DOI:
10.1039/C8RA05902K
(Paper)
RSC Adv., 2018,
8, 30712-30716
Oxidised carbon nanotubes as dual-domain synergetic stabilizers in electroconductive carbon nanotube flexible coatings†
Received
11th July 2018
, Accepted 26th August 2018
First published on 31st August 2018
Abstract
We report that combining oxidised carbon nanotubes (O-CNTs) and pristine CNTs may be the answer for more electroconductive composites. Short (<1 μm) oxidised multi-wall CNTs (O-MWCNTs) acted as an unobvious and excellent conductivity enhancer in MWCNT-based composite thin films. ‘Blending’ O-MWCNTs (1.5 wt%) with 250 μm-long MWCNTs (98.5 wt%), both of well-defined morphology and physicochemistry, led to a 3- and 26-fold increase in specific conductivity as compared to purely MWCNT- or purely O-MWCNT-based thin films, respectively. We explain the enhanced conductivity by the effect of a dual-domain structure of O-MWCNTs. The scale-up method, i.e. screen-printing, opens a route to application in textronics (i.e. electrical and electronic textiles) and hence targets for medicine, civil/military engineering, wellness, etc.
1 Introduction
Carbon nanotubes (CNTs) due to their unique combination of excellent physical properties are often considered as a wonder filler for conductive nanocomposites.1 Nevertheless, some applications still did not come out of academia since there is a significant problem with agglomeration of CNTs (van der Waals intertube attraction, π-stacking).2,3 Poor dispersibility of CNTs results in difficulties with the formation of effective conductive pathways in the matrix and aggravated mechanical properties of the composite systems.4,5 One of the methods which enables efficient dispersion of CNTs and therefore homogeneity of composites is covalent functionalisation, i.e. chemical modification of the CNTs' outer walls with appropriate functional groups.6 This method weakens the inter-tube attraction and provides improvement of affinity to the matrix (and/or solvent). Nevertheless, according to the established CNT conduction mechanisms,7 it is not a straightforward route toward conductive composites. Covalent functionalisation, particularly destructive oxidative treatments, results in the deterioration of the π-electronic system (introduction of vacancies), hampering transport of the electrons in the as-modified CNTs at the molecular level (Fig. 1).8,9 The methods allowing preservation of the π-conjugated system are practically limited to the examples of [2 + 1] cycloaddition reactions and are rather far from generality.10 There are a few reports on CNT doping achieved as a result of chemical modifications, although such an effect is poorly controllable as it strongly depends on the degree of functionalisation (i.e. number of functional groups introduced onto the surface of CNTs), chemical character of the functionalising agent, and the starting material (reproducibility problem).11–13
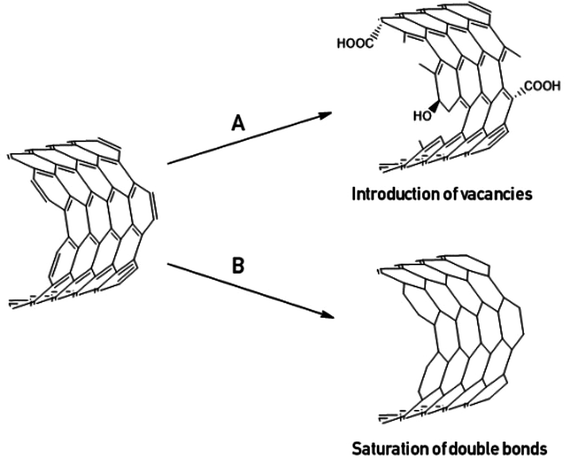 |
| Fig. 1 Possible ways of CNT's sp2-nanoarchitecture deterioration caused by covalent functionalisation: (A) oxidation-induced introduction of structural vacancies; (B) saturation of CNT double bonds being an effect of addition reactions – for clarity an example of CNT hydrogenation is shown. | |
Therefore, it is often proposed to prepare conductive CNT-based nanocomposites using an alternative approach, i.e. non-covalent functionalisation (physical adsorption of appropriate functionalising agents) which does not alter the conductivity of CNTs in such an extensive manner.14,15 However, as common non-ionic surfactants are electrical insulators, they are obvious contaminants of the conductive composites. Moreover, non-covalently functionalised CNTs are very sensitive to the conditions due to the reversible character of physisorption. So, both functionalisation strategies have advantages and disadvantages considering further use of functionalised CNTs in the composites. Here, we would like to demonstrate our findings made while the search for the answer for the title question, that is – whether oxidation (which belongs to family of covalent functionalisations) may be anyhow beneficial in the light of fabrication of the electroconductive composites.
2 Experimental
CNT synthesis and functionalisation
Multi-wall CNTs (MWCNTs) were synthesized via catalytic chemical vapour deposition (c-CVD) method. O-MWCNTs were obtained in a process involving the mixture of concentrated nitric and sulphuric acids (3
:
1 v/v). Detailed information about the synthetic procedures and characterization of the materials can be found in ESI.† MWCNTs and O-MWCNTs were characterized (100 TEM randomly taken measurements) by outer diameter/length equal to 44 ± 12 nm/250 ± 120 μm and 21 ± 11 nm/0.8 ± 0.4 μm, respectively.
Preparation of composite thin films
Grinded (1 min, Bosch MM6003 grinder, 180 W) nanofillers were pre-dispersed (sonication, 15 min) in water (20 mL) and subsequently added to a vigorously stirred acrylic resin suspension (Sico Screen Inks NV, SX 150, resin content 10 wt%) (10.000 g). The resulting viscous liquid was stirred (30 min) and further screen-printed (steel stencil, mesh 120, pressure = 50 kPa) on a poly(ethylene terephthalate) (PET) knitwear substrate (240 g m−2, 368 warp threads per 10 cm, 312 weft threads per 10 cm), in a sequential fashion (printing/drying in oven for 5 min at 120 °C) – 20 repetitions of a printing-drying sequence. The demonstration kit was prepared using a 6-diode LED tape (3528SMD, 3M, 60 diodes per m, 4.8 W m−1), copper wiring and DC Power Supply Unit (TTi P120-H). Detailed description of materials and analytical methods is presented in ESI.†
3 Results and discussion
As the composite materials are associated with large-scale applications, we decided to perform our experiments using MWCNTs. The rationale behind this decision was twofold: (i) unlike their single-walled counterparts (SWCNTs) they are readily available in a multi-tonne scale; and (ii) their electrical behaviour is considered to be generally independent of their chirality.16,17 In order to answer the title question, we started with the preparation of O-MWCNT using a well-known protocol involving treatment of pristine MWCNTs with a mixture of concentrated nitric and sulphuric acid. Oxidation is recognised as super-effective in terms of improved dispersibility of so-treated nanotubes.18,19 The enhanced dispersibility of O-CNTs is observed especially in polar systems due to the introduction of hydrophilic moieties onto the CNTs surface (mainly carboxylic and phenolic). On the other hand, oxidation is known for ruthless deterioration of CNTs' unique structure making it a perfect model for our considerations.19,20 Functionalisation degree, i.e. population of oxygen containing groups was determined both by means of thermogravimetric analysis and modified Boehm titration protocol (ESI†) and it was 4.2 mmol g−1 (both COOH and OH) (Fig. S1, ESI†). Morphology of nanotubes before and after oxidation was analysed by TEM (Fig. 2A and B) and revealed a drastic nanotube shortening after oxidative treatment – MWCNTs were preferentially cut at nodes containing residual catalyst nanoparticles.21,22 Energy Dispersive Spectroscopy (EDX) (Fig. 2C and D) provided further evidences in the physicochemistry of MWCNTs and O-MWCNTs surfaces. And so, EDX analysis of MWCNTs – as performed for randomly selected SEM images of area ca. 72
900 μm2 – revealed only C- and Fe-content of 91.2 and 8.8 wt%. The above contents were assigned from the peaks at 277 eV (Kα) for carbon and 704 (Lα), 6400 (Kα) and 7057 eV (Kβ) for iron, respectively. In turn, for O-MWCNTs one could observe a decay of signals from iron as it could be suspected for Fe@MWCNTs treated with strong and oxidizing acids. Here the EDX spectra shown relative contents of 86.5 and 13.5 wt% for C of O, respectively, with only a residual content of Fe. Once the O-MWCNTs were prepared, we used them as a conductive phase in the first series of composite thin films (acrylic resin matrix). The higher O-MWCNT content was applied, the higher viscosity of pre-composite was observed making impossible to obtain uniform distribution of filler for O-MWCNTs concentrations exceeding 75 wt% (related to the polymer matrix).
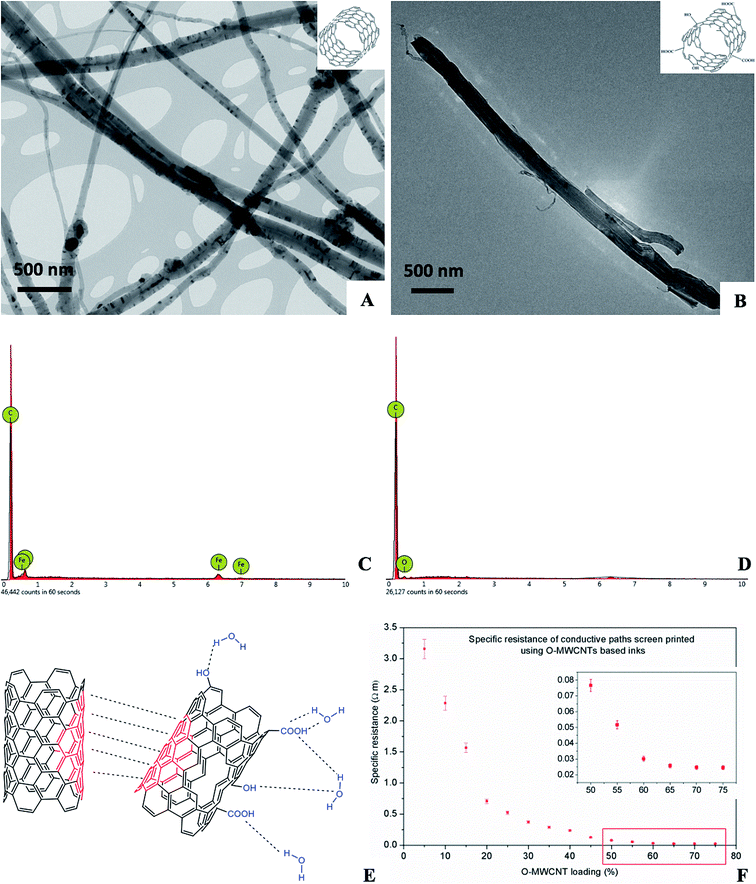 |
| Fig. 2 TEM images of MWCNTs (A) and O-MWCNTs (B) (the insets show schematic structures of nanotubes); EDX spectra of MWCNTs (C) and O-MWCNTs (D); postulated dual-domain character of O-MWCNTs in MWCNT the aqueous inks (E); specific resistance of composite thin films (screen printed on a woven PET support using O-MWCNT based ink) (F); an inset shows the behaviour at higher O-MWCNT loadings. | |
Electrical measurements showed that specific resistance (ρ) of the composites strongly depended on the loading of O-MWCNTs (Fig. 2D). Unfortunately, the values of specific resistance were on average almost 2-fold higher than those of corresponding composites prepared using just pristine MWCNTs. Such observation could be explained by the above-mentioned oxidation-induced disruption of π-electronic network. And although the aqueous O-MWCNT dispersions were months-stable, improved distribution within the matrix did not overcome the decreased conductivity of O-MWCNTs at the molecular level. Moreover, it is obvious that substantial shortening could hinder the formation of effective conductive pathways within the material (increased contact resistance). Importantly, for loadings exceeding 40 wt% of O-MWCNTs thin films were rigid, brittle and poorly adhered to the support.
Even if electrical conductivities of O-MWCNT based composites were lower than those for MWCNTs, they were still significantly higher than those of common non-covalent functionalising ionic agents (e.g. SDS).23 Thus, we hypothesised that due to an O-MWCNTs dual-domain structure (i.e., on one hand, they have hydrophilic oxygen containing groups, and, on the other, vast sp2-philic surfaces), they could act as a highly selective quasi-surfactant for pristine MWCNTs (Fig. 2C). Similar approach was presented by Kuzmicz et al. but the authors employed spherical graphitized nitrogen-doped carbon nanobubbles instead of functionalised CNTs.24 To test our hypothesis, we have prepared a series of composites containing total amount of nanofiller, i.e. both O-MWCNTs and MWCNTs, of 20 wt% with a varying weight ratio (Fig. 3A).
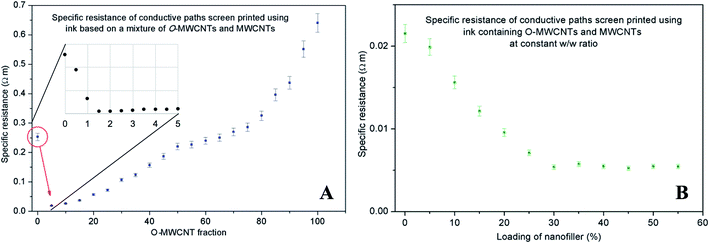 |
| Fig. 3 (A) Specific resistance (ρ) of composites containing 20 wt% of nanofiller at different O-MWCNT/MWCNT ratios; an inset shows the magnified area for the range of lower O-MWCNTs fraction, i.e. from 0–5 wt% with the local minimum; (B) electrical behaviour of thin films as prints at constant O-MWCNT/MWCNT 1.5 : 98.5 w/w ratio. | |
The initial results went far beyond our expectations since the addition of as little as 5 wt% of O-MWCNTs led to ca. 90%-drop in specific resistance of the composite as compared to the one prepared using pristine MWCNTs. Further increase of O-MWCNT/MWCNT ratio led to a gradual increase of the resistance as imperfect O-MWCNTs started to dominate electrical behaviour of the materials. Encouraged by those results, we studied lower (0–8 wt%) weight fractions of O-MWCNTs and we found that the lowest specific resistance could be achieved for the composite containing 1.5 wt% of O-MWCNTs (related to the total conductive phase content) (Fig. 3A, inset). Hence, we prepared a series of composites with an increasing amount of nanofiller of such composition. Fig. 3B represents electrical behaviour of the composites as a function of the so-prepared nanofiller loading. And so, specific resistance dropped slowly with the increasing total loading of nanofiller and, above 30 wt%, remained virtually constant at 5 mΩ m. The loadings exceeding 35 wt%, at the macroscopic scale, resulted in stiff and brittle composite films. It should be emphasized that specific resistance of 5 mΩ m (which equals to the specific conductivity of 2 S cm−1) is much higher than expected if we consider insulating behaviour of the matrix.
There are recent reports on MWCNT-based composite systems on textile supports where specific conductivities exceeded 10 S cm−1 although the matrix was conductive (doped) polypyrrole and the composite fabrication method was much more complex than reported here.25
In order to demonstrate one of the possible applications of the presented methodology, we have built a simple experimental setup consisting of DC power supply connected with crocodile clips to a LED strip light via on-textile printed composite thin film (Fig. S4, ESI†). The sole and tested conductor in the circuit, i.e. apart from the regular cables and ‘crocodile-type’ electrodes, was the MWCNT/O-MWCNT coating. Since PET textile is an insulator the circuit remained open if the ‘crocodile’ electrodes were connected to the white (non-coated) zone of the textile. Microstructure of the non-printed PET textile and the O-MWCNT/MWCNT print at the interphase were presented as SEM images (Fig. S2A–C, ESI†) respectively, showing good adhesion of the coating at low level of the into-fibre migration. Importantly, manipulating (several dozens of bending and stretching, thirty cycles of washing using commercial washing powder at 40 °C and drying at 100 °C) (videos, V1–V3, ESI†) did not affect the electrical performance of the coatings making the proposed methodology promising for applications in the field of smart or e-textiles.
4 Conclusions
The main question of this work was whether employing harsh oxidation as the covalent functionalisation constitutes a reasonable approach when one considers using so-functionalised CNTs in the conductive composites. And, the answer is not unambiguous while being very prospective. On one hand, functionalised CNTs as O-MWCNTs are obviously a way poorer nanofillers than their pristine counterparts in terms of electrical conductivity of composites. On the other, they can find applications in the field but in the rather unconventional role. In fact, the distinct dual-domain molecular structure of functionalised CNTs (f-CNTs) seems to be an interesting alternative to the typical non-covalent approach based on insulating surfactants. We believe that strategies involving f-CNTs as supramolecular functionalising agents can open a new chapter in the CNT-based composites story. Obviously, further studies are needed – starting with the establishment of the enhanced conductivity mechanism. Future work should take under account other, less destructive, functionalisation methods such as cycloaddition reactions or halogenations. It could be also interesting to study other representatives of nanocarbons family such as graphene. There is a plenty of room for improvements. Finally, as we decided to prepare the composites using easily scalable screen-printing method, the presented methodology opens a route to numerous applications including printed electronic circuits, flexible sensors, antennas, e-textiles, ‘lab-on-skin’ while the list is obviously not complete. And although we have clearly indicated that adhesion and physicochemical as well as mechanical compatibility were guaranteed by screen-printing technique, one could easily extend the results of our work for other variants – both from the side of chemistry (e.g. organic solvents or cotton) and methods of the paint application.
Conflicts of interest
There are no conflicts of interest to declare.
Acknowledgements
The authors are greatly indebted to Ministry of Science and Higher Education (grant entitled: ‘Methods of organic and bioorganic chemistry in research on the synthesis and properties of substances and materials for medicine and biotechnology’, 04/990/BK_18/0048) and National Science Centre as well as The National Centre for Research and Development (TANGO, TANGO1/266702/NCBR/2015), all Poland, for financing the research and to MSc Eng. Anna Kolanowska for SEM/EDX analysis.
References
- W. Zhang, A. A. Dehghani-Sanij and R. S. Blackburn, J. Mater. Sci., 2007, 42, 3408–3418 CrossRef.
- C. A. Hunter and J. K. M. Sanders, J. Am. Chem. Soc., 1990, 112, 5525–5534 CrossRef.
- C. R. Martinez and B. L. Iverson, Chem. Sci., 2012, 3, 2191–2201 RSC.
- L. Yu, C. Shearer and J. Shapter, Chem. Rev., 2016, 116, 13413–13453 CrossRef PubMed.
- J. Coleman, et al., Phys. Rev. B: Condens. Matter Mater. Phys., 1998, 58, R7492–R7495 CrossRef.
- N. Karousis, N. Tagmatarchis and D. Tasis, Chem. Rev., 2010, 110, 5366–5397 CrossRef PubMed.
- A. B. Kaiser and V. Skákalová, Chem. Soc. Rev., 2011, 40, 3786–3801 RSC.
- J. C. Charlier, Acc. Chem. Res., 2002, 35, 1063–1069 CrossRef PubMed.
- M. Ohnishi, T. Shiga and J. Shiomi, Phys. Rev. B, 2017, 95, 1–10 CrossRef.
- A. Setaro, et al., Nat. Commun., 2017, 8, 1–7 CrossRef PubMed.
- S. Zhang, et al., RSC Adv., 2018, 8, 12692–12700 RSC.
- D. Janas, A. P. Herman, S. Boncel and K. K. K. Koziol, Carbon, 2014, 73, 225–233 CrossRef.
- D. Janas, S. Boncel, A. A. Marek and K. K. Koziol, Mater. Lett., 2013, 106, 137–140 CrossRef.
- O. M. Burlaka, et al., Biotechnol. Acta, 2015, 8, 71–81 Search PubMed.
- T. Fujigaya and N. Nakashima, Sci. Technol. Adv. Mater., 2015, 16, 24802 CrossRef PubMed.
- A. Bachtold, et al., Phys. Rev. Lett., 2000, 84, 6082–6085 CrossRef PubMed.
- P. R. Wallace, Phys. Rev., 1947, 71, 622–634 CrossRef.
- S. Boncel, K. H. Müller, J. N. Skepper, K. Z. Walczak and K. K. K. Koziol, Biomaterials, 2011, 32, 7677–7686 CrossRef PubMed.
- V. Datsyuk, et al., Carbon, 2008, 46, 833–840 CrossRef.
- R. Gusmão, Z. Sofer, M. Nováček and M. Pumera, ChemElectroChem, 2016, 3, 1713–1719 CrossRef.
- K. J. Ziegler, Z. Gu, H. Peng, E. L. Flor, R. H. Hauge and R. E. Smalley, J. Am. Chem. Soc., 2005, 127, 1541–1547 CrossRef PubMed.
- J. Li and Y. Zhang, Appl. Surf. Sci., 2006, 252, 2944–2948 CrossRef.
- O. Regev, P. N. B. ElKati, J. Loos and C. E. Koning, Adv. Mater., 2004, 16, 248–251 CrossRef.
- D. Kuzmicz, et al., Angew. Chem., Int. Ed., 2014, 53, 1062–1066 CrossRef PubMed.
- R. M. A. P. Lima, J. J. Alcaraz-Espinoza, F. A. G. da Silva and H. P. de Oliveira, ACS Appl. Mater. Interfaces, 2018, 10, 13783–13795 CrossRef PubMed.
Footnote |
† Electronic supplementary information (ESI) available. See DOI: 10.1039/c8ra05902k |
|
This journal is © The Royal Society of Chemistry 2018 |
Click here to see how this site uses Cookies. View our privacy policy here.