DOI:
10.1039/C8RA07559J
(Paper)
RSC Adv., 2018,
8, 39529-39535
Characterization of immobilized tyrosinase – an enzyme that is stable in organic solvent at 100 °C†
Received
11th September 2018
, Accepted 2nd November 2018
First published on 27th November 2018
Abstract
Tyrosinase is a copper-containing enzyme present in plant and animal tissues, which catalyzes the production of melanin and other pigments. In organic solvent, tyrosinase can convert N-acetyl-L-tyrosine ethyl ester (insoluble in aqueous) to a derivative of L-dopamine (a drug used for the treatment of Parkinson's disease). Thus, the performances of tyrosinase in organic solvent have attracted scientific attention since 1980. In this work, we investigated the stability of immobilized tyrosinase at high temperature in anhydrous organic solvent. Triethylaminoethyl cellulose (TEAE-Cellulose) performed the best out of six immobilization platforms. The dry immobilized tyrosinase became extremely thermostable in organic solvent, and the half-life of the dry immobilized tyrosinase in organic solvent is strongly related to the polarity of the organic solvent than their log
P value. The immobilized tyrosinase loses its activity instantaneously in aqueous solution at 100 °C, but it keeps enzymatic activity within 10 min in hydrophilic methanol and over one month in hydrophobic hexane (log
P: 4.66, non-polar) even incubating at 100 °C. This research provides valuable information for the design of new biocatalysts.
Introduction
Mimicking tyrosinase has been a longstanding goal of industrial and academic chemists.1 Tyrosinases (polyphenol oxidase, EC 1.14.18.1), copper-containing oxidoreductases, can enable aerobic oxidation of relatively inert C–H bonds, which remains difficult for synthetic catalysts. Most of the synthetic catalysts can be stable in most of the organic solvents at high temperature (mostly higher than 100 °C). The behavior of tyrosinase in organic solvents at high temperature is very interesting for mimicking tyrosinase. Tyrosinase is an enzyme with monophenolase and diphenolase activity.2–4 Since the 1980s, innovative research has shown that tyrosinase can function well in anhydrous media with minimal water, pioneered mainly by Klibanov et al.5,6 This research remained of broad interest in the last century and mushroom tyrosinase has been utilized as an ideal enzyme by many researchers to study the functioning of enzymes in organic media.7,8 The enzyme regioselectivity and enzymatic catalysis in organic media have been systematically investigated,9,10 but there is no report about the thermostable behavior of tyrosinase in different pure organic solvents at high temperature.
To study the thermostable behavior of tyrosinase,11,12 the enzyme needs to be incubated in different organic solvents and undergo testing of its activity in the same organic solvent. In this way, the enzyme must be easy to reuse.13,14 As previously reported, immobilization techniques are very efficient method to recycle the enzyme.15–17 The enzyme immobilized on a solid carrier can make the enzyme reusability easier.18,19 It is one of the key steps to test the thermostability of immobilized enzymes.20–22 From previous reports,23,24 the adsorption method keeps the original structure of enzyme,25–27 but encounters issues with enzyme leaching. Compared with the covalent bonding,28,29 entrapment,30–32 copolymerization33,34 and encapsulation methods,35–37 the conditions of the adsorption method are much milder than the other methods. Furthermore, the glass beads,38,39 graphene,40,41 magnetic nanoparticles42 and metal–organic frameworks (MOFs)43,44 were chosen as the supports to immobilize the enzyme in previous study. In this study, the biocompatible polymers (i.e. cellulose) were utilized as the ideal matrixes due to their low cost, and renewable and biodegradable nature.45–47 Besides, we systematically studied different polymers (i.e. cellulose matrixes, Pluronic F68 and poly(styrene-co-divinylbenzene)) and traditional glass beads as enzyme support.48
After optimizing the enzyme immobilization material, the organic solvent effect, the position of substituent group and the strength of electron-donating substituent group were studied. Furthermore, the activities of immobilized tyrosinase were tested in various hydrophilic and hydrophobic organic solvents at high temperature. This study provided more reasonable and systematical explanation of tyrosinase in organic solvents.
Experimental section
Materials
p-Cresol, p-chlorophenol, p-methoxyphenol and other chemicals obtained from Sigma Aldrich (USA) were of the highest purity available. Mushroom Tyrosinase (50 kU, powder) was purchased from Sigma Aldrich (USA) as a solid with a specific activity of 2430 unit per mg (1 unit is defined as the enzyme activity resulting in an increase in absorbance at 280 nm of 0.001 at pH 6.5 at 25 °C in a 3 mL reaction volume containing L-tyrosine). Poly(styrene-co-divinylbenzene), glass beads, Pluronic F68, cellulose, carboxymethyl cellulose (CM-Cellulose) and triethylaminoethyl cellulose (TEAE-Cellulose) were also purchased from Sigma Aldrich (USA).
Instrumentation
Scanning electron microscope (SEM) images were obtained through high-resolution SEM (Zeiss Merlin, Germany) with a resolution of 0.8 nm at 15 kV and 1.4 nm at 1 kV. Transmission electron microscope (TEM) images were obtained from FEI Tecnai Multipurpose TEM (ThermoFisher Scientific, USA). UV-Vis spectrum was obtained by a UV-Vis Nanodrop 2000C spectroscopy (ThermoFisher Scientific, USA).
Preparation of tyrosinase and immobilization onto TEAE-Cellulose
Tyrosinase (6 mg) was dissolved in 0.3 mL of 50 mmol L−1 phosphate buffer (pH 7.0), and then 60 mg of cellulose was added. The sticky mixture was spread on a watch glass and left to dry at room temperature. Tyrosinase immobilization onto CM-Cellulose or other materials was prepared following a similar protocol as tyrosinase immobilization onto TEAE-Cellulose.
UV-Vis spectrophotometry detection of the tyrosinase-catalyzed oxidation product
The time course for tyrosinase-catalyzed oxidation of p-cresol in organic solvents was measured by the following procedure: p-cresol was dissolved in a given solvent (its concentration: 50 mmol L−1). 1 mL of p-cresol (50 mmol L−1) in organic solvent was added into a 5 mL round-bottom flask, followed by addition of 5 μL 50 mmol L−1 phosphate buffer (PBS, pH 7.0). Then, the immobilized tyrosinase was added. Subsequently, the suspension was stirred by magnetic stirrers at 250 rpm at 25 °C. Aliquots of the liquid were removed and their absorption spectrum was recorded in the range 400–600 nm. The detection procedure of other substrates in a given solvent was the same as p-cresol in a given solvent.
Stability of tyrosinase in organic solvent at high temperature
The immobilized tyrosinase was transferred into a round bottom flask with anhydrous organic solvent under stirring at 100 °C. After 1 hour, the anhydrous organic solvent was removed, and then 1 mL of p-cresol in toluene with 5 μL 50 mmol L−1 PBS (pH 7.0) was added. The detection procedure was the same as the time course for tyrosinase-catalyzed oxidation of p-cresol in a given solvent.
Results and discussion
Physical characterization of TEAE-Cellulose and tyrosinase immobilization onto TEAE-Cellulose
Fig. 1B displays the SEM image of tyrosinase immobilization onto TEAE-Cellulose as compared to the original TEAE-Cellulose (Fig. 1A). There was a significant change in the morphology between the original TEAE-Cellulose and tyrosinase immobilization onto TEAE-Cellulose. The original TEAE-Cellulose had a relative clear edge, and the TEAE-Cellulose with tyrosinase showed an unclear edge, rough surface and more porosity than the original one. Fig. 1C and D show the TEM image of original TEAE-Cellulose and TEAE-Cellulose with tyrosinase, respectively. The original TEAE-Cellulose surface was very smooth. After tyrosinase immobilization onto TEAE-Cellulose, its surface was rougher than the original one. Pure TEAE-Cellulose has a clear edge from the TEM and SEM images. After tyrosinase is immobilized onto TEAE-Cellulose, TEAE-Cellulose and tyrosinase can cross-link with each other through electrostatic attraction and hydrogen-bond interactions. In this way, the edge of TEAE-Cellulose becomes unclear and rough, and there is some porosity on its surface. Fig. S1A-S1E† display the SEM images of (A) Cellulose, (B) CM-Cellulose, (C) Pluronic F68, (D) poly(styrene-co-divinylbenzene) and (E) glass beads. After immobilization of tyrosinase onto the surface of materials, cellulose, CM-Cellulose and Pluronic F68 morphologies totally changed, and their surfaces became rougher than before. These alterations showed that the tyrosinase had been immobilized onto these materials.
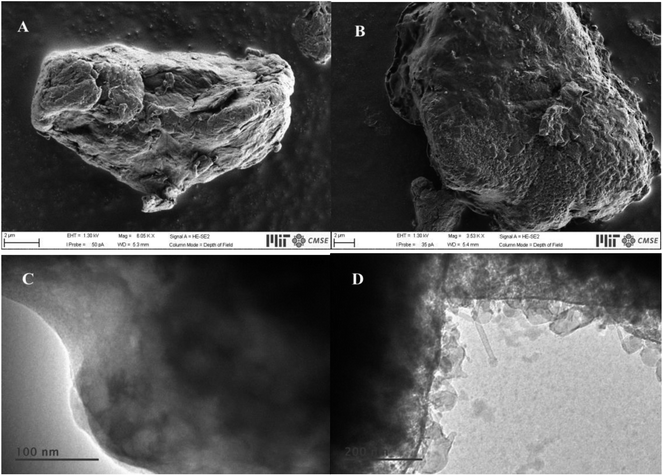 |
| Fig. 1 SEM of (A) triethylaminoethyl cellulose (TEAE-Cellulose) and (B) TEAE-Cellulose with tyrosinase; the TEM of (C) triethylaminoethyl cellulose (TEAE-Cellulose) and (D) TEAE-Cellulose with tyrosinase. | |
Immobilized tyrosinase biocatalytic ability
Six kinds of materials were used for the immobilization of tyrosinase: the triethylaminoethyl group of TEAE-Cellulose exhibits positive charge (pH 7.0, PBS solution); cellulose, Pluronic F68, poly(styrene-co-divinylbenzene) and glass beads have neutral charges; and the carboxymethyl group of CM-Cellulose has negative charge. As shown in Fig. 2, the TEAE-Cellulose could bind tyrosinase (negative charge at pH 7.0) due to charge attraction. TEAE-Cellulose is a positively charged resin used in protein purification or separation. It can lock negatively charged protein – tyrosinase onto the carrier by charge attraction and hydrogen-bond interactions. Cellulose can lock part of tyrosinase only through hydrogen-bond interactions. CM-Cellulose cannot lock tyrosinase like TEAE-Cellulose through charge attraction, but a few of tyrosinase can be locked by hydrogen-bond interactions. The tyrosinase molecules were physically adsorbed onto the surface of Pluronic F68, poly(styrene-co-divinylbenzene) and glass beads. The tyrosinase molecules immobilization efficiency of different materials could be arranged as: TEAE-Cellulose > cellulose = Pluronic F68 = poly(styrene-co-divinylbenzene) = glass beads > CM-Cellulose.
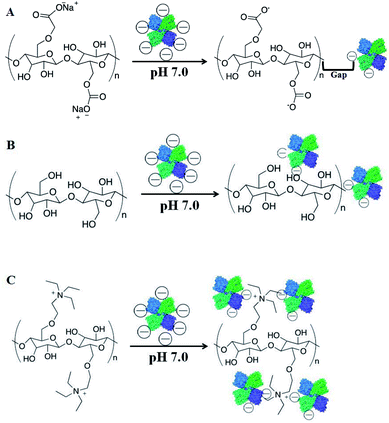 |
| Fig. 2 Schematic diagram describing the mechanism of tyrosinase immobilization onto (A) CM-Cellulose, (B) cellulose and (C) TEAE-Cellulose. | |
p-Cresol was chosen as the test substrate. The tyrosinase can function in chloroform whereby phenol derivatives can be converted into corresponding stable o-quinones. The enzymatic oxidation product of p-cresol is 4-methyl-1,2-benzoquinone, and its maximum absorbance peak is at 395 nm. Kinetic analysis of tyrosinase is shown in Fig. 3. The Vo values of immobilized tyrosinase onto TEAE-Cellulose, cellulose, poly(styrene-co-divinylbenzene), Pluronic F68, glass beads and CM-Cellulose were 6.7 × 10−2, 5.5 × 10−2, 4.4 × 10−2, 3.6 × 10−2, 2.8 × 10−2 and 1.5 × 10−2 mmol L−1 min−1, respectively. The Vo value of immobilized tyrosinase onto TEAE-Cellulose was the highest among those onto CM-Cellulose, cellulose, Pluronic F68, poly(styrene-co-divinylbenzene) and glass beads, and the Vo value of immobilized tyrosinase onto CM-Cellulose was the lowest among these materials. The results were consistent with the mechanism shown in Fig. 2. The TEAE-Cellulose was demonstrated to be the best supporting platform for tyrosinase and it was chosen as the immobilization material in the following research work.
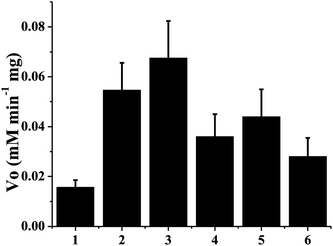 |
| Fig. 3 Vo values of tyrosinase molecular immobilization onto CM-Cellulose, cellulose, TEAE-Cellulose, Pluronic F68, poly(styrene-co-divinylbenzene) and glass beads catalyzed oxidations of p-cresol as substrate in chloroform. p-Cresol concentration for these reactions is 50 mmol L−1. Error bar means standard deviation. | |
Immobilization enzyme kinetics in organic solvent
After optimizing the tyrosinase immobilization platform, the stability of immobilized tyrosinase was systematically assessed in different organic solvents. Organic solvents can affect the structure of enzymes at the secondary, tertiary and quaternary levels.7 It is important to note how organic solvents affect the immobilized tyrosinase activity. From Table 1, the data demonstrates that the immobilized tyrosinase was completely inactive in hydrophilic solvents viz. acetonitrile (log
P, −0.34) and tetrahydrofuran (log
P, 0.46). This can be attributed to the fact that the hydrophilic solvents could strip the essential water from tyrosinase's hydration “shell” (hence deactivate the enzyme). Moreover, the immobilized tyrosinase had biocatalytic ability in hydrophobic solvent i.e. tert-butyl methyl ether (log
P, 0.94), methylene chloride (log
P, 1.25), chloroform (log
P, 1.97), carbon tetrachloride (log
P, 2.83), chlorobenzene (log
P, 2.84) and toluene (log
P, 2.73). Octanol–water partition coefficient (log
P) is used in QSAR studies and rational chemical design as a measurement of molecular hydrophobicity. Our results suggested that the hydrophobicity of organic solvent played an important role in tyrosinase efficiency. As the hydrophobicity of organic solvents increased, most of the biocatalytic efficiency of the immobilized tyrosinase increased. There is an exception to this rule. 1-Octanol is categorized as a hydrophobic solvent and the value of log
P is relatively high (achieved 3, similarly with toluene and carbon tetrachloride), but the Vo value of the immobilized tyrosinase in 1-octanol is relatively low (less than 8.3 × 10−4). In this case, the viscosity of 1-octanol achieves as high as 7.4 × 10−3 Pa s, and the mass-transfer rate of substrate in 1-octanol is very slow. This might be one of the most important parameters in determining the biocatalytic efficiency of the immobilized tyrosinase. As a result, toluene was chosen as the solvent to test the immobilized tyrosinase activity.
Table 1 The activity of tyrosinase immobilization onto TEAE-Cellulose was detected in organic solvent (i.e. CH2Cl2, CCl4 and toluene)
p-Cresol |
Vo (mmol L−1 min−1) |
Std |
log P |
Viscosity 10−3 Pa s |
Acetonitrile |
— |
— |
−0.34 |
0.34 |
Tetrahydrofuran |
— |
— |
0.46 |
0.46 |
1-Octanol |
<8.3 × 10−4 |
— |
3 |
7.4 |
tert-Butyl methyl ether |
8.3 × 10−4 |
2.6 × 10−4 |
0.94 |
0.36 |
CH2Cl2 |
1.5 × 10−3 |
2.5 × 10−4 |
1.25 |
0.42 |
Chlorobenzene |
5.7 × 10−2 |
5.0 × 10−3 |
2.84 |
0.75 |
CHCl3 |
6.1 × 10−2 |
4.6 × 10−3 |
1.97 |
0.54 |
CCl4 |
6.3 × 10−2 |
3.0 × 10−3 |
2.83 |
0.9 |
Toluene |
6.7 × 10−2 |
1.4 × 10−3 |
2.73 |
0.55 |
The biocatalytic efficiency of the immobilized tyrosinase for different substituent groups of para-cresol isomers in hydrophobic organic solvent
After optimizing the immobilized tyrosinase solvent conditions, the relationship between the structure of the substrate and the activity of the immobilized tyrosinase was systematically studied. How the substituent group position (three isomers of p-cresol) influenced the selectivity of the immobilized tyrosinase in toluene was examined. Table 2 shows that the Vo value for tyrosinase in the toluene medium, using p-cresol as substrate, was 8.7-fold higher than those obtained with m-cresol as substrate and more than 30-fold higher than those with o-cresol as substrate (the Vo number was too small to check). The ortho-substituent group was not significantly catalyzed by the immobilized tyrosinase because such substrates sterically hinder the accessibility of the phenolic hydroxyl moiety to the active-site of tyrosinase. The steric influence of the para substituent group of phenol for the biocatalysis of tyrosinase was less than that of meta or ortho substituents.
Table 2 The bioactivity of tyrosinase was tested for different substituent sites in different solvent (i.e. CH2Cl2, CCl4 and toluene)
Substrate |
p-Cresol (Vo) |
p-Cresol (Std) |
m-Cresol (Vo) |
m-Cresol (Std) |
o-Cresol (Vo) |
o-Cresol (Std) |
Toluene |
6.7 × 10−2 |
1.4 × 10−3 |
7.7 × 10−3 |
2.2 × 10−4 |
<8.3 × 10−4 |
— |
Chlorobenzene |
5.7 × 10−2 |
5.0 × 10−3 |
6.5 × 10−3 |
1.9 × 10−5 |
<8.3 × 10−4 |
— |
CHCl3 |
6.1 × 10−2 |
4.6 × 10−3 |
7.0 × 10−3 |
1.2 × 10−4 |
<8.3 × 10−4 |
— |
CCl4 |
6.3 × 10−2 |
3.0 × 10−3 |
7.2 × 10−3 |
1.2 × 10−4 |
<8.3 × 10−4 |
— |
It was essential to know how the different para substituent groups influence the catalytic efficiencies of the immobilized tyrosinase. Different para-substituent groups of phenol were tested in toluene. Table 3 shows that the Vo value of tyrosinase biocatalysis for para substituent were: p-methyl > p-ethyl > p-propyl > p-chloro > p-bromo > p-t-butyl. The methyl, ethyl, propyl and t-butyl groups are electron-donating substituent groups. By increasing the electron-donating ability and the steric size of para-substituents, the corresponding Vo value of the immobilized tyrosinase decreased. It indicated that the tyrosinase activity was related to the electron-donating ability and steric size of the substitute. In contrast, p-chloro and p-bromo are electron-withdrawing substituent groups; their corresponding Vo value of the immobilized tyrosinase decreased with their deactivating ability. The conclusion was consistent with the quantitative assessment of enzymatic catalysis by Dordick et al.49
Table 3 The bioactivity of tyrosinase was detected for six kinds of para-substituents of phenol in toluene
Substrate |
Vo (mmol L−1 min−1) |
Std |
log Kow |
Solubility in water (mol m−3) |
p-Methyl |
6.7 × 10−2 |
1.4 × 10−3 |
1.96 |
184.9 |
p-Ethyl |
4.1 × 10−2 |
3.2 × 10−3 |
2.50 |
65.3 |
p-Propyl |
2.8 × 10−2 |
2.1 × 10−3 |
3.20 |
12.7 |
p-Chloro |
2.1 × 10−2 |
1.8 × 10−3 |
2.40 |
210.0 |
p-Bromo |
9.2 × 10−3 |
8.5 × 10−4 |
2.59 |
85.54 |
p-t-Butyl |
2.0 × 10−3 |
2.0 × 10−5 |
3.65 |
3.861 |
After optimizing the immobilized platform, the substrate and the solvent, the behavior of tyrosinase in anhydrous organic solvent at high temperature was studied. In this way, the immobilized tyrosinase was treated as a synthetic catalyst. Nine kinds of anhydrous organic solvents were used for storing the immobilized tyrosinase at 100 °C. The nine anhydrous organic solvents are methanol (log
P, −0.77; relative polarity, 0.762), ethanol (log
P, −0.31; relative polarity, 0.654), acetonitrile (log
P, −0.34; relative polarity, 0.46), acetone (log
P, −0.24; relative polarity, 0.355), methyl acetate (log
P, 0.18; relative polarity, 0.762), tetrahydrofuran (log
P, 0.46; relative polarity, 0.207), methyl t-butyl ether (log
P, 0.94; relative polarity, 0.124), toluene (log
P, 2.73; relative polarity, 0.099) and hexane (log
P, 3.9; relative polarity, 0.009). As shown in Fig. 4, the half-life of the immobilized tyrosinase is strongly related to the relative polarity value of the storage solvent than their log
P values. After the immobilized tyrosinase is immersed in anhydrous hexane at 100 °C for 96 hours, the immobilized tyrosinase still retains over 60% activity. Conversely, the immobilized tyrosinase loses the activity immediately in water at 100 °C. This indicated that the immobilized tyrosinase could keep its catalytic structure and be used as a synthetic catalyst in anhydrous organic solvent at high temperature. This research provides a lot of information for mimicking enzyme reactions.
 |
| Fig. 4 (A) The half-life of the immobilized tyrosinase vs. the log P values of the organic solvent, (B) the half-life of the immobilized tyrosinase vs. the relative polarity values of the organic solvent. The organic solvent from right to left is methanol, ethanol, acetonitrile, acetone, methyl acetate, tetrahydrofuran, methyl t-butyl ether, toluene and hexane. | |
Conclusion
Triethylaminoethyl cellulose (TEAE-Cellulose) was selected as the best immobilization platform from six kinds of materials. Compared with traditional immobilization materials (glass beads), the activity of tyrosinase immobilization onto TEAE-Cellulose was two times higher than that of immobilization onto glass beads. The immobilized tyrosinase showed potential to be a catalyst in organic solvent without water. The half-life of the dry immobilized tyrosinase in organic solvent was strongly related to the polarity of the solvent rather than the log
P value of the organic solvent. This research could be valuable for the design of new biocatalysts.
Conflicts of interest
There are no conflicts to declare.
Acknowledgements
This study was supported by China Scholarship Council, the National Natural Science Foundation of China (No. 21307161, 61335008 and 61874137). Authors would like to thank Alexander M. Klibanov who gave lots of suggestion in experiment details.
References
- K. V. N. Esguerra and J. P. Lumb, Selectivity in the Aerobic Dearomatization of Phenols: Total Synthesis of Dehydronornuciferine by Chemo- and Regioselective Oxidation, Angew. Chem., Int. Ed., 2018, 57(6), 1514–1518 CrossRef CAS PubMed.
- D. Ma, Z. C. Tu, H. Wang, L. Zhang, N. He and D. J. McClements, Mechanism and kinetics of tyrosinase inhibition by glycolic acid: a study using conventional spectroscopy methods and hydrogen/deuterium exchange coupling with mass spectrometry, Food Funct., 2017, 8(1), 122–131 RSC.
- Z. Ashraf, M. Rafiq, H. Nadeem, M. Hassan, S. Afzal, M. Waseem, K. Afzal and J. Latip, Carvacrol derivatives as mushroom tyrosinase inhibitors; synthesis, kinetics mechanism and molecular docking studies, PLoS One, 2017, 12(5), 1–7 CrossRef PubMed.
- M. Rolff, J. Schottenheim, H. Decker and F. Tuczek, Copper-O2 reactivity of tyrosinase models towards external monophenolic substrates: molecular mechanism and comparison with the enzyme, Chem. Soc. Rev., 2011, 40(7), 4077–4098 RSC.
- R. Z. Kazandjian and A. M. Klibanov, Regioselective Oxidation of Phenols Catalyzed by Polyphenol Oxidase in Chloroform, J. Am. Chem. Soc., 1985, 107(19), 5448–5450 CrossRef CAS.
- A. M. Klibanov, Enzyme-Catalyzed Processes in Organic-Solvents, Ann. N. Y. Acad. Sci., 1987, 501, 129 CrossRef.
- S. G. Burton, Biocatalysis with Polyphenol Oxidase – a Review, Catal. Today, 1994, 22(3), 459–487 CrossRef CAS.
- P. V. Iyer and L. Ananthanarayan, Enzyme stability and stabilization – aqueous and non-aqueous environment, Process Biochem., 2008, 43(10), 1019–1032 CrossRef CAS.
- A. Zaks and A. M. Klibanov, The Effect of Water on Enzyme Action in Organic Media, J. Biol. Chem., 1988, 263(17), 8017–8021 CAS.
- E. Rubio, A. Fernandezmayorales and A. M. Klibanov, Effect of the Solvent on Enzyme Regioselectivity, J. Am. Chem. Soc., 1991, 113(2), 695–696 CrossRef CAS.
- D. A. Cowan and R. Fernandez-Lafuente, Enhancing the functional properties of thermophilic enzymes by chemical modification and immobilization, Enzyme Microb. Technol., 2011, 49(4), 326–346 CrossRef CAS PubMed.
- R. Fernandez-Lafuente, Stabilization of multimeric enzymes: Strategies to prevent subunit dissociation, Enzyme Microb. Technol., 2009, 45(6–7), 405–418 CrossRef CAS.
- R. Plothe, I. Sittko, F. Lanfer, M. Fortmann, M. Roth, V. Kolbach and J. C. Tiller, Poly(2-ethyloxazoline) as Matrix for Highly Active Electrospun Enzymes in Organic Solvents, Biotechnol. Bioeng., 2017, 114(1), 39–45 CrossRef CAS PubMed.
- F. S. Liao, W. S. Lo, Y. S. Hsu, C. C. Wu, S. C. Wang, F. K. Shieh, J. V. Morabito, L. Y. Chou, K. C. W. Wu and C. K. Tsung, Shielding against Unfolding by Embedding Enzymes in Metal-Organic Frameworks via a de Novo Approach, J. Am. Chem. Soc., 2017, 139(19), 6530–6533 CrossRef CAS PubMed.
- U. Guzik, K. Hupert-Kocurek and D. Wojcieszynska, Immobilization as a Strategy for Improving Enzyme Properties-Application to Oxidoreductases, Molecules, 2014, 19(7), 8995–9018 CrossRef PubMed.
- K. Min and Y. J. Yoo, Recent progress in nanobiocatalysis for enzyme immobilization and its application, Biotechnol. Bioprocess Eng., 2014, 19(4), 553–567 CrossRef CAS.
- M. M. Talukder, T. Takeyama, Y. Hayashi, J. C. Wu, T. Kawanishi, N. Shimizu and C. Ogino, Improvement in enzyme activity and stability by addition of low molecular weight polyethylene glycol to sodium bis(2-ethyl-L-hexyl)sulfosuccinate/isooctane reverse micellar system, Appl. Biochem. Biotechnol., 2003, 110(2), 101–112 CrossRef CAS PubMed.
- R. C. Rodrigues, C. Ortiz, A. Berenguer-Murcia, R. Torres and R. Fernandez-Lafuente, Modifying enzyme activity and selectivity by immobilization, Chem. Soc. Rev., 2013, 42(15), 6290–6307 RSC.
- E. T. Hwang and M. B. Gu, Enzyme stabilization by nano/microsized hybrid materials, Eng. Life Sci., 2013, 13(1), 49–61 CrossRef CAS.
- K. Labus, A. Turek, J. Liesiene and J. Bryjak, Efficient Agaricus bisporus tyrosinase immobilization on cellulose-based carriers, Biochem. Eng. J., 2011, 56(3), 232–240 CrossRef CAS.
- M. Bilal, T. Rasheed, Y. Zhao, H. M. N. Iqbal and J. Cui, “Smart” chemistry and its application in peroxidase immobilization using different support materials, Int. J. Biol. Macromol., 2018, 119, 278–290 CrossRef CAS PubMed.
- C. Silva, M. Martins, S. Jing, J. Fu and A. Cavaco-Paulo, Practical insights on enzyme stabilization, Crit. Rev. Biotechnol., 2018, 38(3), 335–350 CrossRef CAS PubMed.
- V. M. Balcao and M. M. D. C. Vila, Structural and functional stabilization of protein entities: state-of-the-art, Adv. Drug Delivery Rev., 2015, 93, 25–41 CrossRef CAS PubMed.
- D. Brady and J. Jordaan, Advances in enzyme immobilisation, Biotechnol. Lett., 2009, 31(11), 1639–1650 CrossRef CAS PubMed.
- D. M. Liu, J. Chen and Y. P. Shi, Tyrosinase immobilization on aminated magnetic nanoparticles by physical adsorption combined with covalent crosslinking with improved catalytic activity, reusability and storage stability, Anal. Chim. Acta, 2018, 1006, 90–98 CrossRef CAS PubMed.
- P. Wang, C. Qi, Y. Yu, J. Yuan, L. Cui, G. Tang, Q. Wang and X. Fan, Covalent Immobilization of Catalase onto Regenerated Silk Fibroins via Tyrosinase-Catalyzed Cross-Linking, Appl. Biochem. Biotechnol., 2015, 177(2), 472–485 CrossRef CAS PubMed.
- T. Jesionowski, J. Zdarta and B. Krajewska, Enzyme immobilization by adsorption: a review, Adsorption, 2014, 20(5–6), 801–821 CrossRef CAS.
- C. Garcia-Galan, A. Berenguer-Murcia, R. Fernandez-Lafuente and R. C. Rodrigues, Potential of Different Enzyme Immobilization Strategies to Improve Enzyme Performance, Adv. Synth. Catal., 2011, 353(16), 2885–2904 CrossRef CAS.
- K. Hernandez and R. Fernandez-Lafuente, Control of protein immobilization: Coupling immobilization and site-directed mutagenesis to improve biocatalyst or biosensor performance, Enzyme Microb. Technol., 2011, 48(2), 107–122 CrossRef CAS PubMed.
- P. Garcia, I. A. Ramallo and R. L. Furlan, Reverse Phase Compatible TLC-Bioautography for Detection of Tyrosinase Inhibitors, Phytochem. Anal., 2017, 28(2), 101–105 CrossRef CAS PubMed.
- L. V. Sigolaeva, S. Y. Gladyr, A. P. Gelissen, O. Mergel, D. V. Pergushov, I. N. Kurochkin, F. A. Plamper and W. Richtering, Dual-stimuli-sensitive microgels as a tool for stimulated spongelike adsorption of biomaterials for biosensor applications, Biomacromolecules, 2014, 15(10), 3735–3745 CrossRef CAS PubMed.
- H. Yagar and S. Kocaturk, Comparison of some biochemical properties of artichoke polyphenol oxidase entrapped in alginate-carrageenan and alginate gels, Artif. Cells, Nanomed., Biotechnol., 2014, 42(4), 268–273 CrossRef CAS PubMed.
- K. Abu-Rabeah, B. Polyak, R. E. Ionescu, S. Cosnier and R. S. Marks, Synthesis and characterization of a pyrrole-alginate conjugate and its application in a biosensor construction, Biomacromolecules, 2005, 6(6), 3313–3318 CrossRef CAS PubMed.
- H. B. Yildiz, E. Sahmetlioglu, A. E. Boyukbayram, L. Toppare and Y. Yagci, Immobilization of tyrosinase and alcohol oxidase in conducting copolymers of thiophene functionalized poly(vinyl alcohol) with pyrrole, Int. J. Biol. Macromol., 2007, 41(3), 332–337 CrossRef CAS PubMed.
- Y. J. Jiang, W. Y. Sun, Y. P. Wang, L. H. Wang, L. Y. Zhou, J. Gao, Y. He, L. Ma and X. Zhang, Protein-based inverse opals: A novel support for enzyme immobilization, Enzyme Microb. Technol., 2017, 96, 42–46 CrossRef CAS PubMed.
- S. K. S. Patel, S. H. Choi, Y. C. Kang and J. K. Lee, Large-scale aerosol-assisted synthesis of biofriendly Fe2O3 yolk-shell particles: a promising support for enzyme immobilization, Nanoscale, 2016, 8(12), 6728–6738 RSC.
- D. Yang, X. Y. Wang, J. F. Shi, X. L. Wang, S. H. Zhang, P. P. Han and Z. Y. Jiang, In situ synthesized rGO-Fe3O4 nanocomposites as enzyme immobilization support for achieving high activity recovery and easy recycling, Biochem. Eng. J., 2016, 105, 273–280 CrossRef CAS.
- S. J. Brooks, L. Coulombel, D. Ahuja, D. S. Clark and J. S. Dordick, Expanding the Scope of Biocatalysis: Oxidative Biotransformations on Solid-Supported Substrates, Adv. Synth. Catal., 2008, 350(10), 1517–1525 CrossRef CAS PubMed.
- J. C. Warrington and B. A. Saville, Tyrosinase inactivation in organic solvents, Biotechnol. Bioeng., 1999, 65(3), 325–333 CrossRef CAS PubMed.
- Z. Qu, W. Na, X. Liu, H. Liu and X. Su, A novel fluorescence biosensor for sensitivity detection of tyrosinase and acid phosphatase based on nitrogen-doped graphene quantum dots, Anal. Chim. Acta, 2018, 997, 52–59 CrossRef CAS PubMed.
- Y. Wang, F. Zhai, Y. Hasebe, H. Jia and Z. Zhang, A highly sensitive electrochemical biosensor for phenol derivatives using a graphene oxide-modified tyrosinase electrode, Bioelectrochemistry, 2018, 122, 174–182 CrossRef CAS PubMed.
- H. Vaghari, H. Jafarizadeh-Malmiri, M. Mohammadlou, A. Berenjian, N. Anarjan, N. Jafari and S. Nasiri, Application of magnetic nanoparticles in smart enzyme immobilization, Biotechnol. Lett., 2016, 38(2), 223–233 CrossRef CAS PubMed.
- X. Lu, X. Wang, L. Wu, L. Wu, R. Dhanjai, L. Fu, Y. Gao and J. Chen, Response Characteristics of Bisphenols on a Metal-Organic Framework-Based Tyrosinase Nanosensor, ACS Appl. Mater. Interfaces, 2016, 8(25), 16533–16539 CrossRef CAS PubMed.
- X. Lian, Y. Huang, Y. Zhu, Y. Fang, R. Zhao, E. Joseph, J. Li, J. P. Pellois and H. C. Zhou, Enzyme-MOF Nanoreactor Activates Nontoxic Paracetamol for Cancer Therapy, Angew. Chem., 2018, 57(20), 5725–5730 CrossRef CAS PubMed.
- P. Zucca, R. Fernandez-Lafuente and E. Sanjust, Agarose and Its Derivatives as Supports for Enzyme Immobilization, Molecules, 2016, 21(11), 1577–1598 CrossRef PubMed.
- E. P. Cipolatti, A. Valerio, R. O. Henriques, D. E. Moritz, J. L. Ninow, D. M. G. Freire, E. A. Manoel, R. Fernandez-Lafuente and D. de Oliveira, Nanomaterials for biocatalyst immobilization – state of the art and future trends, RSC Adv., 2016, 6(106), 104675–104692 RSC.
- J. J. Virgen-Ortiz, S. Peirce, V. G. Tacias-Pascacio, V. Cortes-Corberan, A. Marzocchella, M. E. Russo and R. Fernandez-Lafuente, Reuse of anion exchangers as supports for enzyme immobilization: Reinforcement of the enzyme-support multiinteraction after enzyme inactivation, Process Biochem., 2016, 51(10), 1391–1396 CrossRef CAS.
- O. Barbosa, C. Ortiz, A. Berenguer-Murcia, R. Torres, R. C. Rodrigues and R. Fernandez-Lafuente, Strategies for the one-step immobilization-purification of enzymes as industrial biocatalysts, Biotechnol. Adv., 2015, 33(5), 435–456 CrossRef CAS PubMed.
- K. Ryu and J. S. Dordick, Quantitative and Predictive Correlations for Peroxidase Catalysis in Organic Media, Biotechnol. Tech., 1992, 6(3), 277–282 CrossRef CAS.
Footnote |
† Electronic supplementary information (ESI) available: Brief statement in nonsentence format listing the contents of the material supplied as supporting information. See DOI: 10.1039/c8ra07559j |
|
This journal is © The Royal Society of Chemistry 2018 |
Click here to see how this site uses Cookies. View our privacy policy here.