DOI:
10.1039/C8RA02543F
(Paper)
RSC Adv., 2018,
8, 20287-20294
Ag decorated silica nanostructures for surface plasmon enhanced photocatalysis†
Received
23rd March 2018
, Accepted 28th May 2018
First published on 4th June 2018
Abstract
In this article, we present a novel synthesis of mesoporous SiO2/Ag nanostructures for dye (methylene blue) adsorption and surface plasmon mediated photocatalysis. Mesoporous SiO2 nanoparticles with a pore size of 3.2 nm were synthesized using cetyltrimethylammonium bromide as a structure directing agent and functionalized with (3-aminopropyl)trimethoxysilane to introduce amine groups. The adsorption behavior of non-porous SiO2 nanoparticles was compared with that of the mesoporous silica nanoparticles. The large surface area and higher porosity of mesoporous SiO2 facilitated better adsorption of the dye as compared to the non-porous silica. Ag decorated SiO2 nanoparticles were synthesized by attaching silver (Ag) nanoparticles of different morphologies, i.e. spherical and triangular, on amine functionalized silica. The photocatalytic activity of the mesoporous SiO2/Ag was compared with that of non-porous SiO2/Ag nanoparticles and pristine Ag nanoparticles. Mesoporous SiO2 nanoparticles (kd = 31.3 × 10−3 g mg−1 min−1) showed remarkable improvement in the rate of degradation of methylene blue as compared to non-porous SiO2 (kd = 25.1 × 10−3 g mg−1 min−1) and pristine Ag nanoparticles (kd = 19.3 × 10−3 g mg−1 min−1). Blue Ag nanoparticles, owing to their better charge carrier generation and enhanced surface plasmon resonance, exhibited superior photocatalysis performance as compared to yellow Ag nanoparticles in all nanostructures.
Introduction
Synthetic dyes, having complex aromatic structures, are primary pollutants of various industries including textile, food, cosmetics, printing, electronics, paper, rubber, etc1. These dyes and their degradation products, being carcinogenic and mutagenic, are hazardous to the environment. Various physical and chemical procedures have been developed for treatment of industrial waste-water containing dyes before their disposal into the environment. The best employed method is adsorption, owing to the simple design and effectiveness in removal of dyes without resulting any harmful residues. Moreover, the recyclable nature of the adsorbents makes the process energy and cost effective also. A variety of adsorbents have been explored for removal of dyes such as silica,2,3 magnetic nanoparticles,4 activated carbon,5 zeolites,6 etc. The adsorption process is influenced by numerous factors including surface area of adsorbent, and extent and type of interaction of the dye with the absorbent.7
Mesoporous silica, due to its large surface area, high hydrothermal stability, and diverse surface functionality, has been explored extensively for biomedical applications, namely cell imaging, diagnosis, biosensing, intracellular drug delivery8–15 and controlled pesticide release.16 The presence of silanol groups ensures better adsorption of dye molecules on its surface.17 Researchers have investigated photocatalytic activity of various nanoparticles (NPs) including TiO2,18 ZnO,19,20 MnO2,21 Silver,22 etc. The optical spectra of metallic nanoparticles are dominated by Surface Plasmon Resonance (SPR), which is due to collective oscillation of conduction electrons. Silver nanoparticles (AgNPs) possess intense SPR, which is of great advantage for Surface Enhanced Raman Spectroscopy (SERS) and sensing applications. Recently AgNPs have been proposed as a novel photocatalyst for degradation of organic pollutants due to their SPR absorption in the visible range. Ag NPs, when excited with photon, energize conduction electrons of 5sp bands and excite them to higher-energy states. These excited electrons participate in chemical reactions.23 Additionally, holes left behind in the 5sp bands possess strong oxidizing power, and therefore act as driving force for photocatalysis. SPR frequency of Ag is a function of its particle size and shape. The Ag/SiO2 nanostructures with Ag as core material have been explored for sensing,24,25 antibacterial26 and SERS27 applications. Yellow AgNPs decorated non-porous SiO2/Ag core shell nanostructures have been investigated for plasmon mediated photocatalysis.28 On account of better charge carrier generation, blue AgNPs may improve photocatalytic performance of SiO2/blue Ag nanostructures in a significant manner. The large surface area of mesoporous SiO2 will further facilitate adsorption and hence catalysis.29
In the present work, we have made an attempt to synthesize mesoporous SiO2/Ag composite nanostructures. The adsorption behavior of non-porous silica (SiO2) and mesoporous silica (MS-SiO2) towards methylene blue dye has been investigated. Amine functionalized silica was then used for adsorption of AgNPs on its surface. The synthesized SiO2/Ag composite nanostructures were compared with pristine AgNPs for their photocatalytic behavior against methylene blue. The effect of porosity of silica and shape of AgNPs on photocatalysis was also investigated. MS-SiO2/Ag composite nanostructures are expected to have better adsorption of dye due to large surface area and porosity of mesoporous silica. The dye degradation process may get accelerated by plasmon assisted photocatalysis of Ag nanoparticles.
Experimental
Materials
The chemicals used were tetraethylorthosilicate (TEOS, ≥99%) (Merck, India), N,N,N-cetyltrimethylammonium bromide (CTAB) (Merck, India), (3-aminopropyl)trimethoxysilane (APTMS, 95%) (Merck, India), NaOH (Merck, India), NaBH4 (Merck, India), 30% w/v H2O2 (Merck, India), silver nitrate (Merck, India), sodium citrate (Merck, India), methylene blue (CDH, India), ethanol (Merck, India), 25% (w/v) NH3 solution (≥99%) (Fisher, India), poly(vinyl pyrrolidone) (Mw 40
000, CDH, India) and sodium dodecyl sulphate (SDS) (Merck, India). All the used chemicals were of analytical grade and used as received. Double deionized water was used in all the synthesis processes. All glassware items were first rinsed with aqua regia and thoroughly with deionized water followed by acetone before use.
Methods
Synthesis of non-porous silica nanoparticles using Stober method. Silica nanoparticles were prepared by the Stöber method. In brief, the precursor solution was prepared by adding 0.5 mL TEOS to 2 mL ethanol. The TEOS/ethanol solution was then added to a solution of 100 mL ethanol and 25 mL aqueous ammonia and stirred for 2 h at 40 °C. 8 mL of TEOS diluted with 32 mL ethanol was added in the above prepared solution and stirred for 2 h. Reaction mixture was then centrifuged at 5000 rpm and washed with water followed by ethanol. The precipitates were vacuum dried at 55 °C for 24 h followed by calcination at 450 °C for 2 h.
Synthesis of mesoporous silica nanoparticles. Mesoporous silica nanoparticles were synthesized using CTAB as structure directing agent and TEOS as precursor as reported by Cao et al.16 with a little modification. Briefly, 73 μL of 2 M NaOH (0.156 μmoles), and 0.0221 g (60.6 μmoles) of CTAB were dissolved in 10 mL of deionized water with stirring for 30 minutes at ambient temperature. The temperature was increased to 80 °C in an oil bath, and subsequently 104 μL of TEOS were added drop wise. The reaction time was kept 75 min. Reaction mixture was then centrifuged at 2000 rpm and washed with water followed by ethanol. The precipitates were vacuum dried at 55 °C for 24 h followed by calcination at 550 °C for 5 h.The morphology of obtained nanoparticles was analyzed using field emission scanning electron microscope (FE-SEM) (FEI Quanta 200F, Netherland) and high resolution transmission electron microscope (HRTEM) (JEM 2100F, JEOL, Tokyo Japan). Diameter of the nanoparticles was determined as an average of 200 readings using ImageJ software. Fourier Transform Infrared Spectroscopy (FTIR) (Bruker-Alpha, USA) was used for chemical structure characterization. The porous nature of the product was analyzed using N2 adsorption/desorption isotherms on Micromeritics ASAP 2010 Accelerated Surface Area and Porosity Analyzer. Data was evaluated using the BET method to calculate the surface area and pore size distribution, respectively.
Synthesis of silver nanoparticles. For the synthesis of silver nanoparticles, poly(vinyl pyrrolidone) (PVP) and sodium citrate were used as capping agent and H2O2 as etching agent. In a typical synthesis, 0.002 mmol of silver nitrate and 0.0092 mmol of PVP were dissolved in 50 mL of DI water at 60 °C. 900 μL of 30% H2O2 and 0.0551 mmol of sodium citrate were added to the solution with continuous stirring at 500 rpm. 900 and 500 μL of 0.177 mM solution of sodium borohydride were added into above prepared mixture of silver nitrate for synthesis of blue and yellow Ag, respectively. The reaction mixture was stirred for 30 minutes at 500 rpm. The obtained silver nanoparticles were characterized using UV spectrophotometer (Lambda 35, Perkin elmer, USA), particle size analyzer (Malvern Zetasizer Nano ZS, USA) and high resolution transmission electron microscope (HRTEM).
Functionalization of silica nanoparticles. The obtained silica nanoparticles were functionalized using APTMS. In a typical procedure 0.5 g of silica nanoparticles were dispersed in 5 mL of ammonia solution (25% w/v). 10% (v/v) of APTMS dissolved in chloroform was added drop wise to the above dispersion and kept for 1 h at 75 °C. Functionalized silica particles thus obtained were centrifuged and dried in vacuum oven 55 °C for 24 h for further use.
Adsorption of dye using non-porous and mesoporous silica nanoparticles. Solutions of 50, 100 and 200 mg L−1 methylene blue were prepared in DI water. The dye solutions were then used for adsorption study of both non-porous and mesoporous silica nanoparticles. The samples were analyzed using UV spectrophotometer and adsorption rate constant was calculated for each concentration.
Photocatalysis of methylene blue. 0.5 g of silica particles were dispersed in 5 mL of silver colloidal solution by stirring for 15 min followed by ultrasonication for 15 min and centrifugation at 5000 rpm. SiO2/Ag composite nanostructures settled down leaving behind the clear supernatant and were coded as SiO2/Ag and MS-SiO2/Ag for non-porous and mesoporous silica nanoparticles, respectively. The nanostructures were dried in vacuum oven and examined using HRTEM and EDX. Pristine, SiO2/Ag and MS-SiO2/Ag nanostructures were compared for their photocatalysis behavior using 200 mg L−1 methylene blue. For the study, 0.2% (w/v) of nanostructures were dispersed in methylene blue solution. The methylene blue solution with nanostructures was kept in dark to attain the adsorption/desorption equilibrium and then exposed to the sunlight for photodegradation. The photocatalytic behavior was analyzed using UV spectrophotometer (Scheme 1).
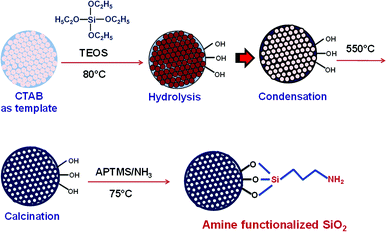 |
| Scheme 1 Synthesis and functionalization of mesoporous silica nanoparticles. | |
Results and discussion
Morphology of SiO2 and MS-SiO2 nanoparticles
The morphology of obtained SiO2 nanoparticles was analyzed using scanning electron microscope (SEM) and high-resolution transmission electron microscope (HRTEM). The SEM micrographs of non-porous silica (SiO2) and mesoporous silica (MS-SiO2) are shown in Fig. S1.† SEM analysis revealed that SiO2 and MS-SiO2 nanoparticles were of spherical shape with an average diameter of 250 ± 30 and 100 ± 12 nm respectively. HRTEM micrographs established the porous nature of the mesoporous silica (Fig. 1) with an estimated pore size of 3.0 ± 0.05 nm. This was further confirmed using BET analysis and the calculated surface area and adsorption average pore diameter was found to be 265 ± 10 m2 g−1 and 3.2 nm respectively.
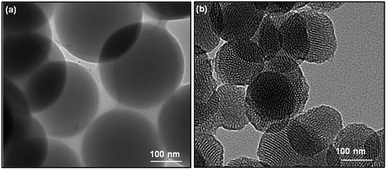 |
| Fig. 1 HRTEM micrographs of (a) SiO2 (b) MS-SiO2. | |
FTIR spectra of amine functionalized mesoporous silica nanoparticles are shown in Fig. S2.† The stretching vibrations of hydrogen-bonded Si–OH group, –OH of physically adsorbed water molecules and –NH2 groups were found as a broad peak at 3425 cm−1. The new peaks at 2959, 2852 and 1633 cm−1 were attributed to asymmetric and symmetric –C–H stretching of aminopropyl and –NH2 bending vibrations respectively.30 Splitting of Si–O–Si symmetric stretching peak at 772 cm−1 further confirmed interaction of Si–O–Si with –NH2 groups.
Characterization of silver nanoparticles
Particle size analysis and UV-Vis spectra of Ag NPs are shown in Fig. 2. The yellow AgNPs were found to have a narrow and small peak at 28 nm and a broad peak at 154 nm. The peak corresponding to 154 nm may be attributed to the aggregated AgNPs. The smaller size and high surface energy could result in larger aggregates. On the other hand, single peak corresponding to 56 nm was observed for blue Ag NPs.
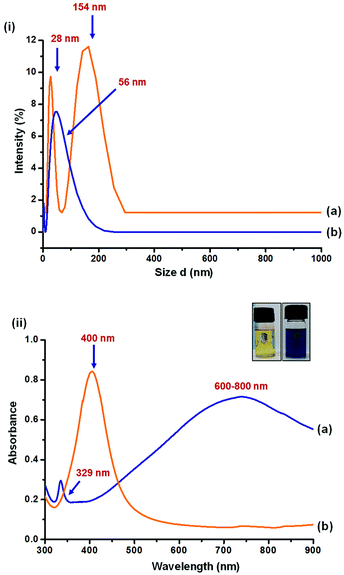 |
| Fig. 2 (i) Particle size analysis and (ii) UV-Vis spectra of (a) yellow Ag and (b) blue Ag. | |
UV-Vis spectra of the particles resemble with literature. Yellow AgNPs, being spherical in shape, were found to have in-plane dipole plasmon resonance peak at 400 nm. As reported in literature31 the in-plane dipole plasmon resonance of blue AgNPs was observed at 600–800 nm. An additional peak at 329 nm and a hump at 470 nm correspond to out-of-plane quadrupole and in-plane quadrupole plasmon resonance of triangular nanoparticles, respectively. Anisotropic triangular silver nanoparticles are reported32 to have high SERS intensity owing to their three surface plasmon resonance (SPR) bands corresponding to dipole and quadrupole plasmon resonance, while only one SPR band is reported for spherical silver nanoparticles.
HRTEM analysis was performed to investigate the morphology of AgNPs. As shown in Fig. 3, the yellow Ag was found to have spherical shape of nanoparticles with an average diameter of 25 ± 5 nm. Blue Ag was however a mixture of spherical as well as triangular nanoparticles as was observed in UV spectrum. The average dimension of nanoparticles was estimated as 40 ± 20 nm.
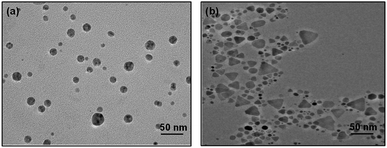 |
| Fig. 3 HRTEM images of (a) yellow Ag and (b) blue Ag. | |
Adsorption studies using non-porous and mesoporous silica
Adsorption kinetic experiments were performed for different dye concentrations i.e. 50, 100 and 200 mg L−1 with 0.1 g L−1 of SiO2 and MS-SiO2. The pseudo-second-order models were applied in order to understand adsorption mechanism. The expression for pseudo-second-order kinetic model is shown in eqn (1) where, qe (mg g−1) and qt (mg g−1) are the amounts of dye adsorbed at equilibrium and dye adsorbed at time t, respectively. |
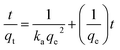 | (1) |
ka is the equilibrium rate constant of pseudo-second order adsorption (g mg−1 min−1). The slope and intercept of the plot of
versus t were used to calculate the rate constant ka (Fig. 4).
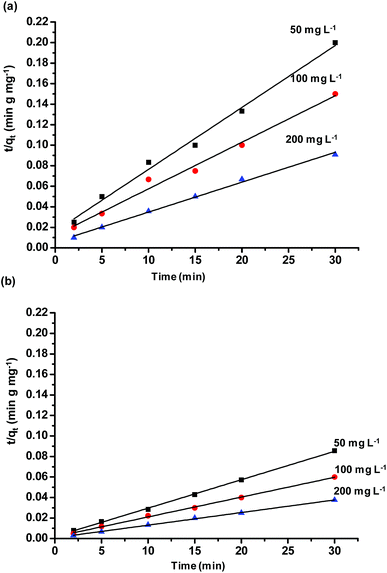 |
| Fig. 4 Effect of dye concentration on adsorption rate constant (ka) (a) SiO2 (b) MS-SiO2 silica. | |
The pseudo first order model was fitted to calculate the value of equilibrium dye concentration qe Cal and adsorption equilibrium rate constant ka. The obtained values of ka and qe Cal for different dye concentrations are mentioned in Table 1.
Table 1 Effect of dye concentration on ka for silica and mesoporous silica
|
Concentration of dye (mg L−1) |
50 |
100 |
200 |
SiO2 |
ka (g mg−1 min−1 × 10−3) |
2.21 |
1.66 |
1.45 |
qe Cal (mg g−1) |
166 |
222 |
344 |
qe Exp (mg g−1) |
150 |
200 |
330 |
![[thin space (1/6-em)]](https://www.rsc.org/images/entities/char_2009.gif) |
MS-SiO2 |
ka (g mg−1 min−1 × 10−3) |
3.92 |
2.01 |
1.8 |
qe Cal (mg g−1) |
357 |
526 |
833 |
qe Exp (mg g−1) |
350 |
500 |
800 |
The qe Cal values matches well with the experimental equilibrium dye concentration qe Exp values. The ka values reduced from 2.21 to 1.45 (g mg−1 min−1) and 3.92 to 1.80 (g mg−1 min−1) in non-porous and mesoporous silica respectively with an increase in dye concentration from 50 to 200 mg L−1.
This trend may be explained by the fact that at lower concentrations, a maximum number of dye molecules would be able to get adsorbed on silica surface, ensuring a higher adsorption. By contrast, at higher dye concentrations, a lower adsorption has been monitored due to the saturation of active adsorption sites. The ka values were found to be higher for mesoporous silica as compared to non-porous silica for all concentrations of dye. It may be inferred from the results that high porosity and large surface area of microporous silica facilitates the adsorption of methylene blue.
FTIR analysis was carried out to investigate the interaction of methylene blue with mesoporous silica nanoparticles leading to its strong adsorption. As shown in Fig. 5, the peak at 800 and 3000–3700 cm−1 corresponding to symmetric stretching of Si–O–Si and –OH respectively were found to be broadened. The broadening of peaks indicates interaction of –OH of silica nanoparticles with methylene blue. Further, the peak corresponding to asymmetric stretching of Si–O–Si at 1050–1150 cm−1 disappeared indicating the adsorption of dye molecules on MS-SiO2 nanoparticles.33 The adsorption of dye also resulted in disappearance of –NH stretching peak at 1633 cm−1 and enhancement of –C
C stretching at 1635 cm−1 due to adsorbed dye molecules. It may therefore be inferred that in addition to porous nature and large surface area, amino and hydroxyl group present on the surface of silica nanoparticles are likely to have weak hydrogen bonding with the dye molecules. This interaction facilitates the strong adsorption of dye on the surface of mesoporous silica nanoparticles as shown in Scheme 2.
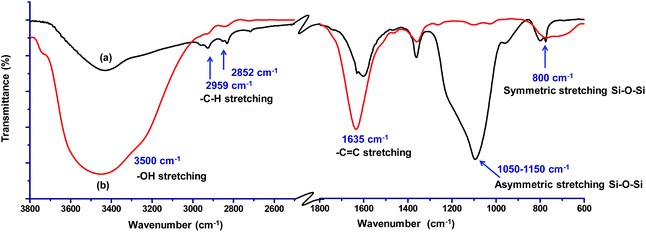 |
| Fig. 5 FTIR spectra of (a) amine functionalized MS-SiO2 nanoparticles and (b) dye adsorbed amine functionalized MS-SiO2 nanoparticles. | |
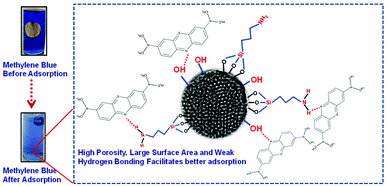 |
| Scheme 2 Mechanism of dye adsorption by amine functionalized MS-SiO2. | |
Morphology of SiO2/Ag composite nanostructures
SiO2 offers surface for adsorption of dye molecules, AgNPs, on the other hand, act as a good photocatalytic agent. HRTEM analysis of SiO2/Ag and MS-SiO2/Ag composite nanostructures are shown in Fig. 6 and 7 respectively. The AgNPs are clearly visible on the surface of SiO2 nanoparticles. The shape of AgNPs is spherical in case of SiO2/yellow Ag composite nanostructures while a mixture of spherical as well as triangular AgNPs was noticed in SiO2/blue Ag composite nanostructures.
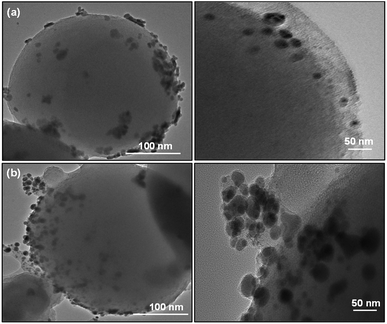 |
| Fig. 6 HRTEM images of (a) SiO2/yellow Ag and (b) SiO2/blue Ag. | |
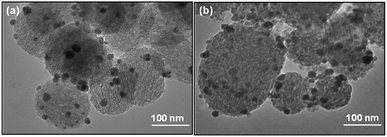 |
| Fig. 7 HRTEM images of (a) MS-SiO2/yellow Ag and (b) MS-SiO2/blue Ag. | |
The presence of Ag in MS-SiO2/blue Ag composite nanostructure was further confirmed by EDX (Energy Dispersive X-ray) analysis (Fig. 8). The EDX mapping results showed homogenous distribution of AgNPs was observed. The triangular shape of AgNPs was also confirmed by EDX mapping.
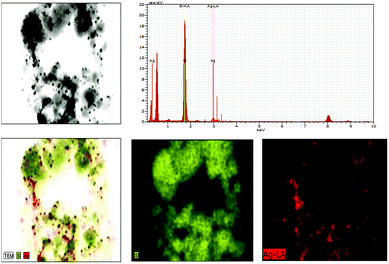 |
| Fig. 8 EDX mapping of MS-SiO2/blue Ag. | |
Photocatalytic behavior of SiO2/Ag composite nanostructures
0.2% (w/v) of Ag and SiO2/Ag composite nanostructures were compared for their photocatalytic activity. The nanoparticles were dispersed in dye solution (0.02% w/v) and placed in dark for 30 min to achieve adsorption–desorption equilibrium followed by exposure to sunlight and analysis of their photocatalytic behaviour.
The dye degradation behaviour of various nanostructures is shown in Fig. 9. The dye degradation was observed in all nanostructures, however, the extent of dye degradation was found to be a function of morphology of silica as well as AgNPs. The blue AgNPs exhibited ∼18% higher degradation as compared to yellow AgNPs. The triangular nanoparticles had two peaks in UV spectra indicating efficient SPR, which resulted in generation of larger number of reactive species leading to better dye degradation. The non-porous SiO2/Ag composite nanostructures were found to possess better photocatalysis behaviour as compared to pristine AgNPs. This may be attributed to adsorption of dye as evident from ka values already shown in Table 1.
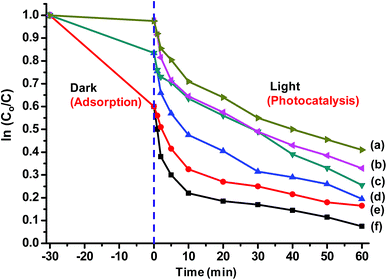 |
| Fig. 9 Photocatalytic behaviour of (a) yellow Ag (b) blue Ag (c) SiO2/yellow Ag (d) SiO2/blue Ag (e) MS-SiO2/yellow Ag and (f) MS-SiO2/blue Ag. | |
They also assisted in better dispersion of AgNPs on their surface leading to improved SPR mediated photocatalysis. The kd values for SiO2/yellow Ag and SiO2/blue Ag composite nanostructures were estimated as 19.9 × 10−3 and 25.1 × 10−3 g mg−1 min−1 respectively with an improvement of 22 and 30% compared to pristine AgNPs. The photocatalysis behaviour was further enhanced in the case of Ag decorated mesoporous SiO2 nanostructures. MS-SiO2/yellow Ag composite nanostructures were found to have kd value of 26.3 × 10−3 g mg−1 min−1 with an improvement of 61% compared to yellow AgNPs. MS-SiO2/blue Ag nanostructures, owing to better SPR mediated photocatalytic activity exhibited even better kd value of 31.3 × 10−3 g mg−1 min−1 (Table 2).
Table 2 Degradation rate constant kd values of Ag and SiO2/Ag composite nanostructures
Samples |
kd (g mg−1 min−1) × 10−3 |
Yellow Ag |
16.3 (R2 = 0.978) |
Blue Ag |
19.3 (R2 = 0.901) |
SiO2/yellow Ag |
19.9 (R2 = 0.878) |
SiO2/blue Ag |
25.1 (R2 = 0.86) |
MS-SiO2/yellow Ag |
26.3 (R2 = 0.822) |
MS-SiO2/blue Ag |
31.3 (R2 = 0.842) |
The porous nature and large surface area of MS-SiO2/Ag composite nanostructures not only resulted inbetter adsorption of methylene blue, but also showed higher photocatalytic degradation (improvement of 62% in kd value) due to surface plasmon mediated photocatalysis by triangular silver nanoparticles. It may therefore be deduced from the results that large surface area and porosity of mesoporous silica nanoparticles along with enhanced SPR of triangular AgNPs make them good adsorbing material as well as efficient photocatalyst. The proposed mechanism of photodegradation is shown in Scheme 3.
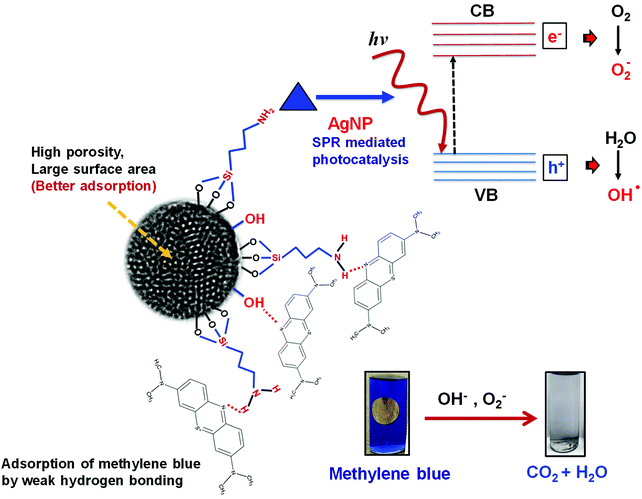 |
| Scheme 3 Photocatalytic mechanism of MS-SiO2/blue Ag composite nanostructures. | |
Conclusions
Non-porous and mesoporous silica nanoparticles were synthesized successfully and characterized using SEM and HRTEM. The estimated pore size of mesoporous silica NPs was found to be 3.2 nm. The adsorption behavior of amine functionalized non-porous and mesoporous silica nanoparticles was compared. The pseudo first order model was fitted to calculate the value of equilibrium dye concentration qe Cal and adsorption equilibrium rate constant ka The qe Cal values were found to match fairly well with the experimental equilibrium dye concentration qe Exp values. The increase in dye concentration from 50 to 200 mg L−1 resulted in decrease in ka values from 2.21 to 1.45 (g mg−1 min−1) and 3.92 to 1.8 (g mg−1 min−1) for non-porous and mesoporous silica respectively. The high porosity and large surface area of mesoporous silica facilitated the adsorption of methylene blue on its surface and the ka values were found to be higher for mesoporous silica as compared to non-porous silica for all the concentrations of dye. Ag decorated SiO2 nanoparticles were successfully synthesized by attaching yellow and blue AgNPs on the surface of amine functionalized silica. The photocatalytic activity of the mesoporous SiO2/Ag was compared with that of non-porous SiO2/Ag nanoparticles and pristine Ag nanoparticles. Mesoporous SiO2/Ag nanoparticles showed remarkable improvement in rate of degradation of methylene blue as compared to non-porous SiO2 and pristine Ag nanoparticles respectively. Blue Ag nanoparticles, owing to their better charge carrier generation and enhanced surface plasmon resonance, exhibited better photocatalysis performance as compared to yellow Ag nanoparticles among all the nanostructures. Large surface area and high porosity of mesoporous silica nanoparticles in combination with surface plasmon resonance of blue Ag contributed to significant improvement in photocatalysis of SiO2/Ag composite nanostructures.
Conflicts of interest
There are no conflicts to declare.
Acknowledgements
The authors would like to thank Bhaskaracharya College of Applied Sciences for providing the Laboratory facilities.
Notes and references
- N. Jain, R. Singh, G. Kumar, B. Pani, R. Nain, K. Dutt, P. K. Muwal and S. Sirohi, ChemistrySelect, 2017, 2, 11415–11421 CrossRef.
- S. M. Rafigh and A. Heydarinasab, ACS Sustainable Chem. Eng., 2017, 5, 10379–10386 CrossRef.
- K. Gude, V. M. Gun'ko and J. P. Blitz, Colloids Surf., A, 2008, 325, 17–20 CrossRef.
- H. Wang and Y. Huang, J. Hazard. Mater., 2011, 191, 163–169 CrossRef PubMed.
- B. Hameed, A. M. Din and A. Ahmad, J. Hazard. Mater., 2007, 141, 819–825 CrossRef PubMed.
- R. Han, J. Zhang, P. Han, Y. Wang, Z. Zhao and M. Tang, Chem. Eng. J., 2009, 145, 496–504 CrossRef.
- G. Wu, A. Koliadima, Y.-S. Her and E. Matijević, J. Colloid Interface Sci., 1997, 195, 222–228 CrossRef PubMed.
- Y. Chen, in Design, Synthesis, Multifunctionalization and Biomedical Applications of Multifunctional Mesoporous Silica-Based Drug Delivery Nanosystems, Springer, 2016, pp. 31–46 Search PubMed.
- L. Tang and J. Cheng, Nano Today, 2013, 8, 290–312 CrossRef PubMed.
- U. K. Sharma, M. Kushwaha, H. Tiwari and A. Prakash, World J. Pharm. Pharm. Sci., 2014, 11, 257–271 Search PubMed.
- X. Huang, N. P. Young and H. E. Townley, Nanomater. Nanotechnol., 2014, 4, 2–15 CrossRef.
- G. Zhang, J. Gao, J. Qian, L. Zhang, K. Zheng, K. Zhong, D. Cai, X. Zhang and Z. Wu, ACS Appl. Mater. Interfaces, 2015, 7, 14192–14200 Search PubMed.
- C. Bharti, U. Nagaich, A. K. Pal and N. Gulati, Int. J. Pharm. Invest., 2015, 5, 124 CrossRef PubMed.
- C. Argyo, V. Weiss, C. Bräuchle and T. Bein, Chem. Mater., 2013, 26, 435–451 CrossRef.
- S. Sharmiladevi, A. S. Priya and M. Sujitha, Int. J. Pharm. Pharm. Sci., 2016, 8, 196–201 Search PubMed.
- L. Cao, H. Zhang, C. Cao, J. Zhang, F. Li and Q. Huang, Nanomaterials, 2016, 6, 126 CrossRef PubMed.
- A. Krysztafkiewicz, S. Binkowski and T. Jesionowski, Appl. Surf. Sci., 2002, 199, 31–39 CrossRef.
- J. Schneider, M. Matsuoka, M. Takeuchi, J. Zhang, Y. Horiuchi, M. Anpo and D. W. Bahnemann, Chem. Rev., 2014, 114, 9919–9986 CrossRef PubMed.
- E. S. Elmolla and M. Chaudhuri, J. Hazard. Mater., 2010, 173, 445–449 CrossRef PubMed.
- G. Bandekar, N. Rajurkar, I. Mulla, U. Mulik, D. Amalnerkar and P. Adhyapak, Appl. Nanosci., 2013, 4, 199 CrossRef.
- S. Li, Z. Ma, L. Wang and J. Liu, Sci. China, Ser. B: Chem., 2008, 51, 179–185 CrossRef.
- Y. Badr and M. Mahmoud, J. Phys. Chem. Solids, 2007, 68, 413–419 CrossRef.
- X. Chen, Z. Zheng, X. Ke, E. Jaatinen, T. Xie, D. Wang, C. Guo, J. Zhao and H. Zhu, Green Chem., 2010, 12, 414–419 RSC.
- K. Aslan, M. Wu, J. R. Lakowicz and C. D. Geddes, J. Fluoresc., 2007, 17, 127–131 CrossRef PubMed.
- A. Nimrodh Ananth, S. Umapathy, J. Sophia, T. Mathavan and D. Mangalaraj, Appl. Nanosci., 2011, 1, 87–96 CrossRef.
- J. Alimunnisa, K. Ravichandran and K. S. Meena, J. Mol. Liq., 2017, 231, 281–287 CrossRef.
- W. Wang, Z. Li, B. Gu, Z. Zhang and H. Xu, ACS Nano, 2009, 3, 3493–3496 CrossRef PubMed.
- K. H. Chen, Y. C. Pu, K. D. Chang, Y. F. Liang, C. M. Liu, J. W. Yeh, H. C. Shih and Y. J. Hsu, J. Phys. Chem. C, 2012, 116, 19039–19045 Search PubMed.
- S. Sirohi, A. Singh, C. Dagar, G. Saini, B. Pani and R. Nain, Appl. Nanosci., 2017, 1–11 Search PubMed.
- G. Castruita-de León, Y. A. Perera-Mercado, L. A. García-Cerda, J. A. Mercado-Silva, H. I. Meléndez-Ortiz, Y. Olivares-Maldonado and L. Alvarez-Contreras, Microporous Mesoporous Mater., 2015, 204, 156–162 CrossRef.
- Q. Zhang, J. Ge, T. Pham, J. Goebl, Y. Hu, Z. Lu and Y. Yin, Angew. Chem., 2009, 121, 3568–3571 CrossRef.
- L. Lu, A. Kobayashi, K. Tawa and Y. Ozaki, Chem. Mater., 2006, 18, 4894–4901 CrossRef.
- H. T. Lu, Colloid J., 2013, 75, 311–318 CrossRef.
Footnote |
† Electronic supplementary information (ESI) available: SEM micrographs of non-porous and mesoporous silica and FTIR of amine functionalized silica. See DOI: 10.1039/c8ra02543f |
|
This journal is © The Royal Society of Chemistry 2018 |
Click here to see how this site uses Cookies. View our privacy policy here.