS PubMed.
-
(a) R. Francke and R. D. Little, Chem. Soc. Rev., 2014, 43, 2492–2521 RSC;
(b) E. Steckhan, Angew. Chem., Int. Ed., 1986, 25, 683–701 CrossRef;
(c) Y. N. Ogibin, M. N. Elinson and G. I. Nikishin, Russ. Chem. Rev., 2009, 78, 89–140 CrossRef CAS;
(d) J. Savéant, Chem. Rev., 2008, 108, 2348–2378 CrossRef PubMed.
- Mediators are prevalent in electrochemical energy conversion applications, see, for example:
(a) D. L. Dubois, Inorg. Chem., 2014, 53, 3935–3960 CrossRef CAS PubMed;
(b) J. J. Concepcion, J. W. Jurss, M. K. Brennaman, P. G. Hoertz, A. O. T. Patrocinio, N. Y. Murakami Iha, J. L. Templeton and T. J. Meyer, Acc. Chem. Res., 2009, 42, 1954–1965 CrossRef CAS PubMed.
-
(a) Y. Yasu, T. Koike and M. Akita, Adv. Synth. Catal., 2012, 354, 3414–3420 CrossRef CAS;
(b) J. C. Tellis, D. N. Primer and G. a. Molander, Science, 2014, 345, 433–436 CrossRef CAS PubMed;
(c) F. Lima, M. A. Kabeshov, D. N. Tran, C. Battilocchio, J. Sedelmeier, G. Sedelmeier, B. Schenkel and S. V. Ley, Angew. Chem., Int. Ed., 2016, 55, 14085–14089 CrossRef CAS PubMed;
(d) G.-X. Li, C. A. Morales-Rivera, Y. Wang, F. Gao, G. He, P. Liu and G. Chen, Chem. Sci., 2016, 7, 6407–6412 RSC;
(e) J. K. Matsui, D. N. Primer and G. A. Molander, Chem. Sci., 2017, 8, 3512–3522 RSC.
-
(a) I. B. Seiple, S. Su, R. A. Rodriguez, R. Gianatassio, Y. Fujiwara, A. L. Sobel and P. S. Baran, J. Am. Chem. Soc., 2010, 132, 13194–13196 CrossRef CAS PubMed;
(b) A. Dickschat and A. Studer, Org. Lett., 2010, 12, 3972–3974 CrossRef CAS PubMed;
(c) K. Miyazawa, Y. Yasu, T. Koike and M. Akita, Chem. Commun., 2013, 49, 7249–7251 RSC;
(d) G. Sorin, R. Martinez mallorquin, Y. Contie, A. Baralle, M. Malacria, J. P. Goddard and L. Fensterbank, Angew. Chem., Int. Ed., 2010, 49, 8721–8723 CrossRef CAS PubMed.
- The two-electron oxidation of boronic acids and trifluoroborates for reaction with nucleophiles under electrochemical conditions has been recently reported. See:
(a) J. Suzuki, N. Shida, S. Inagi and T. Fuchigami, Electroanalysis, 2016, 28, 2797–2801 CrossRef CAS;
(b) J. Suzuki, M. Tanigawa, S. Inagi and T. Fuchigami, ChemElectroChem, 2016, 3, 2078–2083 CrossRef CAS.
- R. Memming, J. Electrochem. Soc., 1969, 116, 785–790 CrossRef CAS.
- Also observed in14a, b and L. A. Shundrin, V. V. Bardin and H. J. Frohn, Z. Anorg. Allg. Chem., 2004, 630, 1253–1257 CrossRef CAS.
- H. G. Roth, N. A. Romero and D. A. Nicewicz, Synlett, 2016, 27, 714–723 CAS.
- See ESI† for details.
- The mechanism suggested by Saveant involves a further oxidation of the benzylic radical to a carbocation that reacts with a surface bound carbon. STM images in this study provide evidence for a molecular monolayer. C. P. Andrieux, F. Gonzalez and J. M. Savéant, J. Am. Chem. Soc., 1997, 119, 4292–4300 CrossRef CAS.
- For example:
(a) M. Raicopol, L. Necula, M. Ionita and L. Pilan, Surf. Interface Anal., 2012, 44, 1081–1085 CrossRef CAS;
(b) T. Menanteau, M. Dias, E. Levillain, A. J. Downard and T. Breton, J. Phys. Chem. C, 2016, 120, 4423–4429 CrossRef CAS.
- Electrolyses performed with a Pt mesh electrode also gave very low yields of products. See ESI† for details.
-
(a) T. Fuchigami, M. Tetsu, T. Tajima and H. Ishii, Synlett, 2001, 1269–1271 CrossRef CAS;
(b) Y. Shen, K. Suzuki, M. Atobe and T. Fuchigami, J. Electroanal. Chem., 2003, 540, 189–194 CrossRef CAS;
(c) Y. Shen, H. Hattori, K. Ding, M. Atobe and T. Fuchigami, Electrochim. Acta, 2006, 51, 2819–2824 CrossRef CAS;
(d) Y. S. Park and R. D. Little, Electrochim. Acta, 2009, 54, 5077–5082 CrossRef CAS;
(e) Y. S. Park and R. D. Little, J. Org. Chem., 2008, 73, 6807–6815 CrossRef CAS PubMed;
(f) Y. S. Park, S. C. Wang, D. J. Tantillo and R. D. Little, J. Org. Chem., 2007, 72, 4351–4357 CrossRef CAS PubMed;
(g) X. Wu, A. P. Davis and A. J. Fry, Org. Lett., 2007, 9, 5633–5636 CrossRef CAS PubMed;
(h) X. Wu, A. P. Davis, P. C. Lambert, L. K. Steffen, O. Toy and A. J. Fry, Tetrahedron, 2009, 65, 2408–2414 CrossRef CAS.
-
(a) N. Lu, N. Zhang, C.-C. Zeng, L.-M. Hu, S. J. Yoo and R. D. Little, J. Org. Chem., 2015, 80, 781–789 CrossRef CAS PubMed;
(b) C. Zeng, N. Zhang, C. M. Lam and R. D. Little, Org. Lett., 2012, 14, 1314–1317 CrossRef CAS PubMed;
(c) R. Francke and R. D. Little, J. Am. Chem. Soc., 2014, 136, 427–435 CrossRef CAS PubMed.
-
(a) L. Zhu, P. Xiong, Z. Y. Mao, Y. H. Wang, X. Yan, X. Lu and H. C. Xu, Angew. Chem., Int. Ed., 2016, 55, 2226–2229 CrossRef CAS PubMed;
(b) Z. Wu and H. Xu, Angew. Chem., Int. Ed., 2017, 56, 4734–4738 CrossRef CAS PubMed;
(c) Z.-W. Hou, Z.-Y. Mao, J. Song and H.-C. Xu, ACS Catal., 2017, 7, 5810–5813 CrossRef CAS . Ferrocene has also been reported (ref. 26(c)–(e)) to mediate the oxidation of acetate ions to their respective decarboxylated alkyl radicals that participate in Kolbe-type couplings, however, no reaction products were isolated or observed.
- C. Costentin and J.-M. Savéant, ChemElectroChem, 2014, 1, 1226–1236 CrossRef CAS.
- CV studies revealed an irreversible, deactivating interaction between fluoride and ferrocenium, which rendered the use of TBAF as unsuitable for boronate formation for mediated oxidation. KOt-Bu and NaOH did not reveal (CV) deactivating electrostatic interactions with ferrocenium, presumably for steric and solubility reasons, respectively. However, several other bases have also been reported to display such effects, see, for example:
(a) Q. Shu, L. Birlenbach and M. Schmittel, Inorg. Chem., 2012, 51, 13123–13127 CrossRef CAS PubMed . It is proposed that an electrostatically induced tight ion pair is formed that adsorbs onto the electrode surface, see:;
(b) M. Buda, A. Ion, J. Moutet, E. Saint-Aman and R. Ziessel, J. Electroanal. Chem., 1999, 469, 132–138 CrossRef CAS . Acetate ions and ferrocenium display similar effects by CV, see;
(c) L. S. Hernández-Munoz, A. Galano, P. D. Astudillo-Sánchez, M. M. Abu-Omar and F. J. González, Electrochim. Acta, 2014, 136, 542–549 CrossRef;
(d) L. S. Hernandez-Munoz, R. J. Fragoso-Soriano, C. Vazquez-Lopez, E. Klimova, L. A. Ortiz-Frade, P. D. Astudillo and F. J. Gonzalez, J. Electroanal. Chem., 2010, 650, 62–67 CrossRef CAS;
(e) P. D. Astudillo, A. Galano and F. J. González, J. Electroanal. Chem., 2007, 610, 137–146 CrossRef CAS , wherein electrode fouling is also observed, which should be due to adsorption of the tight ion pair that is formed.
- Use of stoichiometric FcMe8+, for the oxidation of boronate 2a + NaOH, mostly returned boronic ester 2a, which has a high oxidation potential.18 Use of stoichiometric FcBr2+ for oxidation of 2b gave a fluorination product, in which an increase in the oxidant concentration presumably promotes two-electron oxidation of the benzylboronate to generate a benzylic carbocation that may be trapped by fluoride from the BF4− counter-anion.
- Kolbe-type dimerisation products were not observed under any conditions in this system.
-
(a) T. Vogler and A. Studer, Synthesis, 2008, 2008, 1979–1993 CrossRef;
(b) L. Tebben and A. Studer, Angew. Chem., Int. Ed., 2011, 50, 5034–5068 CrossRef CAS PubMed and references therein.
- For example: Q. Zhu, E. C. Gentry and R. R. Knowles, Angew. Chem., Int. Ed., 2016, 55, 9969–9973 CrossRef CAS PubMed.
-
(a) A. Studer, Chem. Soc. Rev., 2004, 33, 267–273 RSC;
(b) D. Bertin, D. Gigmes, S. R. A. Marque and P. Tordo, Macromolecules, 2005, 38, 2638–2650 CrossRef CAS.
Footnotes |
† Electronic supplementary information (ESI) available: Experimental details, procedures and spectroscopic characterisations. See DOI: 10.1039/c7sc04032f |
‡ Present address: School of Chemistry, University of Bristol, Cantock's Close, Bristol, BS8 1TS, UK, Email: a.lennox@bristol.ac.uk |
|
This journal is © The Royal Society of Chemistry 2018 |