DOI:
10.1039/C8RA02632G
(Paper)
RSC Adv., 2018,
8, 20363-20371
Horseradish peroxidase-mediated synthesis of an antioxidant gallic acid-g-chitosan derivative and its preservation application in cherry tomatoes
Received
26th March 2018
, Accepted 23rd May 2018
First published on 4th June 2018
Abstract
Owing to their good solubility and film-forming properties, phenolic acid-g-chitosan derivatives can be used for preservation of fruits and vegetables. However, the chemical synthesis used for the preparation of these derivatives poses a great challenge to food safety. In this study, a method involving horseradish peroxidase catalysis was used to prepare a gallic acid-g-chitosan derivative. The grafting mechanism was studied. Then, the derivative's ability to scavenge free radicals and its preserving application in cherry tomatoes were evaluated. The results indicated that the reaction for horseradish peroxidase catalysis occurred between the amino group of chitosan and the carboxyl group of gallic acid. After enzymatic grafting, the gallic acid-g-chitosan derivative possessed excellent antioxidant abilities in scavenging DPPH, hydroxyl, and superoxide anion radicals. When the derivative was used for the preservation of cherry tomatoes, the results showed that it could effectively protect the ascorbate–glutathione cycle and antioxidant enzyme system of cherry tomatoes and inhibit enzymatic browning. In addition, since this derivative delayed the postharvest senescence of cherry tomatoes, the aroma compounds remain relatively constant throughout the storage period.
Introduction
In the past few decades, the production of fresh fruit and vegetables has grown rapidly worldwide. Fruit and vegetables contain a variety of nutrients including vitamins, minerals, and antioxidants, accounting for a sizeable proportion of people's consumption.1 Among them, cherry tomatoes (Lycopersicon esculentum) are rich in carotenoids and phenolic compounds that are responsible for the high antioxidant ability of this popular fruit.2 However, cherry tomatoes are seasonal fruits, which are highly susceptible to ripening and aging during storage, thereby reducing their nutritional value.3 Consequently, it is crucial to explore strategies to prevent deterioration and extend the shelf life of cherry tomatoes.
Chitosan (CS) is a naturally occurring mucopolysaccharide with low toxicity, biodegradability, biocompatibility, and a variety of bioactivities, such as antioxidant, anticancer, antimicrobial, and enzyme-inhibitory properties.4 As a renewable resource, it has many prospective applications in many areas. However, the strong internal hydrogen bonding of CS endows its poor solubility in water. Moreover, its antioxidant capacity is mainly derived from the chelating ability of N atom on the amino group and the weak electron-donating ability of the amino group and hydroxyl group. Therefore, CS is considered as a secondary antioxidant, limiting its applications in food preservation.5 The modification of CS has become a new approach to develop CS derivatives while improving its solubility and imparting new properties.
Gallic acid (GA) is a natural hydroxybenzoic acid derived from nutgalls, which has a powerful ability to scavenge free radicals and inhibit oxidation chain reaction.6 However, as a sensitive antioxidant molecule, GA has poor stability and is easily degraded under high temperature, light, and other adverse conditions. Some researchers pointed out that grafting of GA onto CS molecules by chemical methods can enhance the antioxidant capacity of CS and the stability of GA. As a result of chemical grafting, the GA–CS film also showed a good preservation effect.5,7 However, the chemical synthesis has many reaction steps and may not be safe. In recent years, biocatalytic enzymes have been used for the modification and production of phenolic acid-g-CS derivatives.8 They are more attractive than chemical processing owing to their strong specificity and environmental friendliness. Besides, enzymes can be utilized continuously when immobilized technology is performed.9 In terms of health and safety, enzymes can also avoid harm from reactive reagents.
Among grafting reactions catalysed by enzymes, laccase, tyrosinase, and peroxidase have been widely used because of their low cost of production, relatively stable nature and a wider variety of substrates than other enzymes.8 These oxidases catalyse phenol into o-quinone intermediate, which can undergo two different types of reaction with CS to form either Schiff-bases (C
N) or Michael type adducts (C–NH) via covalent linkages.10 So far, laccase has been a commonly used oxidase to catalyse the grafting reaction,11–14 while HRP was rarely used. The team of Gimeno et al. synthesized octyl gallate-g-CS by horseradish peroxidase (HRP), showing that the derivative achieved several good properties, such as antioxidant and antimicrobial properties as well as the adhesiveness.15,16 Considering the difference between phenolic esters and acids, it is necessary to study the synthesis of phenolic acid-g-CS derivatives by HRP and evaluate their feasibility in the preservation of fruits and vegetables benefiting from their safety and environmental friendliness.
In our previous studies, we synthesized GA–CS by laccase catalysis and found it could keep the freshness of fruits with an excellent antioxidant activity.13,14 Thus, in this study, we attempted to graft GA onto CS via HRP-catalysed reaction to preserve cherry tomatoes. The characterization using Fourier-transform infrared spectroscopy (FT-IR), ultraviolet-visible spectroscopy (UV-vis), X-ray diffraction (XRD) and nuclear magnetic resonance spectroscopy (NMR) was conducted. Then the antioxidant activities of GA–CS synthesized by HRP on free radicals are studied, and its fresh-keeping effect on cherry tomatoes, especially some biochemical properties and endogenous enzyme activities related to the antioxidant activity of GA–CS was evaluated. Finally, the electronic nose was used to study the change of aroma components of cherry tomatoes during the storage.
Results and discussion
Characterization of HRP-synthesized GA–CS
CS and its grafted product were characterized by UV-vis, FT-IR, NMR and XRD technologies. As shown in Fig. 1a, no significant ultraviolet absorption band was observed in UV-vis spectrum of CS. In contrast, GA exhibited one band at 221 nm and another at 257 nm, being attributable to the carboxy group and π-system of the benzene ring.11 After HRP incubation, the spectral features of the GA–CS were similar to GA, indicating that the chromophores in both were similar. Furthermore, the absorption band of GA–CS was broadened and shifted to 242 and 313 nm. The observed redshifts were attributed to the less amount of energy required for the n–π* and π–π* transition due to the covalent linkage of GA and chitosan.11 These results indicated that GA had been successfully grafted onto CS.
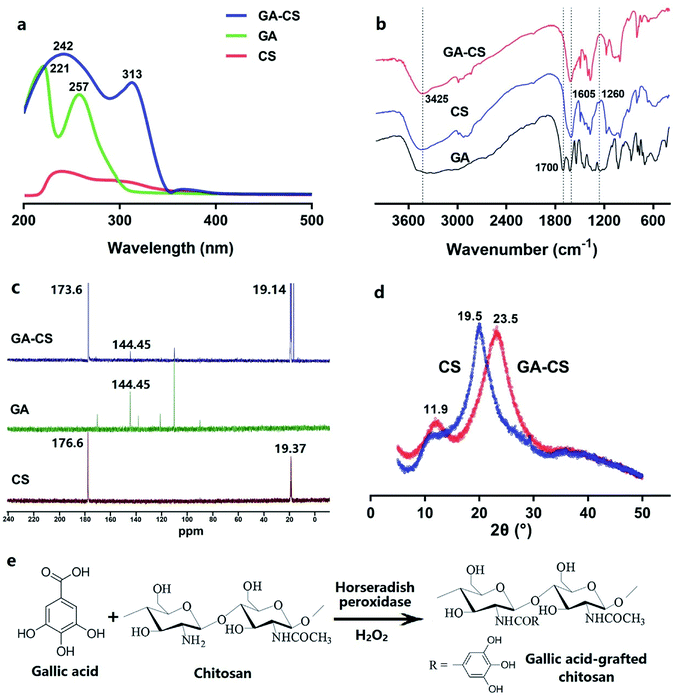 |
| Fig. 1 UV-vis spectra (a), FT-IR spectra (b), 13C NMR spectra (c), and XRD patterns (d) of CS, GA, and GA–CS. Speculated reaction pathway of horseradish peroxidase-mediated synthesis of GA–CS (e). CS represents chitosan, GA represents gallic acid, and GA–CS represents gallic acid–chitosan derivative (GA/CS = 1 : 3, w/w). | |
Further, the FT-IR spectra provided some structural information of CS, GA, and GA–CS. As shown in Fig. 1b, the characteristic peak of aromatic carboxyl group at 1700 cm−1 disappeared from GA after grafting onto CS,17 indicating that the –COOH of GA participated in the HRP-mediated reaction. Considering the reaction mechanism, the groups that could react with –COOH were –OH and –NH2 in CS.8 In Fig. 1b, both native CS and GA–CS exhibited a broadband of 3425 cm−1, which represented a stretching vibration of –OH.11 However, there was no significant difference between the two peaks, suggesting that the hydroxyl group in CS was not the major site of the grafting reaction. Besides, compared to the raw CS, peak of N–H bending vibration (1605 cm−1) in GA–CS was decreased, and the characteristic amide III band (1260 cm−1), corresponding to a complex of C–N stretching and N–H in-plane bending, was disappeared from CS after grafting GA.18 These results illustrated that the amino group in CS reacted with carboxyl group in GA. Liu et al. indicated that the phenoxyl radicals formed by the oxidation of phenol by HRP could be further oxidized into many highly reactive intermediates.19 These intermediates could react with the free amino group of CS to form a covalent bond at pH < 6.3,20 well agreeing with the results of this study.
Solid-state 13C NMR spectrometer was also used to examine the structural changes in CS before and after GA conjugation. The results were shown in Fig. 1c. The peaks at 176.6 ppm (C
O) and 19.37 ppm (–CH3) were observed in CS, corresponding to the carbonyl and methyl groups of N-acetylglucosamine, respectively.21 In the spectrum of GA–CS, besides the carbonyl (173.6 ppm) and methyl groups (19.14 ppm) from CS, a new signal at 144.45 ppm was observed, representing the C
C of gallate group from GA.21 In addition, the increased peak intensity at 173.6 ppm was presumably due to the introduction of C
O between CS and carboxyl groups of GA. These results further confirmed the conjugation of GA on CS chains.
Moreover, the crystallographic structure of CS, GA, and GA–CS were determined by XRD approach. As shown in Fig. 1d, the diffraction pattern of CS showed a characteristic peak at 19.5°, corresponding to crystal form II,22 while GA–CS showed two broader peaks at 23.5° and 11.9°, confirming the successful conjugation of the gallate group onto the CS. Moreover, XRD results revealed that the introduction of GA onto CS caused a decrease in crystallinity of the grafted copolymers. This result suggested that intermolecular hydrogen bindings in GA–CS reduced compared to CS.21 Therefore, the XRD data concluded that the solubility of GA–CS was better than CS, which was consistent with the results from Pasanphan and Chirachanchai.23
Finally, the possible reaction pathway for HRP-catalysed grafting of GA onto CS in the presence of H2O2 was shown in Fig. 1e. In general, GA was oxidized by HRP into intermediates with higher activity, which could be covalently bound to the amino groups of CS to generate GA–CS.
Antioxidant capacity of GA–CS
It has been reported that tomato fruit ripening is closely related to its oxidative status.24 Thus, the antioxidant property of GA–CS, which is important in the postharvest storage of cherry tomatoes, is evaluated by the ability to scavenge the free radicals, including 1,1-diphenyl-2-picrylhydrazyl (DPPH), hydroxyl radicals (OH˙), and superoxide anion radical (O2˙−).
DPPH is a free radical that accepts an electron or hydrogen radical and eventually becomes a stable molecule. As shown in Fig. 2a, CS had a weak inhibitory activity on the DPPH with an EC50 value of 15.2 μg mL−1. Its antioxidant activity might be related to the reaction of free radicals with the hydroxyl group at the C-6 position through H-abstraction, and the reaction of free radicals with the amino group at the C-2 position to form stable macromolecule radicals.25 After grafting GA, CS derivatives had the strongest ability to scavenge DPPH except at the low concentration of 1 μg mL−1 (p < 0.05). The EC50 of GA–CS and GA were 2.76 and 4.45 μg mL−1, respectively. Thus, the DPPH scavenging capacity of GA–CS was improved by introducing GA into CS and seemed totally dependent on the amount of GA introduced.
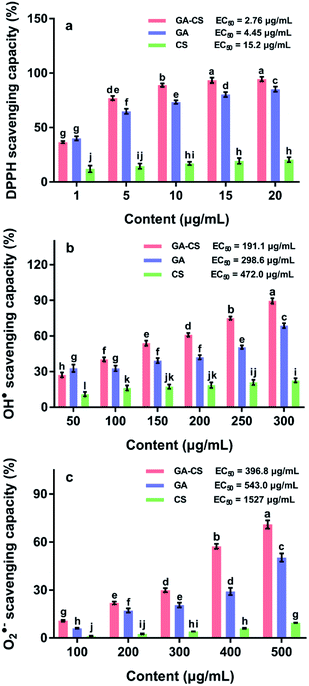 |
| Fig. 2 DPPH (a), OH˙ (b), and O2˙− (c) scavenging capacity of CS, GA and GA–CS. CS represents chitosan, GA represents gallic acid, and GA–CS represents gallic acid–chitosan derivative (GA/CS = 1 : 3, w/w). The same letters indicate no significant difference (p > 0.05). | |
Approaching the next step, the OH˙ scavenging capacity of GA–CS, GA and CS was examined via the Fenton reaction. As shown in Fig. 2b, all test samples scavenged OH˙ in a dose-dependent manner. The EC50 values of GA–CS, GA and CS for were 191.1, 298.6, and 472.0 μg mL−1, respectively. This result indicated that the CS showed the lowest antioxidant potential against OH˙ whereas GA–CS was the strongest. This antioxidant activity appeared to be dependent on the phenolic hydroxyl groups in GA, which was the main active group capable of scavenging OH˙.26
Further, antioxidant efficiency of GA–CS was also evaluated by O2˙− scavenging assay. As shown in Fig. 2c, CS showed only a radical scavenging rate of 9.5% at 500 μg mL−1, whereas the scavenging capacity of GA–CS continued to increase, eventually reaching 71%. The ability of GA to scavenge O2˙− was also increased with an increasing concentration, and the maximum rate could be observed at 50.3%. Compared their EC50, GA–CS showed a minimum, corresponding to the strongest scavenging ability. This result was similar to the result in Fig. 2a and b. However, the O2˙− scavenging efficiency of GA–CS was not as high as DPPH and OH˙, which might be due to different scavenging mechanisms of different radicals.
Effects of GA–CS on ascorbic acid–glutathione cycle of cherry tomato
As discussed above, introduction of GA into CS could improve its antioxidant capacity. Then, during the post-harvest storage period, some physiological indicators of cherry tomatoes closely related to the antioxidant property of GA–CS were further evaluated.
Commonly, ascorbic acid (ASA) and glutathione (GSH) constitute the ASA–GSH cycle, which is an important part of the non-enzymatic antioxidant systems in plants.27 The ASA–GSH cycle is responsible for the removal of H2O2, which can stimulate the increase of enzyme activity in the cycle and increase the scavenging activity of reactive oxygen species (ROS) under moderate stress conditions.27 However, ASA and GSH are usually oxidized and degraded with the ripening of postharvest fruits. As shown in Fig. 3a, ASA level of all cherry tomatoes continued to decline throughout storage. After 10 days, GA–CS treatment showed a higher VC level (14.72 mg/100 g) than other treatments. The above result indicated that HRP-mediated GA–CS derivative could effectively inhibit the oxidation of ASA in cherry tomatoes.
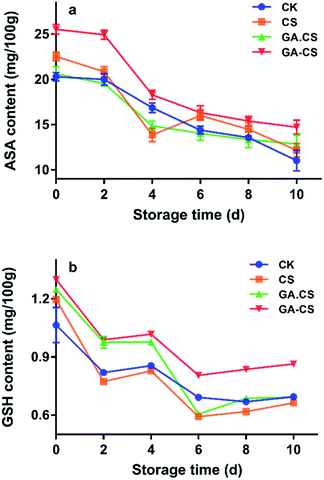 |
| Fig. 3 Changes in ASA (a) and GSH (b) of cherry tomatoes during storage. CS represents chitosan, GA represents gallic acid, GA·CS represents the simple mixing of GA and CS (GA/CS = 1 : 3, w/w), and GA–CS represents gallic acid–chitosan derivative (GA/CS = 1 : 3, w/w). ASA is ascorbic acid, GSH is reduced glutathione. | |
Additionally, the GSH content decreased at the early stage of storage, then increased slightly (Fig. 3b). This might be because cherry tomatoes consumed ASA during storage, resulting in a decrease in GSH content in the ASA–GSH cycle. In the late stage of storage, glutathione reductase reduced oxidized glutathione (GSSH) to GSH due to increased oxidative stress caused by senescence.28 After GA–CS treatment of cherry tomatoes, the GSH content was up to 0.864 mg/100 g after storage for 10 days, which was significantly higher than that of the control group (p < 0.05). This might be because GA–CS had a good antioxidant capacity, which in turn maintain the high activity of related enzymes to protect GSH in cherry tomatoes.
In summary, GA–CS prepared in this study alleviated the degradation of ASA and GSH in cherry tomatoes during storage, which to a certain degree ensured that the ASA–GSH cycle proceeded effectively.
Effects of GA–CS on enzymatic browning of cherry tomato
Like ASA, polyphenols are common antioxidants in cherry tomatoes. They not only bring the enjoyment of colour and taste to humans but also bring health benefits.29 Phenolics in postharvest cherry tomatoes are highly unstable and undergo a various change that deeply affects their taste and nutritional quality. For instance, postharvest handling and transportation induce the subcellular decompartmentalization that stimulates the leakage of phenolic substances from vacuoles, allowing them to contact with polyphenols oxidase (PPO) and peroxidase (POD).30 Then, polyphenols are degraded and oxidized to quinines, followed by brown pigments.
Fig. 4a showed the change in polyphenols in cherry tomatoes during 10 days under different treatments. During the whole storage period, the polyphenols showed a slowly decreasing trend, and the GA–CS group experienced the slowest decline, indicating that GA–CS could inhibit the oxidation of polyphenols. This result might be due to the high ASA content in cherry tomatoes that were treated by GA–CS, and its excellent reducibility prevented the degradation of phenolic compounds.31 Furthermore, PPO activity was consistently lower in the GA–CS treated cherry tomatoes than in the other three groups (Fig. 4b). After 10 days, a significant difference in PPO activity was observed between GA–CS group (0.0668 U g−1 min−1) and other three groups (p < 0.05). The result showed that combination of CS and GA delayed the increase of PPO activity and inhibited enzymatic browning of cherry tomatoes during storage. The antioxidant GA protected the polyphenols in cherry tomatoes and the film-forming property of CS provided an oxygen barrier function.32
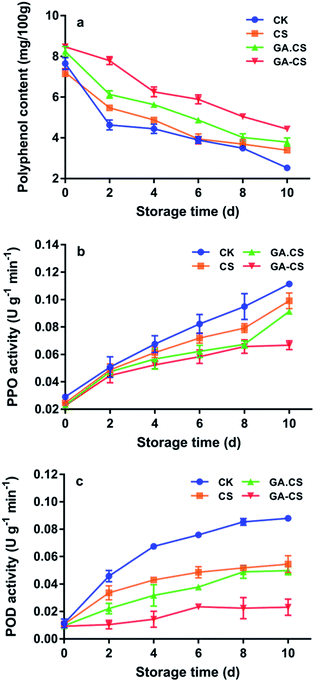 |
| Fig. 4 Polyphenol content (a), PPO activity (b), and POD activity (c) of cherry tomatoes during storage. CS represents chitosan, GA represents gallic acid, GA·CS represents the simple mixing of GA and CS (GA/CS = 1 : 3, w/w), and GA–CS represents gallic acid–chitosan derivative (GA/CS = 1 : 3, w/w). PPO is polyphenol oxidase, POD is peroxidase. | |
Just as PPO, peroxidase (POD) is also involved in browning reactions and producing melanin compounds. It catalyses the decomposition of H2O2 in the presence of a hydrogen donor, such as hydroxycinnamic derivatives and flavans.33 As shown in Fig. 4c, POD activity of GA–CS group showed almost unchanged from the initial state within the first 4 days and reached 0.0231 U g−1 min−1 on the 10th day, while the CK group reached 0.0458 U g−1 min−1 on the second day. This result indicated that GA–CS effectively inhibit the PPO activity. In summary, the antioxidant GA–CS was able to maintain the phenolic content and control the enzymatic browning of cherry tomatoes after harvesting.
Effects of GA–CS on antioxidant enzymes of cherry tomato
As discussed above, owing to its excellent antioxidant capacity, GA–CS synthesized by HRP can protect the ASA–GSH cycle and inhibit enzymatic browning of cherry tomatoes during storage. Then, the effects of GA–CS treatment on activities of some antioxidant enzymes of cherry tomatoes were studied.
In addition to non-enzymatic antioxidants, there are also some enzymatic antioxidants in plants, such as superoxide dismutase (SOD) and catalase (CAT).34 These antioxidant enzymes can remove ROS before their participation in oxidation reactions and alleviate the gradual increase of oxidative stress caused by senescence of fruits and vegetables.35 Generally, in plant cells, the first line of defence was SOD, which converted O2˙− to H2O2. As shown in Fig. 5a, the SOD activity in the control group on the second and tenth days was 3.03 and 16.79 U mL−1 min−1, respectively. The decrease of SOD activity in the later period of storage indicated the ability to scavenge ROS was weakened. However, after 10 days, the SOD activities of the CS, GA·CS, and GA–CS groups were 5.34, 5.74, and 6.42 U mL−1 min−1 respectively, indicating that these treatments could effectively protect the activity of SOD.
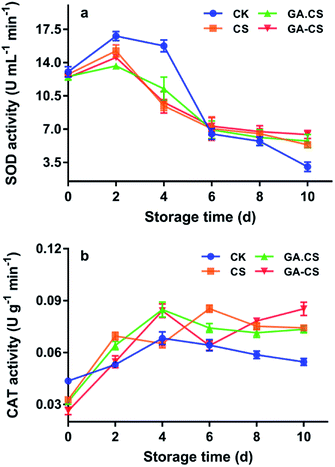 |
| Fig. 5 SOD (a) and CAT (b) activities of cherry tomatoes during storage. CS represents chitosan, GA represents gallic acid, GA·CS represents the simple mixing of GA and CS (GA/CS = 1 : 3, w/w), and GA–CS represents gallic acid–chitosan derivative (GA/CS = 1 : 3, w/w). SOD is superoxide dismutase, CAT is catalase. | |
Moreover, when H2O2 is overproduced from O2˙−, it is further decomposed by CAT, which converts H2O2 to water. As shown in Fig. 5b, similar to SOD, the CAT activity in the control group also increased first and then decreased. The maximum value was 0.0683 U mL−1 min−1 on the fourth day, showing that CAT activity was activated by oxidative stress early in senescence. By the tenth day, CAT activity of cherry tomatoes was minimized to 0.0546 U mL−1 min−1. However, the change in CAT activity of cherry tomatoes coated by GA–CS during storage fluctuated, and a maximum of 0.0853 U mL−1 min−1 was retained. The above result showed that GA–CS could protect the antioxidant enzyme system, enhance the ability to remove ROS, improve anti-aging ability, and extend cherry tomatoes' shelf life.
Effects of GA–CS on aroma compounds of cherry tomato
In general, every fruit has its own characteristic aroma because of the presence of unique monoterpenes, esters, organic acids, aldehydes, ketones. With the advancing ripening, production of volatile components usually increases.36 Thus, aroma changes of different coated cherry tomatoes were assessed by principal component analysis during storage.
As shown in Fig. 6, although the trend was not an irregular distribution along the first and second principal components, the fruity substances at different storage time were concentrated. They had no overlapping area and were easily distinguishable from each other, which was consistent with another study using electronic nose to evaluate the shelf life of tomato storage.37 Besides, the first principal components (axis-x) of CK, CS, GA·CS and GA–CS treatment were 93.75%, 94.71%, 95.47%, and 98.20%, respectively. The second principal component (axis-y) was 5.87%, 4.65%, 4.35%, and 1.41%, respectively. Therefore, the first principal component contributed a lot to the overall aroma, while the contribution of the second principal component was negligible.
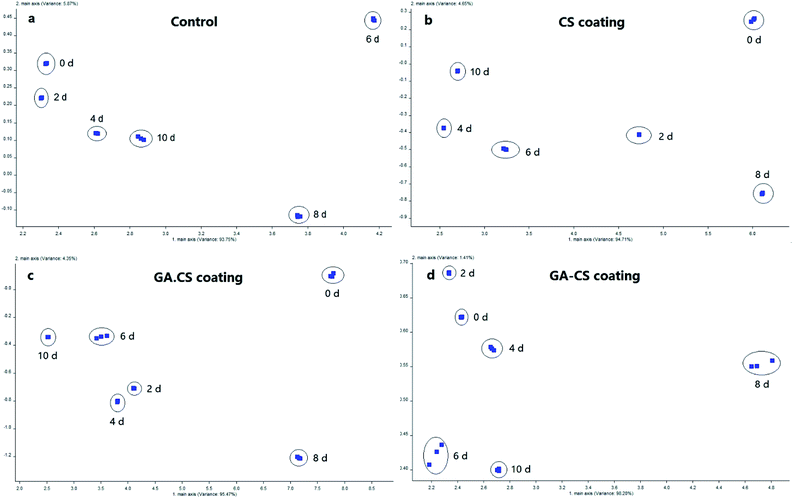 |
| Fig. 6 Electronic nose data – principal component analysis results of cherry tomatoes treated with control (a), CS (b), GA·CS (c), GA–CS (d). GA·CS represents the simple mixing of GA and CS (GA/CS = 1 : 3, w/w), and GA–CS represents gallic acid–chitosan derivative (GA/CS = 1 : 3, w/w). | |
As shown in Fig. 6a, the aroma of the control group was slightly different in the first four days. From the 6th day to the 10th day, the aroma was significantly different, indicating that the physiological activity of the control group during the later period of storage was active, and the aroma changed more. As shown in Fig. 6b and c, the response distance of CS and GA·CS group during storage was large, showing that the aroma components of the cherry tomatoes varied significantly after the two treatments. As shown in Fig. 6d, in addition to the eighth day, the first principal component of GA–CS group changed little during the entire storage period, and the second principal component changed greatly. However, since the second principal component could be neglected in the overall characteristic aroma, this result also showed that the aroma components in cherry tomatoes treated by GA–CS were stable, reflecting the good fresh-keeping effect of GA–CS on cherry tomatoes. Therefore, compared with other treatments, GA–CS can maintain the stability of cherry tomato aroma, well agreeing with the results of other physical and chemical indicators.
Experimental
Materials
CS (Mw 500 kDa) with a deacetylation degree of 90.8% was purchased from Qingdao YunZhou Biotechnology Co., Ltd., China. Before use, CS dissolved in a 2% (w/v) acetic acid was purified by precipitation using NaOH. The precipitate was washed several times with a great amount of deionized water until the pH reached 7.0. GA was obtained from Tianjin Kermel Chemical Reagent Co., Ltd. (Tianjin, China). HRP (EC1.11.1.17, 206 U mg−1) was purchased from Beijing Solarbio Science & Technology Co., Ltd. (Beijing, China). Fresh cherry tomatoes (Lycopersicon esculentum) were purchased from a local supermarket (Qingdao, China). All other reagents were of grade and were used as received without further purification.
Preparation of GA–CS
CS solution (1%, w/v) was prepared by agitating CS flakes in an acetic acid/sodium acetate buffer solution (pH 4.5) at ambient temperature overnight. Then GA was added to CS solution with a GA/CS ratio of (1
:
3, w/w). After homogeneous distribution, HRP (4 U mg−1) was slowly added whilst stirring, 1 mL of 30% H2O2 was added to the reaction solution, reacting for 5 h at 25 °C. The unreacted ingredients and the by-products were removed by dialyzing with 3500 molecular weight cut-off dialysis tubing against distilled water for 72 h. The precipitate obtained was centrifuged and freeze-dried to obtain the GA–CS.
UV-vis, FT-IR, NMR, and XRD analysis
UV-vis spectra of GA–CS sample were recorded using a UV-vis 2450 spectrophotometer (Shimadzu Corporation, Japan) in a range of 200–500 nm. FT-IR spectra were recorded on a Nicolet Nexus 670 infrared spectrophotometer (Thermo Fisher Scientific Inc., USA) with 32 scans at a resolution of 2 cm−1 in a frequency range of 4000–400 cm−1. Solid state 13C NMR test was performed on a Bruker UltraShield 600 PLUS NMR spectrometer (Bruker, Germany). Freeze-dried hydrogels were used and operated at a recording frequency of 100.63 MHz and spinning rate of 8 kHz. XRD patterns were obtained with X-ray diffractometer (Bruker AXS, Germany) using Cu as Kα radiation, and the Bragg's angle 2θ was scanned from 5 to 50° at the rate of 0.1° min−1.
Determination of antioxidant activity
The DPPH scavenging capacity of the GA–CS was quantified according to the method of Mishra et al.38 Briefly, the derivatives with different concentrations were mixed with and without DPPH solution (0.1 mM), then the mixtures were incubated at 25 °C for 30 min. The DPPH scavenging capacity of the coating solutions absorbance of the mixtures was determined at 517 nm. Scavenging effect of GA–CS was calculated by the following equation,
where A0 is the absorbance of the control, As is the absorbance of the sample, and Ai is the absorbance of the sample under identical condition as As with ethanol instead of DPPH solution.
The OH˙ scavenging assay was performed based on Smirnoff and Cumbes.39 1 mL of FeSO4 (6 mM), sample solutions with different concentration, 1 mL of H2O2 (6 mM) and 1 mL of salicylic acid solution was mixed and incubated at 37 °C for 30 min. The scavenging activity of OH˙ was evaluated by the following equation,
where
A0 was the absorbance of the distilled water at 510 nm,
Ai was the absorbance of the distilled water replaced by salicylic acid, and
As was the absorbance of the sample.
The O2˙− scavenging capacity of samples were examined by a pyrogallol autoxidation system with minor modification.40 Briefly, 3 mL of Tris–HCl buffer solution (50 mM, pH 8.2) was incubated in a water bath at 25 °C for 20 min, and then reacted with 10 μL of pyrogallol solution (3 mM) for 3 min, at last 50 μL ascorbic acid was added. The absorbance at 420 nm (A0) was measured 30 s later, indicating the speed of pyrogallol autoxidation. The A1 autoxidation speed was obtained using the above method with the addition of test compounds (0.2 mL) into the Tris–HCl buffer. A blank control of reagent was obtained as A2. The scavenging activity of O2˙− was evaluated by the following equation,
Pretreatment of cherry tomatoes
Cherry tomatoes were washed and randomly divided into 4 groups, and consequently soaked into different coating solutions for 2 min, including 10 mg mL−1 of CS dissolved in acetic acid solution, 250 mg GA and 750 mg CS dissolved in 100 mL acetic acid solution (GA·CS), and 10 mg mL−1 GA–CS dissolved in deionized water. After draining, the cherry tomatoes were then packed in PE plastic bag and stored at 15 °C (the temperature used in display cabinets of fresh fruits and vegetables in a supermarket) for determination of ASA, GSH, polyphenol, and some endogenous enzyme analysis. These parameters were measured every two days.
ASA content
The content of ASA was determined using the 2, 6-dichloroindophenol method.41 10 g of cherry tomato pulp was vortex mixed with 100 mL oxalic acid (20 g L−1). The mixture was then centrifuged at 8000g for 10 min to obtain the supernatant. 10 mL of the supernatant was titrated with 2,6-dichlorophenolindophenol to a reddish colour. The titration volume was recorded when the colour was stable for at least 30 s. Oxalic acid solution (20 g L−1) was used as the blank control with titration performed as described above. The ASA content (mg/100 g) was calculated as follows:
where V1 was the titration volume for the sample (mL), V0 was the titration volume for the blank control (mL), V was the total supernatant volume (mL), c was the ASA content titrated by 1 mL of 2,6-dichlorophenol, Vs was the volume of the sample (mL), and m was the sample mass (g).
Polyphenol content
5 g of cherry tomatoes was homogenized and mixed with 20 mL of 60% (v/v) ethanol. After extraction for 2 h and filtration, the volume was set to 500 mL. The content of polyphenol was determined by the Folin–Ciocalteu method with some modification.42 The reaction mixture was composed of 1 mL of cherry tomatoes extracts, 1 mL of Folin–Ciocalteu reagent, and 3 mL of 20% (w/w) NaCO3 solution. After incubation for 2 h at 25 °C in darkness, the absorbance of samples was measured at 760 nm. The polyphenol content was expressed in terms of GA equivalents based on a standard curve.
GSH content
The content of GSH was measured based on 2-vinylpyridine method.43 In detail, the reaction was initiated by adding 1 unit of glutathione reductase and monitored the increase in absorbance at 412 nm. For measuring oxidized glutathione (GSSG), 20 μL of the extract was mixed with 200 μL of phosphate buffer (0.5 M, pH = 7.5) and 4 μL of 2-vinylpyridine, and the mixture was incubated at 25 °C for 30 min to remove GSH by derivatization. GSSG was assayed by the same way as for total GSH previously. GSH content was determined by subtracting the value for GSSG from the total GSH content.
Endogenous enzyme activity
For determination of endogenous enzyme activity in cherry tomatoes, samples (0.1 g) were ground in a chilled mortar with 1% (w/v) polyvinylpolypyrrolidone and then homogenized with 1.2 mL of 50 mM potassium phosphate buffer (PB) (pH 7.8) containing 1 mM EDTA-Na2 and 0.3% (w/v) Triton X-100. Each homogenate was centrifuged at 13
000g for 20 min at 4 °C and the supernatant was used for the following assays. Activities of peroxidase (POD), catalase (CAT) and superoxide dismutase (SOD) were all measured according to Wang et al.44 Activity of polyphenol oxidase (PPO) was measured based on Chisari et al.45
Analysis of aroma compounds
The aroma ingredients were analyzed by a PEN3 portable E-nose instrument (Win Muster Airsense Analytics Inc., Germany). According to methods previously reported,46 each sample was weighed 40.0 g in a sample vial before being sealed. After equilibrating for 90 min at 25 °C, the hermetic vial was pierced by a Luer lock needle connected to a 3 mm Teflon tubing by using headspace air intake. The flow rate of sample introduction and interior was 200 mL min−1. The preparation time was 5 s before E-nose measurement began, and the measurement process lasted for 60 s, during which the absorbed gases were measured each second. The E-nose system was automatically zero cleared for 60 s and then standardized for 300 s. Afterward, the next headspace sampling started repetitively until all samples were examined. Each sample was measured for five repeats. The relatively stable signal at 55–57 s was extracted as feature data for subsequent principal component analysis.
Statistical analysis
Data were expressed as the mean ± standard deviation of three separate experiments. All statistical analysis was conducted using SPSS 19.0 software (SPSS Inc., USA). The Shapiro–Wilk test was used to assess the normality of data and the Levene test was used to check the homoscedasticity. The difference between the heating treatments was evaluated using the one-way analysis of variance (ANOVA) and Duncan's multiple comparisons.47
Conclusions
In this study, the HRP-catalysed method was used to prepare GA–CS. The reaction mechanism was explored by UV-vis, FT-IR, NMR, and XRD technologies. Then, antioxidant abilities of GA–CS were studied, and fresh-keeping effects of GA–CS on cherry tomatoes were evaluated. The results showed that the formation of GA–CS might begin with the highly active intermediates derived from GA oxidized by HRP. Then, covalent bond was formed between amino groups of CS and carboxyl group of GA. After enzymatic grafting, GA–CS showed the excellent antioxidant capacity and could scavenge DPPH, OH˙, and O2˙−. When applying in the preservation of cherry tomato, GA–CS could effectively protect the ASA–GSH cycle, maintain the activity of intracellular antioxidant enzymes, and inhibit the enzymatic browning. In addition, the aroma components of cherry tomatoes were relatively constant throughout the storage period because the postharvest aging of cherry tomatoes was delayed.
Conflicts of interest
There are no conflicts to declare.
Acknowledgements
This study was funded by National Natural Science Foundation of China (31401549), Special Funds for Taishan Scholars Project of Shandong Province, Shandong Modern Vegetable Industrial Technology System (SDAIT-05-21), Agriculture Scientific and Technological Innovation Project of Shandong Academy of Agriculture Sciences (CXGC2016B10) and Talents of High Level Scientific Research Foundation of Qingdao Agriculture University (631207).
References
- A. Septembre-Malaterre, F. Remize and P. Poucheret, Food Res. Int., 2018, 104, 86–99 CrossRef PubMed.
- O. Masetti, A. Ciampa, L. Nisini, P. Sequi and M. T. Dell'Abate, Food Res. Int., 2017, 100, 623–630 CrossRef PubMed.
- S. Wu, M. Lu and S. Wang, Food Chem., 2016, 199, 296–300 CrossRef PubMed.
- W. Xia, P. Liu, J. Zhang and J. Chen, Food Hydrocolloids, 2011, 25, 170–179 CrossRef.
- B. Schreiber, J. J. Bozell, D. G. Hayes and S. Zivanovic, Food Hydrocolloids, 2013, 33, 207–214 CrossRef.
- S. Roidoung, K. D. Dolan and M. Siddiq, Food Chem., 2016, 210, 422–427 CrossRef PubMed.
- P. Guo, J. D. Anderson, J. J. Bozell and S. Zivanovic, Carbohydr. Polym., 2016, 140, 171–180 CrossRef PubMed.
- J. Liu, H. Pu, S. Liu, J. Kan and C. Jin, Carbohydr. Polym., 2017, 174, 999–1017 CrossRef PubMed.
- O. Vittorio, M. Cojoc, M. Curcio, U. G. Spizzirri, S. Hampel, F. P. Nicoletta, F. Iemma, A. Dubrovska, M. Kavallaris and G. Cirillo, Macromol. Chem. Phys., 2016, 217, 1488–1492 CrossRef.
- F. Hollmann and I. W. Arends, Polymers, 2012, 4, 759–793 CrossRef.
- M. Božič, S. Gorgieva and V. Kokol, Carbohydr. Polym., 2012, 87, 2388–2398 CrossRef.
- A. Aljawish, I. Chevalot, J. Jasniewski, C. Paris, J. Scher and L. Muniglia, Food Chem., 2014, 145, 1046–1054 CrossRef PubMed.
- H. Wu, Y. Wang, T. Zhen, D. Luo, X. Zhang, S. Yang and C. Wang, Food Sci., 2017, 38, 227–232 Search PubMed.
- H. Wu, T. Zhen, C. Chen, Y. Wang, D. Luo, X. Zhang and C. Wang, Transations of the Chinese Society of Agricultural Engineering, 2017, 33, 285–292 Search PubMed.
- L. Itzincab-Mejía, A. López-Luna, M. Gimeno, K. Shirai and E. Bárzana, Int. J. Food Sci. Technol., 2013, 48, 2034–2041 Search PubMed.
- L. Zavaleta-Avejar, E. Bosquez-Molina, M. Gimeno, J. P. Pérez-Orozco and K. Shirai, Food Hydrocolloids, 2014, 39, 113–119 CrossRef.
- W. Pasanphan, G. R. Buettner and S. Chirachanchai, J. Appl. Polym. Sci., 2008, 109, 38–46 CrossRef.
- A. Sionkowska, B. Kaczmarek, K. Lewandowska, S. Grabska, M. Pokrywczyńska, T. Kloskowski and T. Drewa, Int. J. Biol. Macromol., 2016, 89, 442–448 CrossRef PubMed.
- N. Liu, S. Ni, A. J. Ragauskas, X. Meng, N. Hao and Y. Fu, J. Biotechnol., 2018, 269, 8–15 CrossRef PubMed.
- A. Aljawish, I. Chevalot, J. Jasniewski, J. Scher and L. Muniglia, J. Mol. Catal. B: Enzym., 2015, 112, 25–39 CrossRef.
- J. Liu, J. F. Lu, J. Kan and C. H. Jin, Int. J. Biol.
Macromol., 2013, 62, 321–329 CrossRef PubMed.
- R. J. Samuels, Solid state characterization of the structure of chitosan films, J. Polym. Sci., Polym. Chem. Ed., 1981, 19, 1081–1105 CrossRef.
- W. Pasanphan and S. Chirachanchai, Carbohydr. Polym., 2008, 72, 169–177 CrossRef.
- A. Jimenez, G. Creissen, B. Kular, J. Firmin, S. Robinson, M. Verhoeyen and P. Mullineaux, Planta, 2002, 214, 751–758 CrossRef PubMed.
- W. Xie, P. Xu and Q. Liu, Bioorg. Med. Chem. Lett., 2001, 11, 1699–1701 CrossRef PubMed.
- J. W. Chen, Z. Q. Zhu, T. X. Hu and D. Y. Zhu, Acta Pharmacol. Sin., 2002, 23, 667–672 Search PubMed.
- A. Mirto, F. Iannuzzi, P. Carillo, L. F. Ciarmiello, P. Woodrow and A. Fuggi, Food Chem., 2018, 240, 559–566 CrossRef PubMed.
- S. S. Gill and N. Tuteja, Plant Physiol. Biochem., 2010, 48, 909–930 CrossRef PubMed.
- A. Crozier, I. B. Jaganath and M. N. Clifford, Nat. Prod. Rep., 2009, 26, 1001–1043 RSC.
- P. M. Toivonen and D. A. Brummell, Postharvest Biol. Technol., 2008, 48, 1–14 CrossRef.
- E. Cocci, P. Rocculi, S. Romani and M. D. Rosa, Postharvest Biol. Technol., 2006, 39, 265–271 CrossRef.
- G. Kerch, Trends Food Sci. Technol., 2015, 46, 159–166 CrossRef.
- L. Vamos-Vigyazo, Crit. Rev. Food Sci. Nutr., 1981, 15, 49–127 CrossRef PubMed.
- O. Blokhina, E. Virolainen and K. V. Fagerstedt, Ann. Bot., 2003, 91, 179–194 CrossRef PubMed.
- B. Halliwell, Plant Physiol., 2006, 141, 312–322 CrossRef PubMed.
- F. R. Thewes, A. Brackmann, R. de Oliveira Anese, E. S. Bronzatto, E. E. Schultz and R. Wagner, Postharvest Biol. Technol., 2017, 127, 1–13 CrossRef.
- V. Giovenzana, R. Beghi, S. Buratti, R. Civelli and R. Guidetti, Talanta, 2014, 120, 368–375 CrossRef PubMed.
- K. Mishra, H. Ojha and N. K. Chaudhury, Food Chem., 2012, 130, 1036–1043 CrossRef.
- N. Smirnoff and Q. J. Cumbes, Phytochemistry, 1989, 28, 1057–1060 CrossRef.
- A. J. Sfahlan, A. Mahmoodzadeh, A. Hasanzadeh, R. Heidari and R. Jamei, Food Chem., 2009, 115, 529–533 CrossRef.
- X. Liu, J. Ren, Y. Zhu, W. Han, H. Xuan and L. Ge, Colloids Surf., A, 2016, 502, 102–106 CrossRef.
- M. Musci and S. Yao, Int. J. Food Sci. Nutr., 2017, 68, 913–918 CrossRef PubMed.
- O. W. Griffith, Anal. Biochem., 1980, 106, 207–212 CrossRef PubMed.
- L. Y. Wang, J. L. Liu, W. X. Wang and Y. Sun, Photosynthetica, 2016, 54, 19–27 CrossRef.
- M. Chisari, R. N. Barbagallo and G. Spagna, J. Agric. Food Chem., 2007, 55, 3469–3476 CrossRef PubMed.
- Q. Li, X. Yu, L. Xu and J. M. Gao, Food Chem., 2017, 221, 1113–1119 CrossRef PubMed.
- D. Granato, V. M. de Araújo Calado and B. Jarvis, Food Res. Int., 2014, 55, 137–149 CrossRef.
|
This journal is © The Royal Society of Chemistry 2018 |
Click here to see how this site uses Cookies. View our privacy policy here.