DOI:
10.1039/C8RA07115B
(Paper)
RSC Adv., 2018,
8, 41040-41047
The influence of molecular structure on collision radius for optical sensing of molecular oxygen based on cyclometalated Ir(III) complexes†
Received
26th August 2018
, Accepted 3rd December 2018
First published on 7th December 2018
Abstract
Three triphenylamine (TPA) substituted cyclometalated Ir(III) complexes IrA1, IrA2, and IrA3 based on Ir(ppy)3 were synthesized and applied as phosphorescent probes for the monitoring of molecular oxygen. The phosphorescence intensity of all the Ir(III) complexes in tetrahydrofuran (THF) was gradually quenched with an increase of oxygen concentration. The increase of TPA substituents on the meta-position of 2-phenylpyridine (IrA1-IrA3) gradually improved the oxygen sensitivity of cyclometalated Ir(III) complexes. IrA3 showed the highest oxygen sensitivity in THF with a KappSV of 204.8 bar−1 and a limit of detection (LOD) of 0.27 mbar. The relationship between molecular structure and the collision radiuses (σ) of all the Ir(III) complexes has been investigated on the basis of the Demas model and the fundamental expression of luminescence quenching systems by oxygen. The ratio of collision radiuses are σIrA1/σIr(ppy)3 = 1.27 ± 0.05, σIrA2/σIr(ppy)3 = 1.72 ± 0.10, and σIrA3/σIr(ppy)3 = 2.13 ± 0.07, respectively. The introduction and increase of TPA substituents can obviously increase the collision radiuses of cyclometalated Ir(III) complexes which leading to potential oxygen sensitivity. And the incremental effect of collision radiuses caused by the introduction of TPA substituents resulted in outstanding oxygen sensitivity of IrA3. The results demonstrate for the first time evidence between molecular structure and oxygen sensitivity of the emitters for optical sensing.
1. Introduction
Optical sensors have attracted a great deal of interest for oxygen quantification due to the advantages of excellent reversibility, quick response, minimal invasiveness and suitability for monitoring of oxygen in gaseous condition and in solution (dissolved oxygen).1–4 Various formats of optical oxygen sensors have been developed including fibre-optic sensors, micro- or nanoparticles, paints and so on.5–9 Optical oxygen sensors depended on the dynamic (diffusion-controlled) quenching of emitters by molecular oxygen which is characterized by the Stern–Volmer equation (eqn (1))10 |
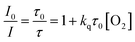 | (1) |
where I and τ are the intensity and decay time of the emitter respectively. I0 and τ0 are the corresponding values in the absence of oxygen. kq means bimolecular quenching constant and [O2] expresses the molar concentration of oxygen. I0/I (or τ0/τ) versus [O2] are linear with slopes of kqτ0. In principle, oxygen sensitivity is roughly in proportion to phosphorescent decay time of the emitter.11–14 State of the art optical oxygen sensors are mainly made up of phosphorescent iridium, platinum complexes, BF2, and aluminum chelates, which possess decay time varying in long range of microseconds to milliseconds.15–19
To prevent potential interference and loss of emitters, polymer matrices are often used as permeation-selective barrier.20–24 In eqn (2), the oxygen concentration in the polymer matrix is proportional to the oxygen partial pressure (pO2) just above the film. And the proportionality constant SO2 is the oxygen solubility in organic solvents and polymer matrices defined by Henry's law.25
Because quenching of phosphorescence is caused by diffusion between emitters and molecular oxygen, the bimolecular quenching constant kq by the expression (eqn (3))26
|
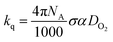 | (3) |
DO2 is the diffusion coefficient of oxygen in solvents or matrices, and
NA is Avogadro's constant.
α represents the probability that a collision leads to quenching. Many authors set
α equal to unity for phosphorescence quenching by oxygen in analyzing data.
27–31 σ is the collision radius of an emitter molecule to molecular oxygen. Upon substitution of
eqn (2) and
(3) into
(1), the fundamental expression (
eqn (4)) governing luminescence quenching by oxygen for systems in equilibrium with oxygen was obtained.
26 |
 | (4) |
On one hand, oxygen sensitivity could be effectively enhanced by using of high oxygen permeable matrix. The extent of quenching markedly depends on the oxygen permeability (PO2) of the matrix, where PO2 is defined as the product of DO2 and SO2 (PO2 = DO2SO2). Much work so far has focused on the modification of the matrices with fluorinated substituents, etc.32–35 On the other hand, decay time (τ0) of the emitters also determined oxygen sensitivity of the sensors. Considerable research efforts have been devoted to the design and synthesis of emitters with long triplet decay time.3,11,36,37 Borisov and co-workers reported an aluminium chelate Al(HBANPF)3 with ultra-long decaying phosphorescence (350 ms). Al(HBANPF)3 which were immobilized in perfluorinated polymers can monitor oxygen in nearly anoxic system.3 Zhao et al. investigated the oxygen sensitivity of two dual-emissive Pt(II) complexes Pt-1 and Pt-2. And Pt-2 with long phosphorescent decay time (3.75 μs) exhibited higher oxygen sensitivity (136.0 bar−1) compared with short-decay Pt-1 (1.53 μs, 45.1 bar−1).11 Besides PO2 and τ0, the collision radius (σ) of the emitter is the third factor to oxygen sensitivity of emitters which was often ignored by researchers. In some cases, oxygen sensitivity and decay time of emitters are not proportional in the same microenvironment (emitters were immobilized in the same matrix with the same preparation method).7,10,22,36–43 For instance, neutral blue emitting cyclometalated Ir(III) complexes Ir-1 and Ir-2 were immobilized in a nanostructured aluminum oxide–hydroxide matrix named AP200/19. Ir-2 with long phosphorescent decay time showed lower oxygen sensitivity compared to short-decay Ir-1 (Fig. 1).40 Phosphorescent decay time of Cu(I) complex Cu-2 is 17-fold longer than Cu-1, however the oxygen sensitivity of Cu-2 is 72% of Cu-1 (Fig. 1).44 Obviously, changes of molecular structure of the emitter lead to the changing of the collision radius, and then affect oxygen sensitivity. For quantification of low concentrations or low partial pressures of oxygen, researchers would like to design phosphorescent emitters with long decay time and then immobilized them in the matrices with extremely high oxygen permeability. To the best of our knowledge, no systematic study concerning how change of molecular structure affect the collision radius (σ) of a phosphorescent emitter for optical oxygen sensing.
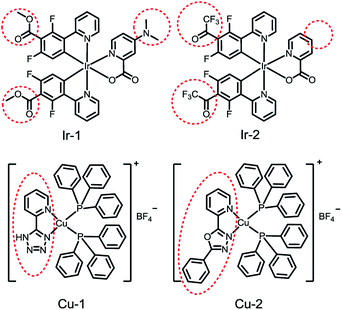 |
| Fig. 1 Chemical structure of phosphorescent complexes Ir-1, Ir-2, Cu-1, and Cu-2. Structure variations of intraligands of Ir-1 and Ir-2, Cu-1 and Cu-2 are showed in red circles. | |
Triphenylamine (TPA) substituent is an important structural motif in numerous dye-sensitized solar cells (DSSCs) and organic electroluminescence materials because of features, such as non-co-planarity of the three phenyl substituents, strong electron-donating nature and high light-to-electrical energy.45,46 Herein, we report a systematic investigation of the relationship between the molecular structure and the collision radius (σ) of the emitter by modification of cyclometalated Ir(III) complexes Ir(ppy)3 with incremental introduction of TPA substituents. Chemical structures of cyclometalated Ir(III) complexes IrA1, IrA2, IrA3 and Ir(ppy)3 are shown in Scheme 1.
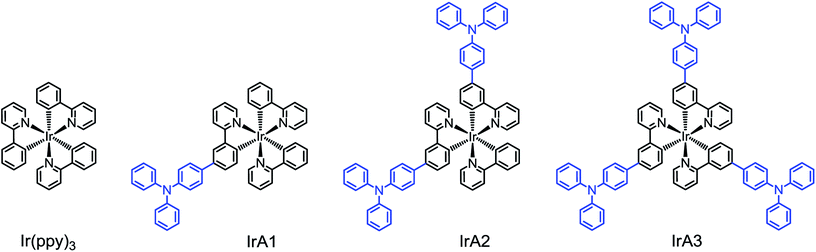 |
| Scheme 1 Structures of the cyclometalated Ir(III) complexes. | |
2. Result and discussion
2.1. Photophysical and electrochemical property
The photophysical properties of IrA1–IrA3 and Ir(ppy)3 are exhibited Table 1. The absorptions of 250–375 nm which belong to π–π* transitions in intraligands enhanced with the incremental introduction of TPA substituents on meta-position of 2-phenylpyridine ligands (Fig. 2). The phenomenon also caused the increase of phosphorescence quantum efficiency of Ir(ppy)3 (0.40), IrA1 (0.48), IrA2 (0.49), and IrA3 (0.53) (Table 1). The transient phosphorescence decay for each Ir(III) complex in THF was mono-exponential, and the lifetimes ranged from 2.38 μs to 2.93 μs. Compared to Ir(ppy)3 (2.38 μs), phosphorescent lifetime of IrA1 (2.93 μs) became longer with introducing a TPA substituent on 2-phenylpyridine. Notably, the incremental introduction of TPA substituents on 2-phenylpyridines of Ir(ppy)3 gradually shortened the lifetimes of cyclometalated Ir(III) complexes. Phosphorescent lifetimes of all the Ir(III) complexes are in the sequence of IrA1 > IrA2 > IrA3 > Ir(ppy)3 (Fig. S1, see ESI†). The emission maxima for all the Ir(III) complexes are in region from 511 to 522 nm. Based on Ir(ppy)3, the emission maxima of IrA1–IrA3 shifts to a longer wavelength when increasing TPA substituents at the meta-position on benzene ring of 2-phenylpyridine ligands. The change in emission color of all the Ir(III) complexes were quantified with CIE coordinates of Ir(ppy)3 (0.2750, 0.6268), IrA1 (0.2977, 0.6354), IrA2 (0.3184, 0.6228), and IrA3 (0.3164, 0.6341) in Fig. S2 (see ESI†). The electrochemical properties of IrA1–IrA3 and Ir(ppy)3 were examined using cyclic voltammetry (Fig. S3, see ESI†). All the cyclometalated Ir(III) complexes showed reversible oxidation waves. The oxidation potentials of IrA1-IrA3 and Ir(ppy)3 are 0.78 V, 0.77 V, 0.78 V, and 0.84 V, respectively, which reflected the donor character of TPA groups. The frontier orbital energy levels of all the Ir(III) complexes are given in Table S1 (see ESI†).
Table 1 Photophysical data of all the Ir(III) complexes
Complexes |
Absorption (293 K) λabs a(nm) |
Emission maxima λem (nm) (293 K) |
Φpb |
τ c(μs) |
Measured in THF at a concentration of 10−5 M and log ε values are shown in parentheses and extinction coefficients (104 M−1 cm−1) are shown in parentheses. In degassed THF relative to [Ir(ppy)3] (Φp = 0.40), (λex = 400 nm). Measured in degassed THF at a sample concentration of ca. 10−5 M and the excitation wavelength was set at 355 nm for all the samples at 293 K. |
Ir(ppy)3 |
250 (1.87), 284 (3.75), 379 (1.12), 411 (0.71), 456 (0.27), 490 (0.12) |
511 |
0.40 |
2.38 |
IrA1 |
249 (1.71), 287 (4.56), 340 (3.37), 412 (0.78), 444 (0.32), 488 (0.09) |
520 |
0.48 |
2.93 |
IrA2 |
254 (1.27), 287 (4.12), 334 (5.07), 405 (0.60), 480 (0.10) |
522 |
0.49 |
2.51 |
IrA3 |
262 (4.18), 296 (8.42), 332 (9.48), 404 (1.32), 472 (0.27) |
522 |
0.53 |
2.42 |
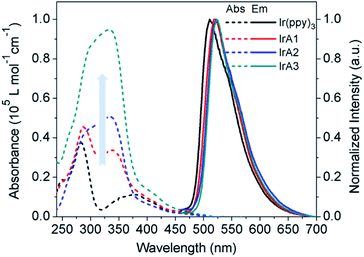 |
| Fig. 2 Absorption and emission spectra of IrA1–IrA3 and Ir(ppy)3; 10−5 M in degassed THF, 25 °C. | |
2.2. Theoretical calculations
Density functional theory (DFT) calculations were performed in order to estimate the ground-state energy levels and electron density distributions of the orbitals of IrA1–IrA3 and Ir(ppy)3.47 And the ground-state geometry of were optimized under the polarizable continuum model (PCM, solvent = THF) of the self-consistent reaction field (SCRF). The HOMO and LUMO isosurfaces displayed in Fig. 3 have π symmetry with opposite phases above and below the molecular plane. The LUMOs of IrA1–IrA3 and Ir(ppy)3 consist of a mixture of 2-phenylpyridine moieties. The HOMO of Ir(ppy)3 is predominantly distributed on the benzene rings of 2-phenylpyridine ligands and Pt atom. The TPA moieties, benzene rings of 2-phenylpyridine ligands and Pt atoms contribute the HOMOs of IrA1–IrA3. The introduction of a TPA substituent on 2-phenylpyridine caused the delocalization of electron from 2-phenylpyridine moieties to the TPA moiety with energy rise (0.29 eV) of HOMO of IrA1. While the incremental introduction of TPA substituents on 2-phenylpyridines have little effect on the HOMOs of IrA2 and IrA3 (Fig. 3).
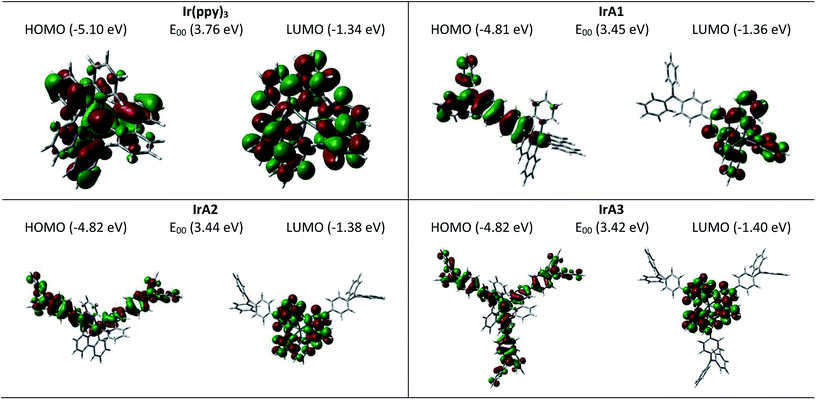 |
| Fig. 3 HOMOs and LUMOs of IrA1–IrA3 and Ir(ppy)3 for the ground state geometry. Calculated by DFT/PCM = THF at the B3LYP/6-31G(d)/LanL2DZ level using Gaussian 16. | |
The low-lying excited states of the singlet (S1) and the triplet (T1) of IrA1–IrA3 and Ir(ppy)3 were also studied with the time-dependent DFT calculations under PCM model (solvent = THF) based on the optimized ground state geometry (Table 2).47 The calculated S1 to T1 energy gaps of IrA1–IrA3 and Ir(ppy)3 are 0.33 eV, 0.31 eV, 0.30 eV, and 0.29 eV, respectively. The orbitals are involved in HOMO−3, HOMO−2, HOMO−1, HOMO, LUMO, and LUMO+1. The S1 and T1 states of all the Ir(III) complexes were mainly assigned as HOMO to LUMO of electron transition. Similar to Ir(ppy)3, the S1 state and T1 state of IrA3 were rendered with considerable nature of IL, mixed with MLCT character. The S1 state and T1 state of IrA1 were rendered with nature of LLCT, MLCT character. And the S1 state and T1 state of IrA2 were rendered with nature of IL, LLCT, mixed with MLCT character (Table 2 and Fig. S4 see ESI†).
Table 2 Electronic excitation energies, oscillator strengths (f), configuration-interaction coefficients (CI), and orbital transition contribution percentages (Co) of the low-lying electronically excited states of IrA1–IrA3 and Ir(ppy)3
|
Elec. Trans. |
TDDFT//B3LYP/6-31G(d)/LanL2DZ |
Energya |
fb |
Compositionc |
CId |
Coe |
Characterf |
Only the S1 and T1 states are presented. Oscillator strengths. And the oscillator strengths of the T1 states are zero because of the neglect of spin–orbit coupling in time-dependent DFT calculations. Only the main configurations are presented. H and L denote HOMO and LUMO, respectively. The configuration-interaction coefficients (CI) are in absolute values. The contribution percentages of orbital transitions. Only the orbital pairs whose Co values above 10% are presented. IL: intraligand, LLCT: ligand-to-ligand charge transfer, MLCT: metal-to-ligand charge transfer. |
Ir(ppy)3 |
S0 → S1 |
3.00 eV, 413 nm |
0.0109 |
H → L |
0.69705 |
97.18% |
IL, MLCT |
S0 → T1 |
2.71 eV, 457 nm |
0.0000 |
H → L |
0.54221 |
58.80% |
IL, MLCT |
IrA1 |
S0 → S1 |
2.92 eV, 425 nm |
0.0238 |
H → L |
0.57752 |
66.71% |
LLCT, MLCT |
H-1 → L |
0.30978 |
19.19% |
LLCT, MLCT |
S0 → T1 |
2.59 eV, 479 nm |
0.0000 |
H → L |
0.40814 |
33.32% |
LLCT, MLCT |
H → L+1 |
−0.35624 |
25.38% |
LLCT, MLCT |
IrA2 |
S0 → S1 |
2.89 eV, 429 nm |
0.0133 |
H → L |
0.60632 |
73.52% |
IL, LLCT, MLCT |
H−2 → L |
0.25367 |
12.87% |
LLCT, MLCT |
S0 → T1 |
2.58 eV, 481 nm |
0.0000 |
H → L |
0.42034 |
35.34% |
IL, LLCT, MLCT |
H−1 → L+1 |
0.28375 |
16.10% |
IL |
IrA3 |
S0→S1 |
2.88 eV, 431 nm |
0.0059 |
H → L |
0.62533 |
78.21% |
IL, MLCT |
H−3 → L |
0.29739 |
17.69% |
IL, MLCT |
S0 → T1 |
2.58 eV, 481 nm |
0.0000 |
H → L |
0.42993 |
36.97% |
IL, MLCT |
H−3 → L |
0.26116 |
13.64% |
IL, MLCT |
2.3. Oxygen sensing
Purified tetrahydrofuran (THF) was selected as dispersion phase for the bimolecular quenching process (Fig. 4). The emission of all the Ir(III) complexes obviously weaken with the increase of oxygen concentration. Above 83% of original intensity was quenched with 6.8% oxygen in the air volume. Notably, the emission of IrA3 was almost completely quenched (above 90% of original intensity) at the oxygen concentration of 6.8% which showed high oxygen sensitivity.
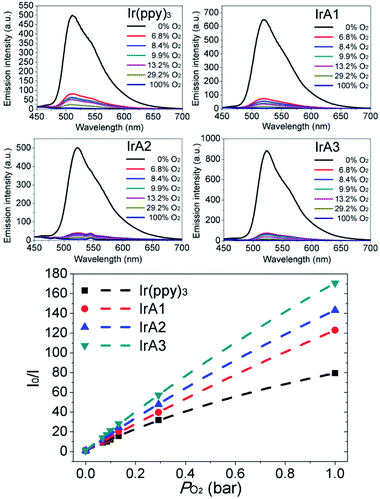 |
| Fig. 4 Dependence of emission spectra and Stern–Volmer plots of all the Ir(III) complexes; 10−5 M in THF on oxygen concentration of 0–100%, 25 °C. | |
Demas et al. have investigated the quenching mechanism of oxygen of several emitters in a lot of polymer matrices.48,49 The results showed that heterogeneous microenvironment exists in the emitter-contained matrix. The Demas' model48 was introduced to fit the Stern–Volmer plots (SVPs) of all the Ir(III) complexes in THF (Fig. 4) which reads as follows in eqn (5)
|
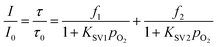 | (5) |
f1 and f2 are fractions of two microenvironments of the heterogeneous optical sensing system, respectively, which are defined as f1 + f2 being 1. f1 is the quenchable fraction with quenching rate constant KSV1, and f2 is the fraction which is not quenched at all with quenching rate constant KSV2. Weighted quenching constant KappSV (KappSV = f1 × KSV1 + f2 × KSV2) is the guide of oxygen sensitivity of an optical oxygen sensor. The Demas' model is well-suited to describe homogeneity of oxygen sensing system. The Stern–Volmer constants for all the Ir(III) complexes in THF are summarized in Table S2 (see ESI†). The I0/I100 of IrA1-IrA3 and Ir(ppy)3 are 122.9, 143.2, 170.8, and 79.4. And KappSV are 138.6 bar−1, 171.4 bar−1, 204.8 bar−1, and 119.6 bar−1, respectively. IrA3 showed outstanding oxygen sensitivity in THF. Contrary to the sequence of phosphorescent lifetimes, the oxygen sensitivities are in the following order: IrA3 > IrA2 > IrA1 > Ir(ppy)3. Compared to Ir(ppy)3, the introduction of TPA substituents on cyclometalating ligand 2-phenylpyridine improved oxygen sensitivity of cyclometalated Ir(III) complexes. On the other hand, the increase of TPA substituents on meta-position of 2-phenylpyridine ligands (IrA1–IrA3) gradually improved the oxygen sensitivity (Fig. 4). Large quenchable fractions (f1) of IrA1 (0.999), IrA2 (0.998), and IrA3 (0.998) are higher than Ir(ppy)3 (0.985) which exhibited remarkable linearity of SVPs (Table S2, see ESI†). With the introduction of TPA substituents at meta-position on 2-phenylpyridine ligands, f1 values were steadily improved which caused more homogeneous microenvironment (high oxygen sensitivity) of cyclometalated Ir(III) complexes. The limit of detection (LOD) of all the cyclometalated Ir(III) complexes in THF was calculated of IrA1 (0.39 mbar), IrA2 (0.30 mbar), IrA3 (0.27 mbar) compared to Ir(ppy)3 (0.43 mbar) (see ESI†).
From eqn (4), the ratio of collision radiuses (σ1/σ2) of two emitters with different molecular structure under homogeneous microenvironment and uniform oxygen partial pressure is obtains by the expression, reads as (eqn (6))
|
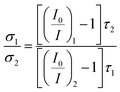 | (6) |
The relationship between molecular structure and the collision radius have been investigated by parallelly measuring data of I0 and I100 five times. And results of bimolecular quenching process in THF and phosphorescent decay time of IrA1–IrA3 and Ir(ppy)3 were employed for analyzing the variation of collision radiuses (Fig. 5 and Table S3, see ESI†). The ratio of collision radiuses are σIrA1/σIr(ppy)3 = 1.27 ± 0.05, σIrA2/σIr(ppy)3 = 1.72 ± 0.10, and σIrA3/σIr(ppy)3 = 2.13 ± 0.07, respectively. Comparision with Ir(ppy)3, the collision radius of IrA1 can be obviously increased with the introduction of a TPA substituent at meta-position on benzene ring of 2-phenylpyridine ligand. And compared to IrA1, the progressive increase of TPA substituents on the rest of 2-phenylpyridine ligands strongly increased the collision radius of cyclometalated Ir(III) complexes (IrA2 and IrA3) as well which leading to higher collision probability between cyclometalated Ir(III) complexes and molecular oxygen, and provided potential oxygen sensitivity.
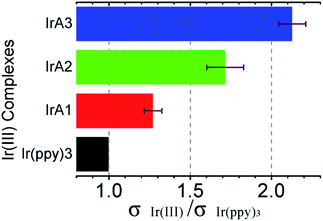 |
| Fig. 5 The variation of collision radiuses of IrA1–IrA3 via Ir(ppy)3 for bimolecular quenching process. | |
As a practical performance, IrA3 was composited with a thin film of silicon gel supported on a glass slide to construct a simple oxygen sensor. The phosphorescence of IrA3 oxygen sensor was totally quenched in air atmosphere and emitted bright yellow-green light under N2 stream. The quenching-emission response of IrA3 oxygen sensor is ultrafast and fully reversible that a flame-like light spot can be seen (Fig. 6).
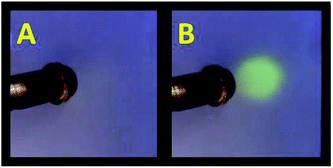 |
| Fig. 6 (A) Photographs of a IrA3 oxygen sensor excited at 365 nm under air (B) with a nitrogen stream in copper pipe. | |
3. Conclusions
In conclusion, three TPA substituted cyclometalated Ir(III) complexes IrA1, IrA2, and IrA3 based on Ir(ppy)3 were synthesized and applied as phosphorescent probes for analysis of molecular oxygen. The phosphorescent intensity of Ir(III) complexes in THF was drastically changed by the oxygen concentration. IrA3 demonstrated the highest oxygen sensitivity with I0/I100 of 170.8, KappSV of 204.8 bar−1, and LOD of 0.27 mbar. With the incremental introduction of TPA substituents at meta-position on 2-phenylpyridine ligands of Ir(ppy)3, the oxygen sensitivities of IrA1–IrA3 were obviously improved, and the f1 values were gradually increased which caused more homogeneous oxygen sensing system and high oxygen sensitivity. The relationship between molecular structure and the collision radius of the emitter for optical oxygen sensing have been investigated and quantized through the cooperation of the Demas' model and the fundamental expression of luminescence quenching systems by oxygen. The incremental introduction of TPA substituents could effectively increase the collision radiuses of cyclometalated Ir(III) complexes which leading to more likely collision between the emitter and molecular oxygen, and provided high oxygen sensitivity. This research could potentially be applied as new prospect for molecular structure design of the emitter with high oxygen sensitivity for optical monitoring of molecular oxygen.
4. Experimental
4.1. Materials and methods
Unless otherwise noted, all the cross-coupling reactions for preparing the ligands were carried out in air. 2-Bromopyridine, (3-bromophenyl)boronic acid, (4-(diphenylamino)phenyl)boronic acid, phenylboronic acid, and iridium(III) chloride trihydrate were purchased from Adamas. Other chemicals were purchased from commercial sources and used without further purification. 1H NMR and 13C NMR spectra were recorded on a BRUKER AVANCE III HD 500 MHz spectrophotometer. Mass spectra were recorded with a MALDI micro MX spectrometer. Phosphorescence lifetimes were determined on a Laser Flash Photolysis (LFP) (Dalian Institute of Chemical Physics). Absorption spectra were recorded by cary series 5000 UV-Vis-NIR spectrometer (Agilent Technologies). Emission spectra were recorded by cary eclipse G9800A fluorescence spectrophotometer (Agilent Technologies). Cyclic voltammograms were recorded on an electrochemical workstation PARSTAT 2273 (Princeton Applied Research) at room temperature.
4.2. Synthesis section
Synthetic procedure of 2-(3-bromophenyl)pyridine. A mixture of 2-bromopyridine (2.0 mmol, 191 μL), 1.5 equiv. of (3-bromophenyl)boronic acid (3.0 mmol, 482 mg), 2 equiv. of K2CO3 (4.0 mmol, 548 mg), Pd(OAc)2 (1.5 mol%, 0.03 mmol, 6.74 mg), ethanol/water (6 mL/2 mL) was stirred at 80 °C in air for indicated time. The reaction mixture was added to brine (15 mL) and extracted four times with ethyl acetate (4 × 15 mL). The solvent was concentrated and the product 2-(3-bromophenyl)pyridine was isolated by short-column chromatography on silica gel (200–300 mesh).
2-(3-Bromophenyl)pyridine12. Yield: 53.2%; colorless oil. 1H NMR (500 MHz, CDCl3): δ 8.70 (d, 1H), 8.17 (s, 1H), 7.91 (d, 1H), 7.77 (td, 1H), 7.71 (d, 1H), 7.54 (dd, 1H), 7.34 (t, 1H), 7.29–7.25 (m, 1H).
Synthetic procedure of ligand N,N-diphenyl-3′-(pyridin-2-yl)-[1,1′-biphenyl]-4-amine (L1). A mixture of 2-(3-bromophenyl)pyridine (0.25 mmol, 58.25 mg), 1.5 equiv. of (4-(diphenylamino)phenyl)boronic acid (0.375 mmol, 108.40 mg), 2 equiv. of K2CO3 (0.5 mmol, 69 mg), Pd(OAc)2 (1.5 mol%, 0.00375 mmol, 0.84 mg), ethanol/water (3 mL/1 mL) was stirred at 80 °C in air for indicated time. The reaction mixture was added to brine (15 mL) and extracted four times with ethyl acetate (4 × 15 mL). The solvent was concentrated and the product L1 was isolated by short-column chromatography on silica gel (200–300 mesh).
L1. Yield: 84.2%; white solid. 1H NMR (500 MHz, CDCl3) δ 8.71 (s, 1H), 8.20 (d, 1H), 7.92 (d, 1H), 7.77 (d, 2H), 7.63–7.50 (m, 4H), 7.26 (d, 5H), 7.17–7.11 (m, 6H), 7.07–6.98 (m, 2H). 13C NMR (126 MHz, CDCl3) δ 157.5, 149.7, 147.7, 147.3, 141.2, 139.9, 136.8, 135.0, 129.3, 129.2, 128.0, 127.3, 125.4, 125.4, 124.4, 123.9, 122.9, 122.2, 120.7. HRMS (EI): calc.: 398.1783 [M]+. Found: 398.1780 [M]+.
Synthetic procedure of IrA1. The cyclometalating ligand 2-phenylpyridine (0.4 mmol, 57 μL) was reacted with 0.6 equiv. IrCl3·3H2O (0.24 mmol, 84.5 mg) in a mixture of 2-ethoxyethanol and water (9 mL/3 mL) at 105 °C for 24 h to afford an iridium dimer, which was subsequently reacted with 0.6 equiv. of the cyclometalating ligand L1 (0.24 mmol, 95.5 mg) and 5 equiv. of Na2CO3 (2 mmol, 212 mg) in glycerol (10 mL) at 200 °C for 48 h.
IrA1. Yield: 73.6%; yellow acicular microcrystal. 1H NMR (500 MHz, CDCl3) δ 7.96 (td, 2H), 7.86 (d, 4H), 7.64 (d, 2H), 7.58–7.54 (m, 2H), 7.51 (dd, 2H), 7.27–7.20 (m, 4H), 7.11 (d, 10H), 6.98 (dd, 3H), 6.85 (dd, 8H). 13C NMR (126 MHz, CDCl3) δ 166.7, 166.6, 161.3, 147.9, 147.1, 147.1, 145.9, 144.3, 143.7, 137.4, 137.1, 135.9, 129.9, 129.2, 127.1, 124.7, 124.0, 123.9, 122.5, 122.1, 121.9, 119.9, 119.8, 118.9, 118.8. HRMS (EI): calc.: 898.2647 [M]+. Found: 898.2646 [M]+.
Synthetic procedure of IrA2. The cyclometalating ligand L1 (0.4 mmol, 159.2 mg) was reacted with 0.6 equiv. IrCl3·3H2O (0.24 mmol, 84.5 mg) in a mixture of 2-ethoxyethanol and water (9 mL/3 mL) at 105 °C for 24 h to afford an iridium dimer, which was subsequently reacted with 0.6 equiv. of the cyclometalating ligand 2-phenylpyridine (0.24 mmol, 34.2 μL) and 5 equiv. of Na2CO3 (2 mmol, 212 mg) in glycerol (10 mL) at 200 °C for 48 h.
IrA2. Yield: 71.0%; yellow acicular microcrystal. 1H NMR (500 MHz, CDCl3) δ 7.96 (t, 4H), 7.85 (d, 4H), 7.61–7.54 (m, 6H), 7.50 (dd, 4H), 7.26–7.20 (m, 8H), 7.10 (d, 12H), 6.98 (dd, 8H), 6.85 (d, 4H). 13C NMR (126 MHz, CDCl3) δ 166.8, 166.7, 148.0, 147.3, 147.2, 146.0, 144.4, 143.8, 137.5, 137.2, 137.0, 136.1, 132.2, 130.0, 129.9, 129.3, 128.6, 127.2, 124.8, 124.1, 122.6, 122.2, 120.1, 119.9, 118.9. HRMS (EI): calc.: 1141.3695 [M]+. Found: 1141.3692 [M]+.
Synthetic procedure of IrA3. The cyclometalating ligand L1 (0.4 mmol, 159.2 mg) was reacted with 0.6 equiv. IrCl3·3H2O (0.24 mmol, 84.5 mg) in a mixture of 2-ethoxyethanol and water (9 mL/3 mL) at 105 °C for 24 h to afford an iridium dimer, which was subsequently reacted with 0.6 equiv. of the cyclometalating ligand L1 (0.24 mmol, 95.5 mg) and 5 equiv. of Na2CO3 (2 mmol, 212 mg) in glycerol (10 mL) at 200 °C for 48 h.
IrA3. Yield: 68.7%; yellow acicular microcrystal. 1H NMR (500 MHz, CDCl3) δ 7.98 (d, 3H), 7.87 (s, 3H), 7.61 (dd, 6H), 7.50 (d, 6H), 7.27–7.21 (m, 9H), 7.11 (d, 24H), 6.98 (dd, 7.4 Hz, 9H), 6.92–6.88 (m, 3H). 13C NMR (126 MHz, CDCl3) δ 166.8, 166.7, 148.0, 147.3, 146.0, 144.4, 137.5, 136.9, 136.2, 129.3, 128.7, 128.6, 127.1, 124.7, 124.16, 124.1, 122.5, 122.2, 119.0. HRMS (EI): calc.: 1384.4743 [M]+. Found: 1384.4743 [M]+.
4.3. Oxygen sensing
The specific concentrations of oxygen in nitrogen at atmospheric pressure (from 0–100% in volume fraction) were generated with two tube flowmeter. The volumetric flow rate was monitored by bubble flowmeter.10
4.4. IrA3 oxygen sensor
IrA3 was immobilized in a silicon gel plate (the layer thickness of silicon gel is 0.2 ± 0.03 mm; the granularity of silicon gel is 8 ± 2 μm) using thin-layer chromatography technic with IrA3–THF eluent solution (0.05 mg mL−1). The silicon gel oxygen sensor was dried for 4 h to remove THF before oxygen sensing tests.
Conflicts of interest
There are no conflicts to declare.
Acknowledgements
This work was supported by the funds of Liaoning Shihua University (2016XJJ-099, 2017XJJ-001) and the fund of Department of Education Of Liaoning Province (L2017LQN007).
Notes and references
- A. Persson, E. Gross, P. Laurent, K. E. Busch, H. Bretes and M. de Bono, Nature, 2009, 458, 1030 CrossRef CAS PubMed.
- X. D. Wang and O. S. Wolfbeis, Chem. Soc. Rev., 2014, 43, 3666 RSC.
- P. Lehner, C. Staudinger, S. M. Borisov and I. Klimant, Nat. Commun., 2014, 5, 4460 CrossRef CAS PubMed.
- A. Jana, B. J. Crowston, J. R. Shewring, L. K. McKenzie, H. E. Bryant, S. W. Botchway, A. D. Ward, A. J. Amoroso, E. Baggaley and M. D. Ward, Inorg. Chem., 2016, 55, 5623 CrossRef CAS PubMed.
- O. S. Wolfbeis, Adv. Mater., 2008, 20, 3759 CrossRef CAS.
- X.-d. Wang, J. A. Stolwijk, T. Lang, M. Sperber, R. J. Meier, J. Wegener and O. S. Wolfbeis, J. Am. Chem. Soc., 2012, 134, 17011 CrossRef CAS PubMed.
- M. Li, B. Zheng, D. Luo, H. Sun, N. Wang, Y. Huang, J. Dai, D. Xiao, S.-J. Su and Z. Lu, Chem. Commun., 2015, 51, 1926 RSC.
- M. Amela-Cortes, S. Paofai, S. Cordier, H. Folliot and Y. Molard, Chem. Commun., 2015, 51, 8177 RSC.
- Y. Wang, B. Li, Y. Liu, L. Zhang, Q. Zuo, L. Shi and Z. Su, Chem. Commun., 2009, 5868 RSC.
- Y. Xing, C. Liu, X. Song and J. Li, J. Mater. Chem. C, 2015, 3, 2166 RSC.
- Y. Liu, H. Guo and J. Zhao, Chem. Commun., 2011, 47, 11471 RSC.
- Y. Xing, C. Liu, J.-H. Xiu and J.-Y. Li, Inorg. Chem., 2015, 54, 7783 CrossRef CAS PubMed.
- C. Liu, X. Lv, Y. Xing and J. Qiu, J. Mater. Chem. C, 2015, 3, 8010 RSC.
- C. Liu, H. Yu, X. Rao, X. Lv, Z. Jin and J. Qiu, Dyes Pigm., 2017, 136, 641 CrossRef CAS.
- M. Quaranta, S. M. Borisov and I. Klimant, Bioanal. Rev., 2012, 4, 115 CrossRef PubMed.
- O. S. Wolfbeis, J. Mater. Chem., 2005, 15, 2657 RSC.
- Y. Amao, Microchim. Acta, 2003, 143, 1 CrossRef CAS.
- S. Tobita and T. Yoshihara, Curr. Opin. Chem. Biol., 2016, 33, 39 CrossRef CAS PubMed.
- C. A. DeRosa, S. A. Seaman, A. S. Mathew, C. M. Gorick, Z. Fan, J. N. Demas, S. M. Peirce and C. L. Fraser, ACS Sens., 2016, 1, 1366 CrossRef CAS PubMed.
- X. L. Qi, S. Y. Liu, R. B. Lin, P. Q. Liao, J. W. Ye, Z. Lai, Y. Guan, X. N. Cheng, J. P. Zhang and X. M. Chen, Chem. Commun., 2013, 49, 6864 RSC.
- Y. Amao, T. Miyashita and I. Okura, J. Porphyrins Phthalocyanines, 2001, 5, 433 CrossRef CAS.
- C. Liu, X. Song, X. Rao, Y. Xing, Z. Wang, J. Zhao and J. Qiu, Dyes Pigm., 2014, 101, 85 CrossRef CAS.
- B. Lei, L. Wang, H. Zhang, Y. Liu, H. Dong, M. Zheng and X. Zhou, Sens. Actuators, B, 2016, 230, 101 CrossRef CAS.
- N. de Acha, C. Elosúa, D. Martínez, M. Hernáez, I. R. Matías and F. J. Arregui, Sens. Actuators, B, 2017, 239, 1124 CrossRef CAS.
- S.-Y. Liu, X.-L. Qi, R.-B. Lin, X.-N. Cheng, P.-Q. Liao, J.-P. Zhang and X.-M. Chen, Adv. Funct. Mater., 2014, 24, 5866 CrossRef CAS.
- X. Lu and M. A. Winnik, Chem. Mater., 2001, 13, 3449 CrossRef CAS.
- M. Nowakowska, J. Najbar and B. Waligóra, Eur. Polym. J., 1976, 12, 387 CrossRef CAS.
- A. Yekta, Z. Masoumi and M. A. Winnik, Can. J. Chem., 1995, 73, 2021 CrossRef CAS.
- L. K. Patterson, G. Porter and M. R. Topp, Chem. Phys. Lett., 1970, 7, 612 CrossRef CAS.
- J. E. Guillet and M. Andrews, Macromolecules, 1992, 25, 2752 CrossRef CAS.
- J. N. Demas, E. W. Harris and R. P. McBride, J. Am. Chem. Soc., 1977, 99, 3547 CrossRef CAS.
- Y. Amao, K. Asai, T. Miyashita and I. Okura, Polym. Adv. Technol., 2000, 11, 705 CrossRef CAS.
- Y. Amao, T. Miyashita and I. Okura, Analyst, 2000, 125, 871 RSC.
- Y. Amao, T. Miyashita and I. Okura, Anal. Chim. Acta, 2000, 421, 167 CrossRef CAS.
- M. E. Köse, B. F. Carroll and K. S. Schanze, Langmuir, 2005, 21, 9121 CrossRef PubMed.
- W. Wu, C. Cheng, W. Wu, H. Guo, S. Ji, P. Song, K. Han, J. Zhao, X. Zhang, Y. Wu and G. Du, Eur. J. Inorg. Chem., 2010, 2010, 4683 CrossRef.
- W. Wu, W. Wu, S. Ji, H. Guo and J. Zhao, Eur. J. Inorg. Chem., 2010, 2010, 4470 CrossRef.
- W. Wu, W. Wu, S. Ji, H. Guo and J. Zhao, Dalton Trans., 2011, 40, 5953 RSC.
- W. Wu, J. Sun, S. Ji, W. Wu, J. Zhao and H. Guo, Dalton Trans., 2011, 40, 11550 RSC.
- M. Marín-Suárez, B. F. E. Curchod, I. Tavernelli, U. Rothlisberger, R. Scopelliti, I. Jung, D. Di Censo, M. Grätzel, J. F. Fernández-Sánchez, A. Fernández-Gutiérrez, M. K. Nazeeruddin and E. Baranoff, Chem. Mater., 2012, 24, 2330 CrossRef.
- C. Liu, X. Song, Z. Wang and J. Qiu, ChemPlusChem, 2014, 79, 1472 CrossRef CAS.
- C. Liu, H. Yu, Y. Xing, Z. Gao and Z. Jin, Dalton Trans., 2016, 45, 734 RSC.
- H. Yu, C. Liu, X. Lv, J. Xiu and J. Zhao, Dyes Pigm., 2017, 145, 136 CrossRef CAS.
- X.-y. Xu, H.-n. Xiao and A.-P. Deng, Opt. Mater., 2014, 36, 1542 CrossRef CAS.
- Y. Lin, H. Wang, Y. Li, D. Zhu and X. Zhan, J. Mater. Chem. A, 2013, 1, 14627 RSC.
- L. Wang, P. Shen, Z. Cao, X. Liu, Y. Huang, C. Liu, P. Chen, B. Zhao and S. Tan, J. Power Sources, 2014, 246, 831 CrossRef CAS.
- M. J. Frisch, G. W. Trucks, H. B. Schlegel, G. E. Scuseria, M. A. Robb, J. R. Cheeseman, G. Scalmani, V. Barone, B. Mennucci, G. A. Petersson, H. Nakatsuji, M. Caricato, X. Li, H. P. Hratchian, A. F. Izmaylov, J. Bloino, G. Zheng, J. L. Sonnenberg, M. Hada, M. Ehara, K. Toyota, R. Fukuda, J. Hasegawa, M. Ishida, T. Nakajima, Y. Honda, O. Kitao, H. Nakai, T. Vreven, J. A. Montgomery, J. E. Peralta, F. Ogliaro, M. Bearpark, J. J. Heyd, E. Brothers, K. N. Kudin, V. N. Staroverov, R. Kobayashi, J. Normand, K. Raghavachari, A. Rendell, J. C. Burant, S. S. Iyengar, J. Tomasi, M. Cossi, N. Rega, J. M. Millam, M. Klene, J. E. Knox, J. B. Cross, V. Bakken, C. Adamo, J. Jaramillo, R. Gomperts, R. E. Stratmann, O. Yazyev, A. J. Austin, R. Cammi, C. Pomelli, J. W. Ochterski, R. L. Martin, K. Morokuma, V. G. Zakrzewski, G. A. Voth, P. Salvador, J. J. Dannenberg, S. Dapprich, A. D. Daniels, O. Farkas, J. B. Foresman, J. V. Ortiz, J. Cioslowski and D. J. Fox, Gaussian 16, Revision A.03, Gaussian, Inc., Wallingford, CT, 2016 Search PubMed.
- E. Carraway, J. Demas and B. DeGraff, Anal. Chem., 1991, 63, 332 CrossRef CAS.
- E. Carraway, J. Demas, B. DeGraff and J. Bacon, Anal. Chem., 1991, 63, 337 CrossRef CAS.
Footnotes |
† Electronic supplementary information (ESI) available: Phosphorescent emission decay curves and cyclic voltammogram. See DOI: 10.1039/c8ra07115b |
‡ These authors contributed to this work equally. |
|
This journal is © The Royal Society of Chemistry 2018 |
Click here to see how this site uses Cookies. View our privacy policy here.