DOI:
10.1039/C8RA05968C
(Paper)
RSC Adv., 2018,
8, 33301-33313
Comparative study on synchronous adsorption of arsenate and fluoride in aqueous solution onto MgAlFe-LDHs with different intercalating anions†
Received
13th July 2018
, Accepted 15th September 2018
First published on 27th September 2018
Abstract
In this study, a series of MgAlFe-LDHs (Cl−, NO3−, intercalation, and calcined products of a CO32− interlayer) was synthesized and used for adsorption of arsenate and fluoride in individual contaminants and coexisting pollutant systems. Effects of various factors such as initial pH of solution, dosage of materials, coexisting ions, contact time, and initial pollutant concentrations were evaluated. Experimental results showed that different intercalating anions had a significant effect on adsorption performance of arsenate and fluoride in water. The adsorption of arsenate and fluoride on MgAlFe-CLDH, MgAlFe–Cl-LDH or MgAlFe–NO3-LDH can be described by different adsorption isotherm equations. During the simultaneous removal process, arsenate and fluoride competed for adsorption sites of the adsorbent materials, and the fluoride ions had advantages in the competitive adsorption on MgAlFe–Cl-LDH and MgAlFe–NO3-LDH. MgAlFe–NO3-LDH was used to adsorb arsenate and fluoride in coexisting pollution systems (the concentration of each pollutant was 2 mg L−1, the adsorbent dosage was 1.5 g L−1). The remaining arsenic concentration was reduced to less than 10 μg L−1 and the remaining fluoride ion concentration to below 20 μg L−1 which meets the World Health Organization's, EPA's and China's drinking water standards for arsenic and fluoride limits. A possible mechanism is discussed with support from further XRD, SEM, and XPS analysis of the materials after their adsorption.
Introduction
Arsenic and fluoride are contaminants present in natural water environments, and often coexist in groundwater. Especially in some countries, such as China,1 Mexico,2 Pakistan,3,4 Afghanistan,5 India,6 Argentina, and Chile,7 concentrations of arsenic and fluoride in groundwater in some areas are much higher than WHO's and local drinking water standards. Arsenic and fluoride have harmful effects on human health and there are many toxicological studies on arsenic and fluoride coexisting systems.8–10 Some research results have shown that when arsenic and fluoride coexist in water, due to the interaction between them, they will further exacerbate human body neurotoxicity.11
In recent years, the control of pollution resulting from arsenic and fluoride gradually has aroused people's attention.12 Research on the removal of arsenic and fluoride mainly focused on coagulation,13 adsorption,14,15 filtration,16,17 and phytoremediation.18,19 It is known that one of the most promising methods for simultaneous removal of arsenic and fluoride is adsorption because of its low-cost and convenience. Recently, many adsorbents have been used to remove arsenic and fluoride simultaneously, such as granular TiO2–La material,15 aluminum oxide/hydroxide nanoparticles,20 iron oxide nano particles,14 chemically treated laterite,21 brick pieces and marble powder,22 activated red mud,23 and layered double hydroxide.24,25
Layered double hydroxides (LDHs) may be suitable adsorbents for the treatment of arsenic and fluoride complexes pollution in water. Compared to other synthetic adsorbents, the synthesis process of LDHs is relatively simple and less costly. The general formula of LDHs as a layered structure anionic clay is [MII1−xMxIII(OH)2]x+(An−)x/n·mH2O, where MII and MIII are divalent and trivalent cations, respectively while An− denotes an interlayer anion; LDHs have found wide application in the field of pollutant removal in water.26,27 In particular, LDHs have been used to adsorb arsenic or fluoride in single pollutant systems,28 and several LDHs with high adsorption capacity for arsenic or fluoride in water have been reported.29–35 Simultaneous removal of arsenic and fluoride in water is very important for the protection of human health and ecosystems. However, adsorbents with high adsorption capacity for removal of individual contaminants are not necessarily suitable for simultaneous removal of arsenic and fluoride.12 Studies have shown that the simultaneous adsorption of arsenic and fluorine is more efficient with CLDH (calcined product of LDHs).25,36 The fact that the structure of CLDH is prone to structural rebuilding makes the material more recyclable. It also means that arsenic and fluorine are not completely fixed on the material. Application of adsorbents has been hindered due to the influence of other ions in actual water environments and stability after adsorption of pollutants.12 Similarly, limited knowledge is available about comparative adsorption of arsenic and fluoride on LDHs during the simultaneous removal process. A study of the competitive adsorption processes, coexisting ions effect, and possible release after adsorption can facilitate the design of adsorbents for practical application.
Based on our previous research on LDHs,37–40 the objective of this study is to investigate the effect of different intercalation anions on the simultaneous removal of arsenic and fluoride on MgAlFe-LDHs materials in water. Thus, a series of LDHs with different anion intercalation layers were synthesized and the adsorption of arsenate and fluoride on these materials was studied. Effects of various factors in the adsorption process such as initial pH of solution, dosage of materials, coexisting ions, contact time, and initial pollutant concentration were evaluated. A possible mechanism of adsorption and release of arsenic and fluorine after adsorption are discussed. The results may be helpful for the treatment of arsenic and fluoride contaminated water and be beneficial for further understanding the competitive adsorption behavior of arsenate and fluoride on LDHs materials.
Materials and methods
Chemicals
Sodium fluoride and all chemicals used for synthesis were analytical grade and purchased from Sinopharm Group Reagent Co., Ltd. The arsenate (Na2HAsO4·7H2O) was purchased from Sigma-Aldrich with a purity higher than 98%. Stock solutions of 1000 mg L−1 As(V) and F− were prepared with Milli-Q water (18.2 MΩ cm at 25 °C), respectively. Then, working solutions were obtained by diluting the stock solutions with de-ionized water.
Synthesis of LDHs
Mg–Al–Fe-LDHs with a nitrate interlayer were synthesized by a co-precipitation method. First, 0.05 mol Mg(NO3)2·6H2O, 0.045 mol Al(NO3)3·9H2O, and 0.005 mol Fe(NO3)3·9H2O were dissolved in 100 mL of deionized water to obtain a metal ion mixed solution. Then, 0.1 mol NaNO3 and 0.2 mol of NaOH were dissolved in 100 mL of deionized water to obtain an alkali solution. Next, the prepared metal ion mixed solution and the alkali solution were simultaneously dropped into a high-speed stirred reactor containing 50 mL of deionized water while the reactor was charged with nitrogen as a protective gas. Appropriate amounts of NaOH or HCl solution were added to the reactor in order to maintain the pH value around 10. After the reaction was complete, the mixture was stirred for 30 minutes. The resulting suspension was placed in an oven and aged for 24 hours at 353 K. Then, centrifugal separation and washing were carried out. The precipitate was washed repeatedly with deionized water until the pH of the supernatant was neutral. The obtained material was then dried at 353 K and ground to powder and the product was named MgAlFe–NO3-LDH. The MgAlFe–Cl-LDH and MgAlFe–CO3-LDH were also prepared by the same method by using the corresponding chloride or carbonate instead of nitrate. The MgAlFe–CO3-LDH was calcined at 773 K in a muffle furnace for 4 hours and, after cooling, the calcined product MgAlFe-CLDH was obtained. According to the different contents of intercalated ions, the materials were named MgAlFe–NO3-LDH, MgAlFe–Cl-LDH, and MgAlFe-CLDH, respectively.
Characterization and analysis
The aqueous arsenate concentration was determined by inductively coupled plasma optical emission spectrometry (ICP-OES) (720 ES, Agilent Technologies) and atomic fluorescence spectrometry (AFS) (FP6-A, PERSEE). Fluoride concentration was determined by ion chromatography (DIONEX ICS 5000, Thermo Scientific). The materials were characterized by X-ray diffractometer (XRD) (D8 Advance, Bruker), Fourier transfer infrared (FTIR) spectrophotometer (Nicolet 6700, Nicolet), specific surface area analyzer (Autosorb-iQ, Quanta Chrome Instruments), and scanning electron microscopy (SEM) (CM200FEG, PHILIPS and S-4800, HITACHI). Elemental composition and the valence states on the surfaces of the materials were analyzed by X-ray photoelectron spectroscopy (XPS) (EscaLab 250Xi, Thermo Scientific).
Adsorption experiments
Batch adsorption experiments were carried out using a conical flask as an adsorption reaction vessel and placed in a constant temperature shaking incubator at 298 K with 150 rpm. If not specified, the dosage of materials were 0.6 g L−1, the initial pH was adjusted to 6.0, and the adsorption reaction lasted for 24 hours to achieve equilibrium. After reaction, the supernatants were collected and filtered with a 0.22 μm needle filter before measuring the concentrations of residual arsenate and fluoride. All experiments were repeated twice.
In the isothermal experiments, the concentration range of arsenate ranged from 1 mg L−1 to 100 mg L−1. The concentration gradient of fluoride ion was from 1 mg L−1 to 30 mg L−1. The concentration ranges of arsenic and fluoride co-adsorption were consistent with the concentration range of a single pollutant system. In the kinetic and coexisting ion effects experiments, the initial concentrations of arsenic or fluoride were 5 mg L−1. A certain concentration of anionic sodium salt (NaCl, NaNO3, Na2CO3, Na2SO4, Na2HPO4) was added to the aqueous solution containing the contaminants to assess the effect of coexisting anions on adsorption. The initial concentrations were set at 2 mg L−1 in experiments of dosage effects. The pH of the system was adjusted to 6.0. The dosage of adsorbents was set from 0.2 g L−1 to 2.5 g L−1. In the evaluation of the effects of pH on adsorption of arsenic, the initial arsenic concentration was 10 mg L−1, and the initial concentration of fluoride was 5 mg L−1 when assessing the effect of adsorbed fluoride. The pH range was from 3.0 to 10.0.
Desorption experiments
Adsorbents with a dosage of 0.4 g were added to a 50 mL solution where the initial concentration of arsenate or fluoride (C0) was 10 mg L−1. After the adsorption had equilibrated (298 K, 150 rpm, 24 h), the supernatant was taken and used to determine the concentrations of the remaining arsenate and fluoride, and the solid was obtained by centrifugation. Deionized water, NaOH, Na2CO3, and Na2HPO4 solutions were used as the eluent, respectively. Conditions of the desorption test were set as that of the concentration of eluent which was 500 mg L−1, the reaction temperature was 298 K, the oscillation frequency was 150 rpm, and the time of desorption was 24 hours.
Results and discussion
Characterization
XRD patterns of the synthesized LDHs and the calcined material (CLDH) are shown in Fig. 1a. It can be seen from Fig. 1a that the MgAlFe–Cl-LDH and MgAlFe–NO3-LDH materials show a series of characteristic diffraction peaks (003) (006) (015) (018) (110), and (113).41 Because of having been calcined at a high temperature of 778 k, crystal water, free water, lamellar hydroxyl dehydration, and CO32− had all been removed and the corresponding characteristic peaks of the lamellar structure disappeared in the CLDH materials. The diffraction peaks of MgAlFe-CLDH appearing at 2θ of 43° and 63° are Mg–O and Al–O which are characteristic peaks (JCPDES no: 30-794).
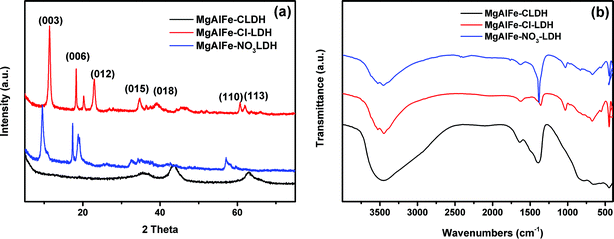 |
| Fig. 1 (a) XRD patterns of MgAlFe-CLDH, MgAlFe–Cl-LDH, and MgAlFe–NO3-LDH. (b) FTIR spectra of MgAlFe-CLDH,MgAlFe–Cl-LDH, and MgAl-e–NO3–LDH. | |
FTIR spectra of the prepared materials are shown in Fig. 1b. The strong and broad absorption peaks in the range of about 3450 cm−1 are derived from the stretching vibration of the hydroxyl groups of the LDHs and the water molecules in the layers, while the bending vibration absorption peaks of the water molecules are at 1639 cm−1, 1384 cm−1 is NO3− antisymmetric stretching vibration, the absorption peak in the low frequency region between wave numbers 500–100 cm−1 is the metal oxygen bond and the metal hydrogen bond,42 namely Mg–O, Mg–OH, Fe–O, Fe–OH, Al–O, and Al–OH.
The N2 adsorption/desorption isotherms of the synthesized materials are shown in Fig. 2a–c. It can be seen from the figures that the adsorption isotherms of these three materials are similar, and the adsorption and desorption curves contain H3 type hysteresis loops,43 which conform to an IV adsorption isotherm in the IUPAC classification, indicating that the three materials are all mesoporous materials.44 It also shows that the three materials have parallel plate structures of the slit hole. At the beginning of cohesion, since the gas–liquid interface is a large plane, capillary condensation occurs only when the pressure is close to the saturated vapor pressure. The specific surface area was calculated by the multi-point BET method. The average pore size was calculated by the BJH method using desorption curve data (Fig. 2d). A summary of results are listed in Table 1. The calculated average pore size also shows that the three materials are mesoporous materials.
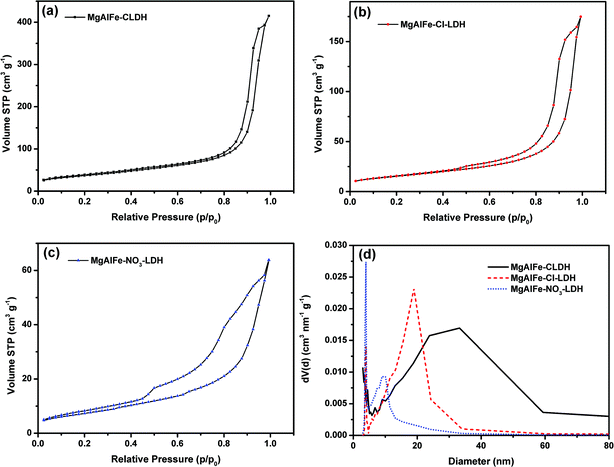 |
| Fig. 2 (a) N2 adsorption/desorption isotherms of MgAlFe-CLDH. (b) N2 adsorption/desorption isotherms of MgAlFe–Cl-LDH. (c) N2 adsorption/desorption isotherms of MgAlFe–NO3-LDH. (d) Pore size distribution of three materials (BJH method). | |
Table 1 Specific surface areas of the synthetic materials, average pore size, and total pore volume
Materials |
BET surface area (m2 g−1) |
C-value in BET equation |
Pore volume (cm3 g−1) |
Average pore size (nm) |
MgAlFe-CLDH |
130.39 |
197.6 |
0.6418 |
24.468 |
MgAlFe–Cl-LDH |
55.08 |
109.7 |
0.2704 |
18.946 |
MgAlFe–NO3-LDH |
27.38 |
53.35 |
0.0987 |
3.924 |
SEM images of the synthesized materials are shown in Fig. 3. It can be seen that MgAlFe–Cl-LDH and MgAlFe–NO3-LDH have sheet-like morphology which shows that the materials have a layered structure. MgAlFe-CLDH has no hierarchical structure and the surface was rough due to decomposition of water and carbon dioxide during the calcination process. Composition of surface elements of the material was analyzed by XPS where it can be seen in Fig. S1–S3† that the metal ratios of MgAlFe-CLDH, MgAlFe–Cl-LDH, and MgAlFe–NO3-LDH were approximately the same.
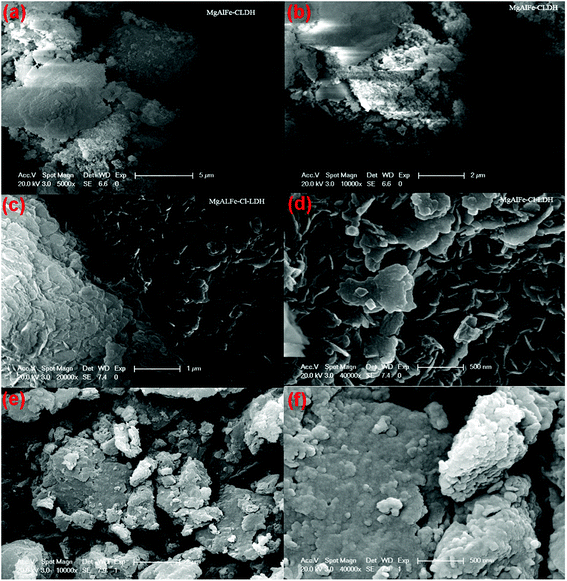 |
| Fig. 3 SEM image of the materials (a) and (b) MgAlFe-CLDH, (c) and (d) MgAlFe–Cl-LDH, (e) and (f) MgAlFe–NO3-LDH. | |
Adsorption of arsenic
Isotherms of arsenic adsorption. In order to understand the adsorption process of arsenate in the three adsorbent materials, an isothermal adsorption experiment was carried out. Langmuir,45,46 Freundlich,47 and Sips48 models were used to analyze the relative data obtained from the adsorption experiment for arsenic and their fitting curves are shown in Fig. 4a–c. The relevant parameters were calculated, as shown in Table S1.†
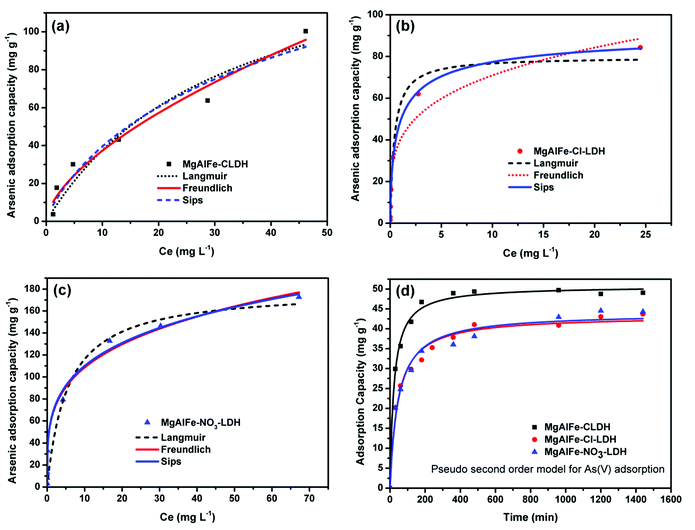 |
| Fig. 4 (a) Isothermal study of adsorption of arsenic with MgAlFe-CLDH. (b) Isothermal study of adsorption of arsenic with MgAlFe–Cl-LDH. (c) Isothermal study of adsorption of arsenic with MgAlFe–NO3-LDH. (d) Kinetic fitting curve of arsenic adsorption on the three materials. | |
The adsorption of arsenate by MgAlFe-CLDH was more consistent with the Freundlich adsorption isotherm equation in the following form:
|
 | (1) |
where
Qe (mg g
−1) is the equilibrium adsorption capacity,
KF (L mg
−1) is the Freundlich constant, and 1/
n is the heterogeneity factor.
The data where As(V) adsorbed onto the MgAlFe–Cl-LDH and MgAlFe–NO3-LDH were more conformed to the Sips equation. The Sips isotherm model follows:
|
 | (2) |
where
Ce (mg L
−1) is the equilibrium concentration,
Qm (mg g
−1) and
Qe (mg g
−1) are the maximum and equilibrium adsorption capacity, respectively,
KS (L mg
−1) is the Sips isotherm constant, and
m is the Sips isotherm exponent.
The Sips adsorption isothermal equation is usually seen as an improved version of the Langmuir equation. The Langmuir model follows:
|
 | (3) |
where
KL (L mg
−1) is the Langmuir adsorption constant.
An interesting result is that the adsorption capacity of arsenic in LDHs intercalated with nitrate is slightly higher than that of CLDH, which is different from previous reports.27,34,49,50 It has been reported that nitrate between the LDHs' layers is most likely to exchange with arsenate compared to other interlayer ions.51 When the concentration of arsenate in the water is higher, then a larger extent of the inter-layer nitrate ion exchange reaction will take place. Thus, the maximum adsorption capacity fitted from the adsorption isotherm curve appears to be close to or slightly higher than that of CLDH material.
Kinetics of arsenic adsorption. The adsorption kinetics of arsenic in MgAlFe-CLDH, MgAlFe–Cl-LDH, and MgAlFe–NO3-LDH are shown in Fig. 4d. It is found that the arsenic adsorption rate of the materials was fast in the first 3 hours, probably because the adsorbent has more active sites in the initial stage. With the prolongation of time, the adsorption sites in the adsorbent were occupied by arsenate, the arsenate concentration in the solution was also reduced, and the reaction rate was reduced until the adsorption reached equilibrium. In order to further explore the adsorption process, a pseudo-first order kinetic model and pseudo-second order kinetic model were used to fit relevant data of the arsenate adsorption kinetics experiments.52The pseudo-first order kinetic model equation follows:
|
ln(Qe − Qt) = ln Qe − K1t
| (4) |
And the pseudo-second order kinetic model equation follows:
|
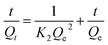 | (5) |
where
Qe (mg g
−1) and
Qt (mg g
−1) are adsorption amounts of the adsorbents at equilibrium and at time
t, respectively;
K1 (min
−1) and
K2 [g (mg min)
−1] are the adsorption rate constants.
As shown in Table S2,† correlation coefficients of the three materials with the pseudo-second order kinetic equation model are larger than those of the pseudo-first order kinetic equation. This suggests that the pseudo-second order kinetic model can better reflect the adsorption of arsenate on these materials.
Effects of anions on arsenic adsorption. There are many different kinds of substances in a natural water system, and especially the presence of various anions may affect adsorption of arsenate on a material. Therefore, we selected several common anions in water to study their effects on the adsorption of arsenic. The PO43−, SO42−, CO32−, NO3−, and Cl− ions were selected as coexisting ion influencing factors and effects of excess anions on the adsorption of arsenate on materials were investigated. The experimental results are shown in Fig. 5. The presence of phosphate can significantly affect removal efficiency of pentavalent arsenic in water. When the concentration of phosphate in water was 1.333 mmol L−1, then removal efficiency of As(V) in water was reduced about 80% on MgAlFe-CLDH, MgAlFe–Cl-LDH, and MgAlFe–NO3-LDH. When the concentration of phosphate in the water increased to 13.33 mmol L−1, then removal efficiency of As(V) decreased about 90%. Those were similar to results reported previously,53 and the reason that phosphate more significantly affects the efficiency of arsenic removal is because it competes for adsorption.54,55 This can be explained by the similar tetrahedral structures of arsenate and phosphate while the presence of nitrate and chloride ions, lacking a tetrahedral structure, have little effect on the removal efficiency of arsenate. This also could be ascribed to the fact that these ions are monovalent and have lower ionic potential, resulting in their weaker competition ability with As(V).56 Carbonate and sulfate have little effect on the adsorption of arsenic by MgAlFe-CLDH, but a higher concentration of carbonate and sulfate can significantly reduce adsorption efficiency of MgAlFe–Cl-LDH and MgAlFe–NO3-LDH to arsenate. When the concentration of carbonate or sulfate in water was 13.33 mmol L−1, then removal efficiency of As(V) was reduced about 60% on MgAlFe–Cl-LDH and MgAlFe–NO3-LDH. High concentrations of carbonate or sulfate can affect the exchange of chloride (or nitrate) ions in the material layers with arsenate in water.
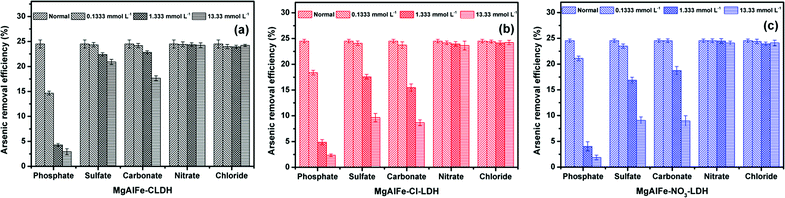 |
| Fig. 5 Effect of coexisting anions on arsenic removal by materials in water. (a) MgAlFe-CLDH. (b) MgAlFe–Cl-LDH. (c) MgAlFe–NO3-LDH. | |
Effect of adsorbent dosage and pH on arsenic adsorption. The effect of the adsorbent dosage on removal efficiency of arsenic was evaluated by gradually increasing the dosage of the material in an experiment. The results of the experiment are shown in Fig. S4.† It can be seen from Fig. S4† that an increasing amount of material increases the removal rate of arsenate. When the MgAlFe–NO3-LDH dosage was 0.4 g L−1, then the removal rate reached 99.9% and the remaining arsenic concentration was below 10 μg L−1. When the dosage of MgAlFe–Cl-LDH reached 1 g L−1, then the removal rate was about 99.8% and the concentration of residual arsenate was also lower than 10 μg L−1. However, when the concentration of MgAlFe-CLDH was 0.1 g L−1, the same effect could be achieved. The above situation meets the World Health Organization's, EPA's, and China's drinking water standards for concentration of arsenic limit requirements. Effects of initial solution pH on arsenate adsorption are shown in Fig. S4b† where it can be seen that the effect of pH on the adsorption of arsenate by MgAlFe-CLDH can be neglected in an initial pH range from 3 to 10. With an increase of pH, the adsorption capacity decreases slowly and may be related to the electrostatic repulsion of arsenate and the surfaces of MgAlFe–Cl-LDH and MgAlFe–NO3-LDH.
Adsorption of fluoride
Isotherms of fluoride adsorption. Relevant data obtained from the isothermal adsorption experiments with fluoride were analyzed and fitted and the obtained fitting curves are shown in Fig. 6a–c. Relevant parameters were also calculated, as shown in Table S3.† It can be seen from Fig. 6 and Table S3† that the data of MgAlFe-CLDH adsorbed fluoride was more consistent with a Freundlich isothermal equation, while the adsorption of fluoride on MgAlFe–Cl-LDH or MgAlFe–NO3-LDH was more consistent with a Sips adsorption isotherm equation. The above results are similar to the adsorption of arsenic which indicates that the mechanism of adsorption of arsenate and fluoride are also similar.
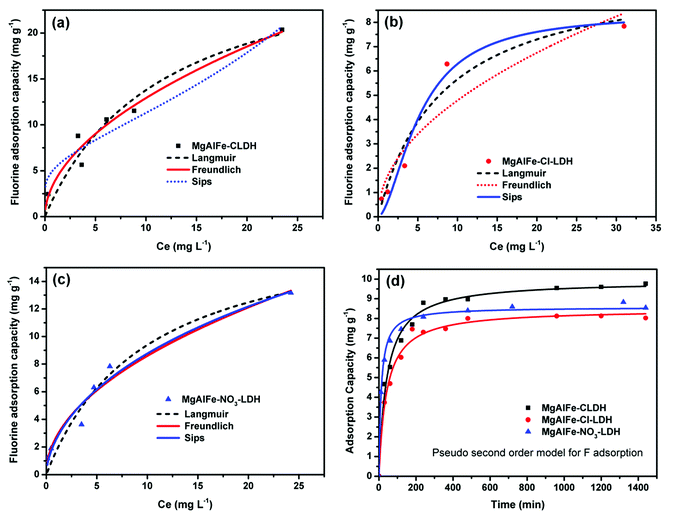 |
| Fig. 6 (a) Isothermal study of adsorption of fluoride with MgAlFe-CLDH. (b) Isothermal study of adsorption of fluoride with MgAlFe–Cl-LDH. (c) Isothermal study of adsorption of fluoride with MgAlFe–NO3-LDH. (d) Kinetic fitting curve of fluoride adsorption on the three materials. | |
Kinetics of fluoride adsorption. The fitting curves of the adsorption kinetics of fluoride on the three materials are shown in Fig. 6d and the obtained fitting parameters are shown in Table S4† where it can be seen that the fluoride adsorption rates on these materials were very fast in the first 3 hours. MgAlFe–NO3-LDH is faster than MgAlFe–Cl-LDH and MgAlFe-CLDH when achieving the adsorption equilibrium of fluoride. This suggests that inter granular nitrate ions are more likely to undergo ion exchange with fluoride ions and have faster exchange rates. In order to further explore the adsorption process, a pseudo-first order kinetic model and pseudo-second order kinetic model were used to fit the relevant data of the fluoride adsorption kinetics experiment. As shown in Table S4,† correlation coefficients of the three materials with the pseudo-second order kinetic equation model are larger than those of the pseudo-first order kinetic equation. This suggests that the pseudo-second order kinetic model better reflects the adsorption of fluoride.
Effects of anions on fluoride adsorption. The PO43−, SO42−, CO32−, NO3−, and Cl− ions were selected as coexisting ion influencing factors. Effects of excess anions on the adsorption of fluoride on the materials were investigated and the experimental results are shown in Fig. 7. The presence of phosphate can significantly affect the removal efficiency of fluoride in water. When the phosphate content in water was 1.333 mmol L−1, the removal efficiency of fluoride in water was reduced about 70% on the MgAlFe-CLDH, MgAlFe–Cl-LDH, and MgAlFe–NO3-LDH. The mechanism of the effect of phosphate on the adsorption of fluoride on materials was similar to the mechanism by which phosphate affects the adsorption of arsenate. Because pK2 of phosphoric acid is 7.21, under the experimental conditions (pH = 6.0), the main form of phosphate is H2PO4−, which can also produce strong competitive adsorption with fluoride ions57 while the presence of nitrate and chloride ions have little effect on the removal efficiency of fluoride. Also, carbonate and sulfate have little effect on the adsorption of fluoride by MgAlFe–NO3-LDH, but a higher concentration of carbonate or sulfate (13.33 mmol L−1) can significantly reduce the adsorption efficiency of MgAlFe–Cl-LDH to fluoride (about 50%). It is noteworthy that the presence of lower concentrations of carbonate or sulfate in water can significantly affect the removal of fluoride by MgAlFe-CLDH. The reason may be that the carbonate or sulfate is structurally reconstituted with CLDH. This process has a significant effect on the adsorption of fluoride ions on the materials.
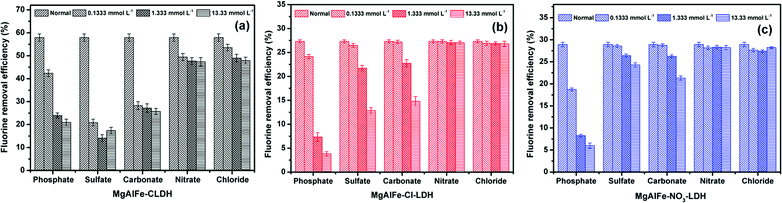 |
| Fig. 7 Effect of coexisting anions on fluoride removal by materials in water. (a) MgAlFe-CLDH; (b) MgAlFe–Cl-LDH; (c) MgAlFe–NO3-LDH. | |
Effect of adsorbent dosage and pH on fluoride adsorption. Effects of dosage of different materials on the removal efficiency of fluoride were evaluated by gradually increasing the dosage of the material in the experiments and results of the experiments are shown in Fig. S5a.† Effects of the initial pH of solution on the adsorption of fluoride on materials were also studied and results are shown in Fig. S5b.† With an increase of material dosage, removal efficiency of fluoride ions in water increases. When the dosages of MgAlFe-CLDH and MgAlFe–NO3-LDH reached 2.0 g L−1, the removal rate of fluoride ions in water reached more than 95%. The fluoride adsorption capacity of the material tends to decrease slowly with increasing pH. This may be related to a weak electrical repulsion between the surfaces of the materials and fluoride ions.
Isothermal study for simultaneous adsorption of arsenic and fluoride. Isothermal experiments of arsenate and fluoride ion coexistent systems were carried out, data fitting curves are shown in Fig. 8–10, and the calculated relevant parameters are shown in Table S5.† It can be seen from Fig. 8, that the equilibrium adsorption capacity (Qe) for arsenate and fluorine on MgAlFe-CLDH in a coexistant (binary) system is lower than that in a single (unitary) system due to the presence of competitive adsorption. It is noteworthy that the adsorption behavior of arsenate and fluoride in a material is consistent with the Freundlich adsorption isothermal equation, whether it is a single (unitary) system or a coexistent (binary) system. Adsorption isotherms of arsenic and fluorine adsorbed on MgAlFe-CLDH are shown in Fig. 9. It can be seen from Fig. 9 the adsorption behavior of arsenate and fluoride on the MgAlFe–Cl-LDH material is consistent with the Sips model, whether it is a unitary or binary system. The equilibrium adsorption capacity (Qe) for arsenate on MgAlFe–Cl-LDH in the binary system is lower than that in the unitary system due to the presence of competitive adsorption. Interestingly, the equilibrium adsorption capacity (Qe) for fluoride on MgAlFe–Cl-LDH in the binary system is higher than that in the unitary system and the maximum adsorption capacity (Qm) of fluoride in the binary system is higher than that of the single system. It is shown that fluoride has an advantage over competitive adsorption in the coexisting system and occupies more adsorbable sites on MgAlFe–Cl-LDH in the adsorption process. The adsorption of arsenate and fluoride on MgAlFe–NO3-LDH isothermal data fitting curves are shown in Fig. 10 which shows that the adsorption behaviors of arsenate and fluoride on the MgAlFe–NO3-LDH material are consistent with the Sips adsorption isothermal equation. The equilibrium adsorption capacity (Qe) for arsenate and fluorine on MgAlFe–NO3-LDH in the binary system was lower than that in the unitary system due to the presence of competitive adsorption. It is noteworthy that the maximum adsorption capacity (Qm) of fluoride in the binary system was higher than that of the single system. Data also showed that fluoride in the coexisting system occupied more adsorbable sites on the material than arsenate.
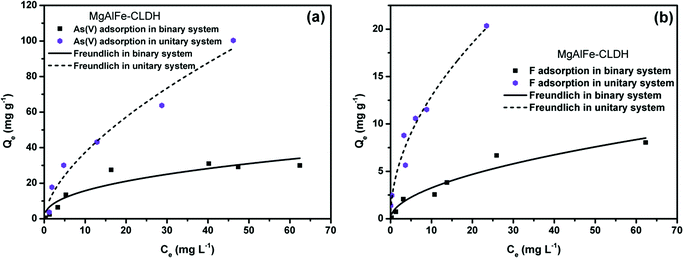 |
| Fig. 8 (a) Isothermal study of adsorption of arsenateon MgAlFe-CLDH in coexistence with fluoride. (b) Isothermal study of adsorption of fluoride with MgAlFe-CLDH in coexistence with arenate. | |
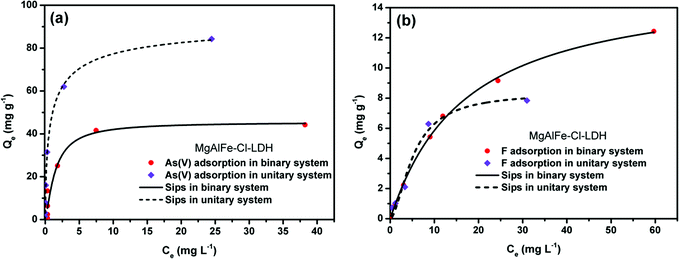 |
| Fig. 9 (a) Isothermal study of adsorption of arsenate on MgAlFe–Cl-LDH in coexistence with fluoride. (b) Isothermal study of adsorption of fluoride with MgAlFe–Cl-LDH in coexistence with arsenate. | |
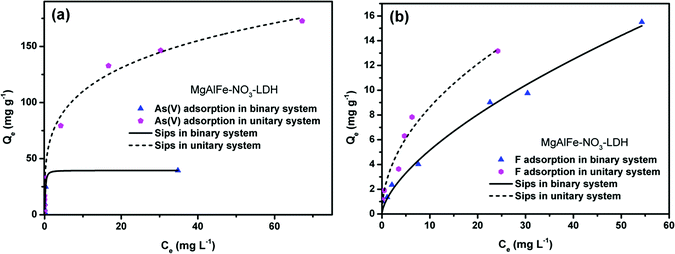 |
| Fig. 10 (a) Isothermal study of adsorption of arsenateon MgAlFe–NO3-LDH in coexistence with fluoride. (b) Isothermal study of adsorption of fluoride with MgAlFe–NO3-LDH in coexistence with arsenate. | |
Simultaneous removal of arsenate and fluoride. The effect of MgAlFe–NO3-LDH dosage on adsorption of the arsenate and fluoride binary system was studied and results are shown in Fig. 11. It was found that a competitive adsorption behaviour between arsenate and fluoride on MgAlFe–NO3-LDH occurred in the experiment within a dosage range of 0.2–1.5 g L−1 when the initial concentrations of arsenate and fluoride were the same 2 mg L−1. The removal rate of fluoride and arsenate increased with an increase in the dosage of MgAlFe–NO3-LDH. When the adsorbent dosage was 1.5 g L−1, the removal rate of fluoride and arsenate were 99.44% and 99.8%, respectively. The remaining concentration of arsenic was less than 10 μg L−1 and the remaining fluoride ion concentration was below 100 μg L−1 which meets the World Health Organization's, EPA's and China's drinking water standards for both arsenic and fluoride concentration limit requirements. It can be found from the image of SEM (Fig. S6a†) that MgAlFe–NO3-LDH after adsorption of arsenate and fluoride remained in a sheet structure. The EDS analysis (Fig. S6b†) showed that composition of arsenic and fluorine existed on the surface of the adsorbent.
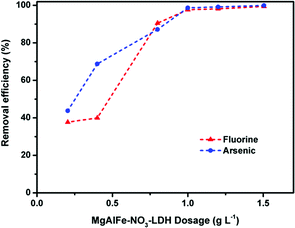 |
| Fig. 11 Effect of MgAlFe–NO3-LDH dosage on the adsorption of As(V) and F− binary system. | |
Desorption. In order to investigate stability and the possibility of adsorbent materials after adsorption of arsenate and fluoride, 100 mg L−1 solutions of NaOH, Na2CO3, and Na2HPO4 were separately used as eluants. The results are listed in Table 2 which shows that when NaOH, Na2CO3, or Na2HPO4 solution are the eluent, some arsenate will be eluted, but the desorption rate is not high. The desorption rate of fluoride ions was higher than that of adsorbed arsenate which indicates that arsenate had a greater affinity for MgAlFe–NO3-LDH than fluoride, and was more difficult to release.
Table 2 Desorption rate of MgAlFe–NO3-LDH adsorbed arsenate and fluoride
Desorption solution |
Desorption rate (%) |
As(V) |
F |
H2O |
0 |
0 |
NaOH |
3.26 |
18.21 |
Na2CO3 |
1.789 |
70.61 |
Na2HPO4 |
25.1 |
59.84 |
Mechanism. The XRD patterns of the three synthesized materials after adsorption of the target ions of arsenate or fluoride are shown in Fig. 12 where it can be seen that MgAlFe-CLDH had obvious lamellar structure characteristic peaks of LDH after the As(V) or F− adsorption. From the SEM photograph of Fig. 13, it was clear that the material had a more lamellar structure after adsorption of arsenic. It was further explained that the adsorption process of CLDH on arsenate and fluoride ions was mainly the process of reconstructing the LDH structure with arsenate or fluoride ion intercalation. The XRD patterns of MgAlFe–Cl-LDH and MgAlFe–NO3-LDH adsorbed arsenic or fluoride are shown in Fig. 12b and c where it can be seen that the lamellar structure of the material did not change significantly. The adsorption process included surface adsorption and interlayer ion exchange.
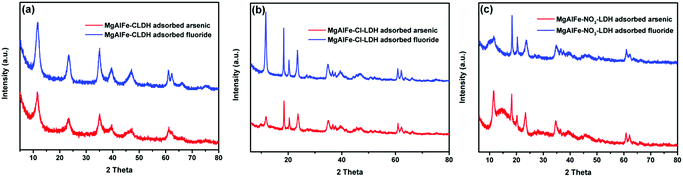 |
| Fig. 12 XRD patterns of materials after adsorption arsenate and fluoride. (a) MgAlFe-CLDH. (b) MgAlFe–Cl-LDH. (c) MgAlFe–NO3-LDH. | |
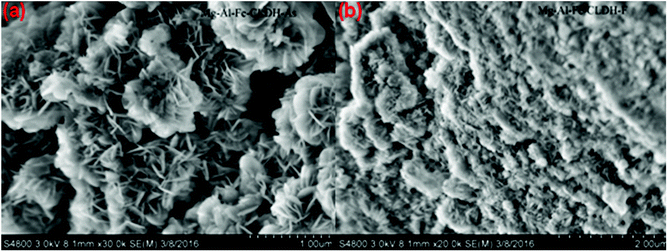 |
| Fig. 13 (a) SEM image of MgAlFe-CLDH after adsorption of arsenate. (b) SEM image of Mg–Al–Fe-CLDH after adsorption of fluoride. | |
Fig. 14a shows the XPS spectra of MgAlFe-CLDH after adsorption. It can be seen from Fig. 14a that fluorine was present in the form of F− due to peaks at 685.4 eV (F–Mg–F) and 684.5 eV (F–Na–F).58,59 It also can be seen from Fig. 14b that magnesium exists in a positive divalent form; the main forms are Mg–O (50.2 eV),60 F–Mg–F (50.9 eV),61 and Mg(OH)2 (49.5 eV).62 Arsenic adsorbed on the surface of MgAlFe-CLDH exists in the form of HAsO42− deduced because of presence of the peak near 45.5 eV.58 From Fig. 15, it shown that the surface features of MgAlFe–Cl-LDH are similar to those of MgAlFe-CLDH after simultaneous adsorption of arsenic and fluoride.
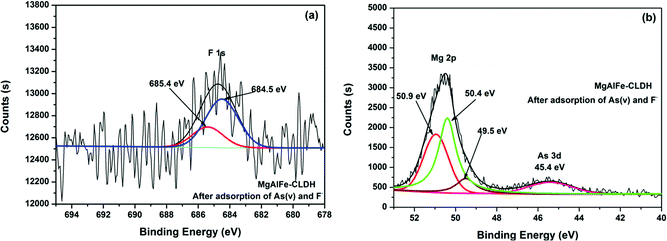 |
| Fig. 14 (a) XPS spectra of MgAlFe-CLDH after adsorption of As(V) and F− peak of F 1s. (b) XPS spectra of MgAlFe-CLDH after adsorption of As(V) and F− peak of Mg 2p and As 3d. | |
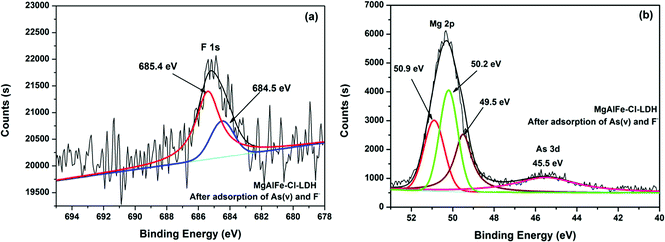 |
| Fig. 15 (a) XPS spectra of MgAlFe–Cl-LDH after adsorption of As(V) and F− peak of F 1s. (b) XPS spectra of MgAlFe–Cl-LDH after adsorption of As(V) and F− peak of Mg 2p and As 3d. | |
Fig. 16 shows that the state of partial fluorine existing on the surface of MgAlFe–NO3-LDH exists in a special form.63 Arsenic adsorbed on MgAlFe–NO3-LDH exists in the form of HAsO42−, but it may also exist in other forms. The peak at 49.4 eV represents a similar form of AsF6−.58,64 This indicated that unlike the former two MgAlFe–Cl-LDH and MgAlFe-CLDH materials, the effect of arsenic and fluorine on the surface of MgAlFe–NO3-LDH may be stronger. The interaction among arsenate, fluoride, and surface composition of the adsorbent is more intense during the simultaneous adsorption on MgAlFe–NO3-LDH which may be the reason why MgAlFe–NO3-LDH is more efficient in simultaneously removing arsenic and fluorine. This also makes arsenic and fluorine adsorbed on the material more stable and not easily desorbed.
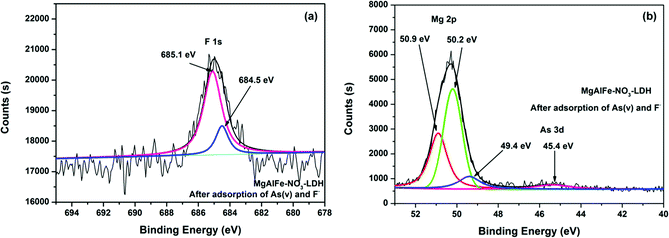 |
| Fig. 16 (a) XPS spectra of MgAlFe–NO3-LDH after adsorption of As(V) and F− peak of F 1s. (b) XPS spectra of MgAlFe–NO3-LDH after adsorption of As(V) and F− peak of Mg 2p and As 3d. | |
Conclusions
Adsorption of arsenate and fluoride on MgAlFe-CLDH was consistent with the Freundlich adsorption isotherm equation both in a single pollutant system and composite pollutant system. However, the adsorption of arsenate and fluoride on MgAlFe–Cl-LDH or MgAlFe–NO3-LDH meet the Sips adsorption isothermal equation. During the simultaneous removal process in a mixed pollutant system, arsenic and fluoride compete for adsorption sites on the material, and the results showed that fluoride is more advantageous in the competition with MgAlFe–Cl-LDH and MgAlFe–NO3-LDH. The interaction between arsenic and fluoride on nitrate-intercalated LDHs was stronger, which may be the reason why nitrate-intercalated LDHs perform better on the efficiency of simultaneous adsorption. MgAlFe–NO3-LDH was used to adsorb arsenate and fluoride in a coexistent system with a concentration of 2 mg L−1 for each pollutant and a dosage of the adsorbent material of 1.5 g L−1. After the adsorption, the remaining concentration of arsenic was less than 10 μg L−1 and the remaining fluoride ion concentration was below 20 μg L−1 which meets the World Health Organization's, EPA's, and China's drinking water standards for both arsenic and fluoride concentration limit requirements.
Conflicts of interest
There are no conflicts to declare.
Acknowledgements
Financial support was obtained from the Swedish Research Council via the contract Dnr. 639-2013-6913, the International Science and Technology Cooperation Program of China (2016YFE0123700) and China Postdoctoral Science Foundation (No. 2017M621529).
References
- A. M. Khair, C. C. Li, Q. H. Hu, X. B. Gao and Y. X. Wanga, Geochem. Int., 2014, 52, 868–881 CrossRef CAS.
- C. Gonzalez-Horta, L. Ballinas-Casarrubias, B. Sanchez-Ramirez, M. C. Ishida, A. Barrera-Hernandez, D. Gutierrez-Torres, O. L. Zacarias, R. J. Saunders, Z. Drobna, M. A. Mendez, G. Garcia-Vargas, D. Loomis, M. Styblo and L. M. Del Razo, Int. J. Environ. Res. Public Health, 2015, 12, 4587–4601 CrossRef CAS PubMed.
- A. Rasool, T. F. Xiao, Z. T. Baig, S. Masood, K. M. G. Mostofa and M. Iqbal, Environ. Sci. Pollut. Res., 2015, 22, 19729–19746 CrossRef CAS PubMed.
- A. Shahab, S. Qi and M. Zaheer, Environ. Sci. Pollut. Res., 2018 DOI:10.1007/s11356-018-2320-8.
- E. Hayat and A. Baba, Environ. Monit. Assess., 2017, 189, 318 CrossRef PubMed.
- M. Kumar, A. Das, N. Das, R. Goswami and U. K. Singh, Chemosphere, 2016, 150, 227–238 CrossRef CAS PubMed.
- M. T. Alarcon-Herrera, J. Bundschuh, B. Nath, H. B. Nicolli, M. Gutierrez, V. M. Reyes-Gomez, D. Nunez, I. R. Martin-Dominguez and O. Sracek, J. Hazard. Mater., 2013, 262, 960–969 CrossRef CAS PubMed.
- M. I. Jimenez-Cordova, L. C. Sanchez-Pena, T. Matousek, E. Villarreal, M. Cardenas-Gonzalez, A. Barrera-Hernandez, M. C. Gonzalez-Horta, O. C. Barbier and L. M. Del Razo, Toxicol. Lett., 2016, 259, S121 CrossRef.
- E. E. Villarreal-Vega, M. I. Jimenez-Cordova, L. C. Sanchez-Pena, J. Narvaez-Morales, A. Barrera-Hernandez, M. Cardenas-Gonzalez, L. M. Del Razo, M. C. Gonzalez-Horta and O. Barbier, Toxicol. Lett., 2016, 259, S133–S134 CrossRef.
- H. Khan, Y. Verma and S. V. S. Rana, Cancer Med., 2018, 7, 33 Search PubMed.
- Y. P. Zhu, S. H. Xi, M. Y. Li, T. T. Ding, N. Liu, F. Y. Cao, Y. Zeng, X. J. Liu, J. W. Tong and S. F. Jiang, NeuroToxicology, 2017, 59, 56–64 CrossRef CAS PubMed.
- S. V. Jadhav, E. Bringas, G. D. Yadav, V. K. Rathod, I. Ortiz and K. V. Marathe, J. Environ. Manage., 2015, 162, 306–325 CrossRef CAS PubMed.
- L. S. Thakur and P. Mondar, J. Environ. Manage., 2017, 190, 102–112 CrossRef CAS PubMed.
- T. C. Prathna, S. K. Sharma and M. Kennedy, Desalin. Water Treat., 2017, 67, 187–195 CrossRef CAS.
- L. Yan, H. W. Tu, T. S. Chan and C. Y. Jing, Chem. Eng. J., 2017, 313, 983–992 CrossRef CAS.
- B. D. Xi, X. W. Wang, W. J. Liu, X. F. Xia, D. S. Li, L. S. He, H. M. Wang, W. J. Sun, T. X. Yang and W. Tao, Sep. Sci. Technol., 2014, 49, 2642–2649 CrossRef CAS.
- R. C. Hott, L. F. O. Maia, M. S. Santos, M. C. Faria, L. C. A. Oliveira, M. C. Pereira, C. A. Bomfeti and J. L. Rodrigues, Environ. Sci. Pollut. Res., 2018, 25, 13857–13867 CrossRef CAS PubMed.
- J. Y. Zhao, H. M. Guo, J. Ma and Z. L. Shen, Int. J. Phytorem., 2015, 17, 355–362 CrossRef CAS PubMed.
- K. D. Brahman, T. G. Kazi, J. A. Baig, H. I. Afridi, S. S. Arain, S. Saraj, M. B. Arain and S. A. Arain, Chemosphere, 2016, 150, 320–328 CrossRef CAS PubMed.
- V. K. Rathore and P. Mondal, Ind. Eng. Chem. Res., 2017, 56, 8081–8094 CrossRef CAS.
- V. K. Rathore, D. K. Dohare and P. Mondal, J. Environ. Chem. Eng., 2016, 4, 2417–2430 CrossRef CAS.
- S. Bibi, A. Farooqi, K. Hussain and N. Haider, J. Cleaner Prod., 2015, 87, 882–896 CrossRef CAS.
- H. M. Guo, L. J. Yang and X. Q. Zhou, Sep. Sci. Technol., 2014, 49, 2412–2425 CrossRef CAS.
- P. P. Huang, C. Y. Cao, F. Wei, Y. B. Sun and W. G. Song, RSC Adv., 2015, 5, 10412–10417 RSC.
- D. J. Kang, X. L. Yu, S. R. Tong, M. F. Ge, J. C. Zuo, C. Y. Cao and W. G. Song, Chem. Eng. J., 2013, 228, 731–740 CrossRef CAS.
- F. Cavani, F. Trifirò and A. Vaccari, Catal. Today, 1991, 11, 173–301 CrossRef CAS.
- K.-H. Goh, T.-T. Lim and Z. Dong, Water Res., 2008, 42, 1343–1368 CrossRef CAS PubMed.
- J. Wang, T. Zhang, M. Li, Y. Yang, P. Lu, P. Ning and Q. Wang, RSC Adv., 2018, 8, 22694–22709 RSC.
- T. Zhang, H. Yu, Y. Zhou, J. Rong, Z. Mei and F. Qiu, Korean J. Chem. Eng., 2016, 33, 720–725 CrossRef CAS.
- S. P. Suriyaraj and R. Selvakumar, RSC Adv., 2016, 6, 10565–10583 RSC.
- M. Pigna, J. J. Dynes, A. Violante, A. Sommella and A. G. Caporale, Environ. Eng. Sci., 2016, 33, 98–104 CrossRef CAS.
- Z. Sun, J.-S. Park, D. Kim, C.-H. Shin, W. Zhang, R. Wang and P. Rao, Water, Air, Soil Pollut., 2017, 228 CAS.
- N. Tian, X. K. Tian, X. W. Liu, Z. X. Zhou, C. Yang, L. L. Ma, C. Tian, Y. Li and Y. X. Wang, Chem. Commun., 2016, 52, 11955–11958 RSC.
- M. T. Rahman, T. Kameda, S. Kumagai and T. Yoshioka, React. Kinet., Mech. Catal., 2017, 120, 703–714 CrossRef CAS.
- L. J. Ma, S. M. Islam, H. Y. Liu, J. Zhao, G. B. Sun, H. F. Li, S. L. Ma and M. G. Kanatzidis, Chem. Mater., 2017, 29, 3274–3284 CrossRef CAS.
- P. Wu, J. S. Wu, L. Xia, Y. Liu, L. Y. Xu and S. X. Song, RSC Adv., 2017, 7, 26104–26112 RSC.
- H. Lu, Z. Zhu, H. Zhang, J. Zhu, Y. Qiu, L. Zhu and S. Küppers, ACS Appl. Mater. Interfaces, 2016, 8, 25343–25352 CrossRef CAS PubMed.
- H. Lu, Z. Zhu, H. Zhang, J. Zhu and Y. Qiu, Chem. Eng. J., 2015, 276, 365–375 CrossRef CAS.
- J. Hong, Z. Zhu, H. Lu and Y. Qiu, Chem. Eng. J., 2014, 252, 267–274 CrossRef CAS.
- J. Yu, Z. Zhu, H. Zhang, Y. Qiu and D. Yin, Environ. Sci. Pollut. Res., 2018, 24, 24293–24304 CrossRef PubMed.
- V. Rives, Layered Double Hydroxides: Present and Future, Nova Science Publishers, Inc., New York, 2001 Search PubMed.
- W. Kagunya, R. Baddour-Hadjean, F. Kooli and W. Jones, Chem. Phys., 1998, 236, 225–234 CrossRef CAS.
- K. S. W. Sing and R. T. Williams, Adsorpt. Sci. Technol., 2004, 22, 773–782 CrossRef CAS.
- Y. Li, Y. Xu, X.-y. Chen, F. Ge and R.-l. Zhu, Appl. Catal., B, 2014, 160, 115–121 Search PubMed.
- I. Langmuir, J. Am. Chem. Soc., 1916, 38, 2221–2295 CrossRef CAS.
- I. Langmuir, J. Am. Chem. Soc., 1917, 39, 1848–1906 CrossRef CAS.
- H. Freundlich, Colloid and Capillary Chemistry, Methuen, London, 1926 Search PubMed.
- R. Sips, J. Chem. Phys., 1948, 16, 490–495 CrossRef CAS.
- S.-H. Lee, M. Tanaka, Y. Takahashi and K.-W. Kim, Chemosphere, 2018, 211, 903–911 CrossRef CAS PubMed.
- H. Lu, S. Liu, H. Zhang, Y. Qiu, J. Zhao and Z. Zhu, Water, 2018, 10, 1150 CrossRef.
- S. V. Prasanna and P. V. Kamath, J. Colloid Interface Sci., 2009, 331, 439–445 CrossRef CAS PubMed.
- Y. S. Ho and G. McKay, Water Res., 2000, 34, 735–742 CrossRef CAS.
- A. Violante, M. Pucci, V. Cozzolino, J. Zhu and M. Pigna, J. Colloid Interface Sci., 2009, 333, 63–70 CrossRef CAS PubMed.
- A. G. Caporale, M. Pigna, J. J. Dynes, V. Cozzolino, J. Zhu and A. Violante, J. Hazard. Mater., 2011, 198, 291–298 CrossRef CAS PubMed.
- F. Kolbe, H. Weiss, P. Morgenstern, R. Wennrich, W. Lorenz, K. Schurk, H. Stanjek and B. Daus, J. Colloid Interface Sci., 2011, 357, 460–465 CrossRef CAS PubMed.
- K. H. Goh and T. T. Lim, J. Hazard. Mater., 2010, 180, 401–408 CrossRef CAS PubMed.
- K. Yang, L.-g. Yan, Y.-m. Yang, S.-j. Yu, R.-r. Shan, H.-q. Yu, B.-c. Zhu and B. Du, Sep. Purif. Technol., 2014, 124, 36–42 CrossRef CAS.
- C. D. Wagner and G. E. Muilenberg, Handbook of X-ray Photoelectron Spectroscopy: A Reference Book of Standard Data for Use in X-ray Photoelectron Spectroscopy, Physical Electronics Division, Perkin-Elmer Corp., Eden Prairie, Minn., 1979 Search PubMed.
- D. Briggs, Handbook of X-ray and Ultraviolet Photoelectron Spectroscopy, Heyden, London, Philadelphia, 1977 Search PubMed.
- R. Jerome, P. Teyssie, J. J. Pireaux and J. J. Verbist, Appl. Surf. Sci., 1986, 27, 93–105 CrossRef CAS.
- C. D. Wagner, Faraday Discuss. Chem. Soc., 1975, 60, 291–300 RSC.
- D. E. Haycock, M. Kasrai, C. J. Nicholls and D. S. Urch, J. Chem. Soc., Dalton Trans., 1978, 1791–1796, 10.1039/DT9780001791.
- T. Birchall, J. A. Connor and L. H. Hillier, J. Chem. Soc., Dalton Trans., 1975, 2003–2006 RSC.
- S. Hoste, D. F. Van De Vondel and G. P. Van Der Kelen, J. Electron Spectrosc. Relat. Phenom., 1979, 17, 191–195 CrossRef CAS.
Footnote |
† Electronic supplementary information (ESI) available: Full-range XPS spectra of materials, effect of dosage on arsenic and fluoride adsorption, effect of pH on arsenic and fluoride adsorption, SEM image and EDS figure of material after adsorption of arsenic and fluoride. See DOI: 10.1039/c8ra05968c |
|
This journal is © The Royal Society of Chemistry 2018 |