DOI:
10.1039/C8RA02422G
(Paper)
RSC Adv., 2018,
8, 24399-24410
Patchouli oil isolated from the leaves of Pogostemon cablin ameliorates ethanol-induced acute liver injury in rats via inhibition of oxidative stress and lipid accumulation
Received
20th March 2018
, Accepted 13th June 2018
First published on 5th July 2018
Abstract
Excessive alcohol consumption can cause serious hepatic injury which is associated with oxidative stress and fatty metabolic disturbance. Patchouli oil (PO) is a sort of food supplement with high medicinal value in hepatoprotection, but its ability against ethanol-induced liver failure has not been demonstrated. Thus, this study aimed to investigate the potential hepatoprotection of PO through an ethanol-induced hepatotoxicity rat model. Our results showed that PO pretreatment could dramatically decrease the levels of alanine aminotransferase (ALT), aspartate aminotransferase (AST), alkaline phosphatase (ALP) and lactate dehydrogenase (LDH) in serum, paralleled by an improvement of histopathology alterations. Additionally, PO could markedly suppress the content of reactive oxygen species (ROS), tumor necrosis factor alpha (TNF-α), free fatty acid (FFA), and triglyceride (TG), while enhancing the activities of glutathione (GSH), glutathione reductase (GR), and superoxide dismutase (SOD) as well as the ratio of glutathione to oxidized glutathione (GSH/GSSG) in liver. The protective effect of PO against oxidative stress was interrelated with restraining the mRNA and protein expression of hepatic microsomal enzyme cytochrome P450 2E1 (CYP2E1). What's more, PO pretreatment could also accelerate lipometabolism via up-regulating expressions of adenosine monophosphate-activated protein kinase (AMPK), peroxisome proliferator-activated receptor α (PPAR-α), and carnitine palmitoyltransferase 1 (CPT-1) and down-regulating expressions of nuclear factor-kappaB (NF-κB) p65, sterol regulatory element-binding protein 1 (SREBP-1c), fatty acid synthase (FAS), and stearoyl-CoA desaturase 1 (SCD-1). To conclude, PO showed potent effect against ethanol-induced hepatotoxicity by relieving oxidative stress and preventing lipid accumulation.
1. Introduction
Alcoholic liver injury (ALI) refers to liver disorders caused by long-term or large consumption of alcohol, which include alcoholic fatty liver, hepatitis, hepatic fibrosis and cirrhosis.1 With the increasing living and mental pressure, alcohol abuse prevails leading to high morbidity and even mortality rate of ALI.2 Hence, it is necessary to develop an effective agent to prevent the deterioration of ALI. Actually, some progresses have been made on the pathogenesis of ALI, but researches on specific medicine still remain stagnant.
ALI is a complex process mediated through some pathological mechanisms such as oxidative stress, lipid disorders, inflammatory response and apoptotic reaction, amongst which oxidative stress and abnormal lipid metabolism rank first for ALI occurrence.3,4 The interplay between oxidative stress and steatosis in ethanol-induced liver failure has been discussed by multiple researchers.5,6 Alcohol intake activates the activity of hepatic cytochrome P450 2E1(CYP2E1) that accelerates the alcohol oxidation and acetaldehyde accumulation.7 The interaction between acetaldehyde, proteins and lipids can lead to massive reactive oxygen species (ROS) formation and severe cell damage, and cause hepatic oxidative stress.8 It is reported that ROS are able to induce lipid metabolic disturbance by downregulating adenosine monophosphate-activated protein kinase (AMPK).9 AMPK can directly affect the expression of peroxisome proliferator-activated receptor alpha (PPARα) and carnitine palmitoyltransferase-1 (CPT-1), protecting liver from lipidosis.10 However, increased ROS might also trigger inflammatory response, initiating the nuclear factor kappa B (NF-κB) signaling pathway,11,12 and then producing various inflammatory mediators like tumor necrosis factor alpha (TNF-α).13–15 The release of TNF-α may stimulate the maturation of the sterol regulatory element binding protein-1c (SREBP-1c) and its downstream fatty acid synthase (FAS) as well as stearoyl-CoA desaturase 1 (SCD-1) in hepatocytes, indicating the initiation of lipometabolic disturbance.16,17 Therefore, it is of importance to develop effective therapies for ALI.
Herbal medicines have drawn much attention as an alternative treatment regimen to inhibit the progress of ALI owing to their plentiful resource, multi-targets and relatively wide range of safety.18 Pogostemon cablin (Blanco) Benth (Labiatae), a traditional Chinese herb with aromatic characteristic, is cultivated in Asia extensively, and is always used for the treatment of vomiting, influenza, exogenous fever, gastrointestinal disease and liver stagnation.19,20 Patchouli oil (PO) is the extractive from the dry leaves of Pogostemon cablin.21 PO nowadays is widely applied as an additive in health products industry and food industry.22 PO contains various bioactive substances and exerts biological activities of anti-oxidation, anti-inflammation and multiple-organ protection.23,24 In our previous research, we found that PO could scavenge the overproduction of ROS free radicals, thus relieving oxidative stress and cell necrosis.24 In addition, PO could also suppress NF-κB signaling pathway so as to decrease the release of pro-inflammatory cytokines and alleviate further inflammatory action.25,26 Recent pharmacological study has confirmed the protective effect of n-hexane extractive from Pogostemon patchouli against alcoholic liver injury.27 However, to the best of our knowledge, few reports have illustrated the protective property of PO against ethanol-caused hepatic damage. Therefore, our current study is designed to assess whether PO exploit positive effect on hepatotoxicity induced by alcohol.
2. Materials and methods
2.1 Chemicals and reagents
Pachouli oil (PO) was purchased from Nanhai Zhongnan Co., Ltd. (Foshan, China; Lot 140801). PO and silymarin were dissolved in tween-80 distilled water solution (2% wt/vol). Ethanol was diluted with distill water to 65% (v/v). Primary antibodies against CYP2E1, NF-κB p65, AMPK, PPAR-α, CPT-1, SREBP-1c, FAS, SCD-1, β-actin and secondary antibodies were purchased from Santa Cruz Biotechnology (Santa Cruz, CA, USA). Other required materials are obtained from Sigma Co. LLC. (Guangzhou, China) with highest purity commercially available.
2.2 Components determination of PO
PO was analyzed by gas chromatography-mass spectrometry (GC-MS), on an Agilent GC 7890A/MSD5975C instrument (Agilent Technologies Co. Ltd., Santa Clara, CA, USA). Samples (2 μL) dissolved in diethyl ether (1 mL) were injected onto the HP-5MS column (30 m × 250 μm × 0.5 μm). The temperature program was started at 100 °C. After keeping for 1 min, a gradient increase to 240 °C at a rate of 8°C min−1 and maintained for 5 min. Since the inlet temperature reached 250 °C, the helium carrier gas was used to support the pressure of 12 psi on the column with a constant flow rate of 1.3 mL min−1. Ion source temperature for mass spectrometer was 230 °C, operated in a splitless EI mode at 70 eV. The mass spectrum plot was applied using a full-scan monitoring mode with a mass scan range of m/z 35–400, and the splitting ratio was 60
:
1. The resulting peaks were identified by comparing the mass spectrums and retention index of compounds to those in the databases and values reported in the literatures.
2.3 Animals and experimental protocols
Male wistar rats (weighting 180–220 g, SCXK (YUE) 2013-0034) were purchased from Laboratory Animal Center of Guangzhou University of Chinese Medicine (Guangzhou, China). All performance accorded with the Animal Experimental Ethics Committee of Guangzhou University of Chinese Medicine (Guangzhou, China, no. 2016047). Prior to experimentation, rats were adaptively fed for 1 week with random diet and water under the controlled temperature (22 ± 2 °C) and humidity (55 ± 5%) with a 12 h light/dark cycle. Following acclimation, rats were randomly assigned into six groups (ten rats per group): normal control group (NC), ethanol group (Model), silymarin group (200 mg kg−1, Sily) as the positive control, and three PO pretreatment groups (100, 200 and 400 mg kg−1, respectively). Rats in Sily and PO groups were intragastrically administrated corresponding doses of drugs (10 mL kg−1) once a day, while those in the NC and Model groups received an equal volume of normal saline. The doses of PO and silymarin were ascertained based on the previous investigation and our preliminary experiments.28 After pretreatment for 7 consecutive days, all groups except NC group were given 65% ethanol (10 ml kg−1) intragastrically29 every 12 h at 6 different time points, which has successfully established severe liver injury model. At the end of the experimental period (the 11th day), all rats were injected with 1% pentobarbital sodium for anesthetization. Blood samples were collected for serum biochemistry tests and the livers were quickly dissected, weighed, frozen in liquid nitrogen, and then stored at −80 °C for further studies.
2.4 Blood biochemical studies
The blood samples were placed at room temperature for 30 min before centrifuging to obtain serum for biochemical estimations. Activities of lanine transaminase (ALT), aspartate amino transferase (AST) alkaline phosphatase (ALP), and lactate dehydrogenase (LDH) were assayed by commercial assay kits (Nanjing, Jiancheng) following the manufacturer's protocols.
2.5 Histopathological evaluation
Part of the liver samples were separated and kept in 10% formalin for 48 h and embedded in paraffin for histopathologic examination under a light microscope (Olympus IX71, Olympus Co., Tokyo, Japan).
2.6 Analysis of glutathione (GSH), glutathione reductase (GR), glutathione disulfide (GSSG), superoxide dismutase (SOD) and triglyceride (TG) level
Liver samples were homogenized in cold saline (1
:
9, w/v) before being centrifuged at 10
000 × g for 10 min at 4 °C. Then the supernatant was acquired to measure glutathione (GSH), glutathione disulfide (GSSG), glutathione reductase (GR), superoxide dismutase (SOD) and triglyceride (TG) level by using commercial assay kits (Nanjing, Jiancheng) according to standard instructions.
2.7 Measurement of tumor necrosis factor (TNF-α), reactive oxygen species (ROS) and free fatty acid (FFA) levels
Liver tissue were separated from rats and the content of tumor necrosis factor (TNF-α), reactive oxygen species (ROS) and free fatty acid (FFA) were assayed using specific ELISA kits (ImmunoWay Biotech, America) respectively according to standard instructions.
2.8 Reverse transcription-quantitative polymerase chain reaction (RT-qPCR)
Total RNA from liver samples were isolated using TRIzol reagent and then quantified by detecting the ratio of absorbance at 260 nm to that at 280 nm, and the purity was between 1.8 and 2.0. The level of cytochrome P450 2E1 (CYP2E1), nuclear factor-kappaB (NF-κB) p65, adenosine monophosphate-activated protein kinase (AMPK), peroxisome proliferator-activated receptor α (PPAR-α), carnitine palmitoyltransferase 1 (CPT-1), sterol regulatory element-binding protein 1 (SREBP-1c), stearoyl-CoA desaturase 1 (SCD-1), fatty acid synthase (FAS) and glyceraldehyde-3-phosphate dehydrogenase (GAPDH) in each sample was measured using HiScript® II Q RT SuperMix (+gDNA wiper) and ChamQ™ SYBR® qPCR Master Mix Kit according to the standard protocol provided by the manufacturer (Vazyme Biotech, China). Primer sequences are listed in Table 1. PCR amplification was performed as follows: pre-denaturation at 95 °C for 30 s, followed by 40 cycles of 95 °C for 10 s and 60 °C for 30 s. The relative quantification of gene expression was calculated using the 2−ΔΔCq method and GAPDH acted as an internal control.
Table 1 Primer sequences
Targeted gene |
Direction and sequence |
CYP2E1 |
F: 5′- ATG GCG GTT CTT GGC ATC ACC -3′ |
R: 5′- GTA GCC ATG CAG GAC CAC GAT G -3′ |
NF-κB p65 |
F: 5′- GGA ATC ATC CAG GCT GCT GAA CTG -3′ |
R: 5′- ATC ACG CCA CTC CTC GGT CAC -3′ |
AMPK |
F: 5′- AAC CGT TCT ATT GCC ACT CTG CTG -3′ |
R: 5′- TGG AGG CGA GGT AGA ACT CAC TG -3′ |
PPAR-α |
F: 5′- CTG AGG AAG CCA TTC TGC GAC ATC -3′ |
R: 5′- GCG TCT GAC TCG GTC TTC TTG ATG -3′ |
CPT-1 |
F: 5′- TGG CTA TGG TCA AGG TCC TCT CAG -3′ |
R: 5′- CAG CAG TAT GGC GTG GAT GGT G -3′ |
SREBP-1c |
F: 5′- ACG CCA TCT AGC CTG CCA CTG -3′ |
R: 5′- GGT GTT GAT GCC TGC GGT CTT C -3′ |
SCD-1 |
F: 5′- TCC TAC ACG ACC ACC ACT ACC ATC -3′ |
R: 5′- GCC GAG CCT TGT AAG TCC TGT G -3′ |
FAS |
F: 5′- CCG CCG AGT CCG AGT CTG TC -3′ |
R: 5′- CCG TGA GGT TGC TGT TGT CTG TAG -3′ |
GAPDH |
F: 5′- GTC CAT GCC ATC ACT GCC ACT C -3′ |
R: 5′- CGC CTG CTT CAC CAC CTT CTT G -3′ |
2.9 Western blotting analyses
Liver tissue were extracted from rats and homogenized in Cell Lysis Buffer in order to obtain total proteins and the concentrations were determined by Protein Assay Kit (Nanjing, Jiancheng). Equivalent protein (50–80 μg) from each sample were separated by SDS-PAGE and transferred to a PVDF membrane. After transfection, the membranes were blocked with 5% skimmed milk under the room temperature for 1 h and then incubated with primary antibody of 1
:
500 CYP2E1, 1
:
600 NF-κB p65, 1
:
500 AMPK, 1
:
500 PPAR-α, 1
:
300 CPT-1, 1
:
500 SREBP-1c, 1
:
500 SCD-1, 1
:
1000 FAS and 1
:
1000 β-actin at 4 °C overnight. Next, each membrane was washed 10 minutes for 3 times and incubated with 1
:
2000 HRP-conjugated secondary antibody for 2 h. Subsequently, the bands were visualized by ECL reagent and detected by Western Blotting Detection System (Amersham Life Science, UK). The density of each band was analyzed using Quantity One, software.
2.10 Statistical analysis
All values are exhibited as means ± standard deviation (mean ± SD), and a value of P < 0.05 was considered statistically significant. Statistical analysis was performed using a one-way analysis of variance (ANOVA), followed by an LSD test for multiple comparisons using SPSS software 20.0.
3. Results
3.1 GC-MS analysis of PO
The total ion chromatogram of PO was shown in Fig. 1. Seven compounds with relative content above 1% were identified in patchouli oils. The retention indices and relative proportion of these eleven compounds were listed in Table 1. As exhibited, patchouli alcohol took up the largest proportion (41.993%) in PO, followed by azulene at 23.532%. Besides, α-guaiene accounted for 15.173%. The relative contents of seychellene (7.395%), α-patchoulene (4.505%), β-patchoulene (3.968%) and globulol (3.434%) were all under 10%.
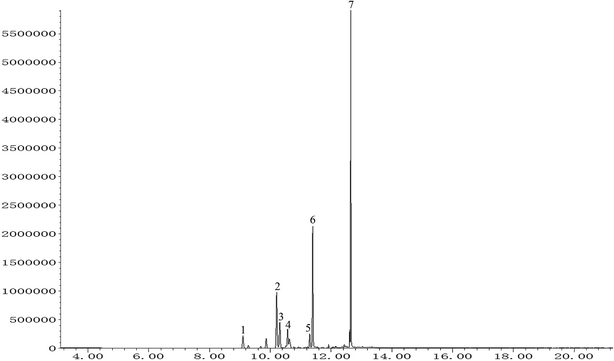 |
| Fig. 1 GC-MS analysis of patchouli oil. Numbers indicated on peaks corresponded to those in Table 1. | |
3.2 Effect of PO on ALT, AST, ALP and LDH levels
Compared with NC group (all p < 0.01), the activities of ALT, AST, ALP and LDH in Model group were dramatically increased by 494.2% (7.23 ± 1.49 vs. 42.96 ± 6.32), 494.4% (11.29 ± 2.01 vs. 67.11 ± 10.89), 121.5% (41.04 ± 5.55 vs. 90.92 ± 13.47), and 47.4% (1163.90 ± 57.62 vs. 1716.00 ± 145.03). However, pretreatment with silymarin and PO (200 and 400 mg kg−1) significantly suppressed these elevated trends (Fig. 2A–D, all p < 0.01). PO at 100 mg kg−1 also showed a similar effect, but there were no statistical significance (p > 0.05) on ALP and LDH expression. Compared with Model group, PO at 400 mg kg−1 exerted the best hepatic protection via reducing ALT, AST, ALP and LDH levels by 71.0%, 75.3%, 49.0% and 26.1%, respectively.
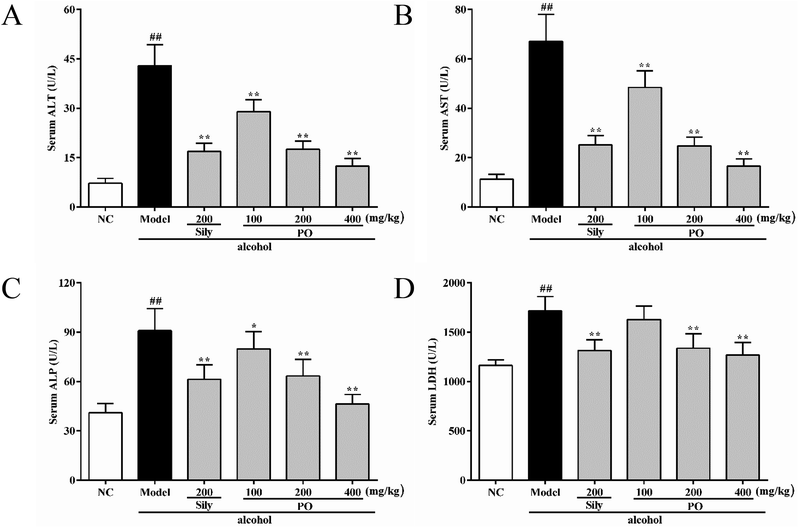 |
| Fig. 2 Effects of PO on levels of ALT, AST, ALP and LDH in liver. (A) ALT; (B) AST; (C) ALP; (D) LDH. Values were presented as the mean ± SD (n = 12). ##p < 0.01 vs. NC group; *p < 0.05, **p < 0.01 vs. Model group. | |
3.3 Histopathological analysis on H&E staining
As shown in Fig. 3A, liver cells of normal group were in compact arrangement (Fig. 3A). Central veins ran through the middle of the lobe, surrounded by hepatic cords that radiated out in all directions. However, liver tissues in Model group appeared serious granular denaturalization, fatty degeneration, inflammatory infiltration and hemorrhagic focus of hepatocytes (Fig. 3B). In contrast, after the administration of silymarin and PO (100, 200 and 400 mg kg−1), liver histopathological lesions were distinctly repaired, as evidence by the improvement of hepatocellular steatosis and the reduction in necrosis areas (Fig. 3C–F). Moreover, restored hepatic structure could be seen in PO group at 400 mg kg−1 with the fewest adipose vacuole attaching.
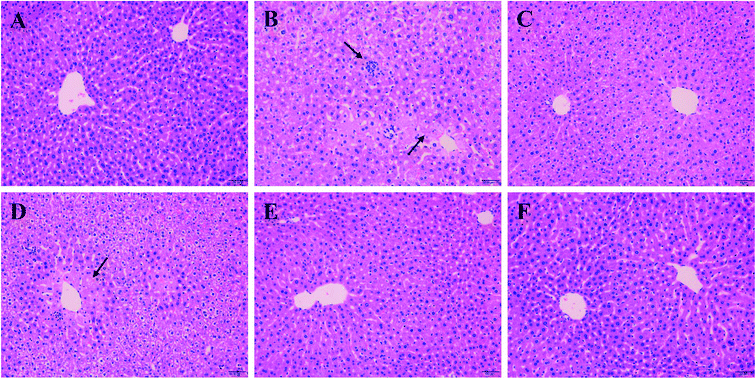 |
| Fig. 3 Effects of PO on histological evaluation in ethanol-induced liver damage (H&E staining, magnification 200×). (A) NC group: visible central veins and thin sinusoids; (B) alcohol Model group: severe liver damage, inflammatory infiltration and necrosis; (C) alcohol + silymarin group; (D) alcohol + PO 100 mg kg−1; (E) alcohol + PO 200 mg kg−1; (F) alcohol + PO 400 mg kg−1: well-formed hepatocytes and minor histopathology change. Arrows indicated both apparent focal necrosis and inflammatory infiltration. | |
3.4 Effect of PO on antioxidant ability and hepatic lipidosis
As shown in Fig. 4, compared with NC group (all p < 0.01), alcohol administration markedly decreased the levels of GSH, GR, SOD and the ratio of GSH/GSSG by 34.3% (200.18 ± 6.79 vs. 131.45 ± 13.22), 61.9% (4.04 ± 0.56 vs. 1.54 ± 0.21), 41.1% (68.45 ± 8.85 vs. 40.32 ± 6.02), and 77.8% (2.83 ± 0.53 vs. 0.63 ± 0.09), while increased the content of TG by 560% (0.10 ± 0.02 vs. 0.66 ± 0.10). Conversely, pretreatment with PO (200 and 400 mg kg−1) dramatically elevated the levels of GSH, GR, SOD and the ratio of GSH/GSSG but reduced the content of TG (all p < 0.05). Additionally, PO at 400 mg kg−1 could almost resume the anomalous situation to normal via ascending GSH, GR, SOD, the ratio of GSH/GSSG by 56.0%, 480%, 86.7%, 280% and descending TG by 74%, respectively, comparing with Model group.
 |
| Fig. 4 Effects of PO on antioxidant ability and hepatic lipidosis levels of GSH, GSH/GSSG, GR, SOD and TG in liver. (A) GSH; (B) GSH/GSSG; (C) GR; (D) SOD; (E) TG. Values were presented as the mean ± SD (n = 12). ##p < 0.01 vs. NC group; **p < 0.01 vs. Model group. | |
3.5 Effect of PO on ROS, TNF-α and FFA levels
Compared with NC group (Fig. 5, (all p < 0.01)), ROS, TNF-α and FFA levels significantly increased by 345.5% (55.70 ± 8.91 vs. 248.16 ± 19.50), 31.4% (208.44 ± 14.23 vs. 273.88 ± 26.79) and 132.4% (59.51 ± 9.73 vs. 138.32 ± 17.07) in Model group. PO administration (100, 200 and 400 mg kg−1) reversed these trends when compared with Model group. However, it should be noticed that PO at 100 mg kg−1 showed no statistical significance as compared with Model group (p > 0.05). In addition, PO at 400 mg kg−1 decreased the elevation (p > 0.01) of ROS, TNF-α and FFA by 68.3%, 27.0% and 53.8%, respectively. These data clearly manifested that PO could effectively protect the liver against ethanol-induced hepatotoxicity.
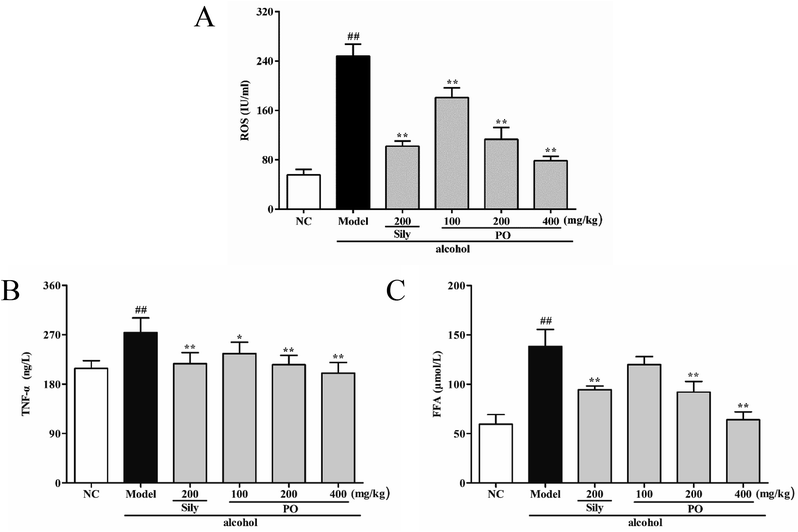 |
| Fig. 5 Effects of PO on levels of ROS, TNF-α and FFA in liver. (A) ROS; (B) TNF-α; (C) FFA. Values were presented as the mean ± SD (n = 12). ##p < 0.01 vs. NC group; *p < 0.05, **p < 0.01 vs. Model group. | |
3.6 Effect of PO on mRNA expression of CYP2E1, NF-κB p65, SREBP-1c, SCD-1, FAS, AMPK, PPAR-α, CPT-1
As shown in Fig. 6 and 7, compared with NC group, the result of RT-qPCR showed that exposure to alcohol could issue in downregulation of AMPK, PPAR-α and CPT-1 mRNA expressions (Fig. 6, p < 0.01) by 72.2% (1.00 ± 0.12 vs. 0.28 ± 0.09), 58.2% (1.00 ± 0.09 vs. 0.42 ± 0.11) and 65.8% (1.00 ± 0.12 vs. 0.34 ± 0.12), respectively, while upregulating CYP2E1, NF-κB p65, SREBP-1c, SCD-1, FAS mRNA expression (Fig. 7, p < 0.01) by 58.7% (1.00 ± 0.22 vs. 1.59 ± 0.20), 54.7% (1.00 ± 0.11 vs. 1.55 ± 0.20), 100% (1.00 ± 0.13 vs. 2.00 ± 0.20), 115% (1.00 ± 0.14 vs. 2.15 ± 0.22) and 212% (1.00 ± 0.24 vs. 3.12 ± 0.48). However, AMPK, PPAR-α and CPT-1 mRNA expressions in PO-administrated rats were significantly enhanced (all p < 0.05) in a dose-related manner while CYP2E1, NF-κB p65, SREBP-1c, SCD-1 and FAS mRNA expressions were markedly inhibited (all p < 0.05). Moreover, compared to the Model group, PO at 400 mg kg−1 could elevate expressions of AMPK, PPAR-α and CPT-1 by 272%, 142%, 200% and, at the same time, reduce expressions of CYP2E1, NF-κB p65, SREBP-1c, SCD-1 and FAS by 40.4%, 51.2%, 68.9%, 68.7% and 81.2%, respectively, suggesting that pretreatment with PO prevented ethanol-induced liver steatosis.
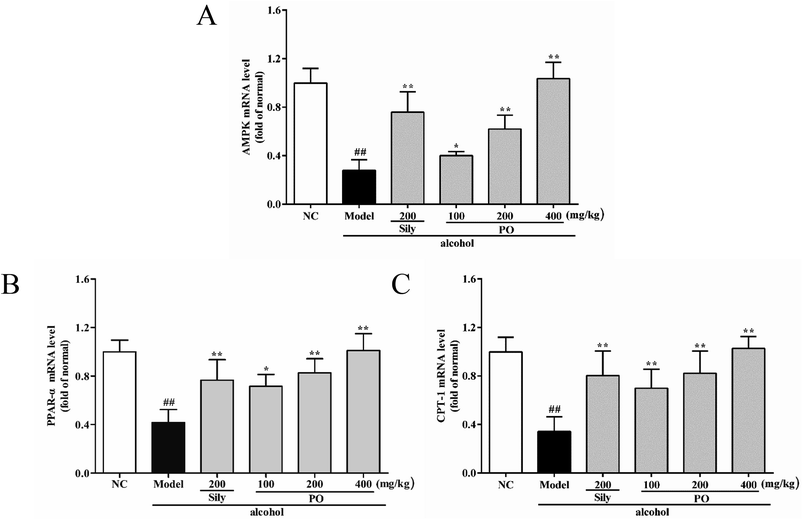 |
| Fig. 6 Effects of PO on the transcriptional levels of (A) AMPK, (B) PPAR-α and (C) CPT-1 in liver. Values were presented as the mean ± SD (n = 12). ##p < 0.01 vs. NC group; *p < 0.05, **p < 0.01 vs. Model group. | |
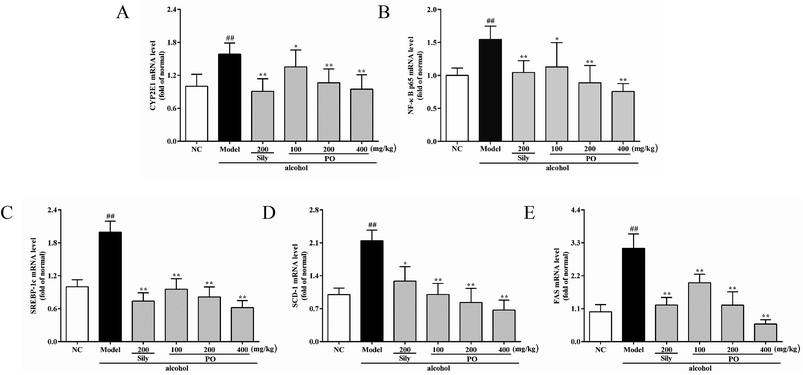 |
| Fig. 7 Effects of PO on the transcriptional levels of (A) CYP2E1, (B) NF-κB p65, (C) SREBP-1c, (D) SCD-1 and (E) FAS in liver. Values were presented as the mean ± SD (n = 12). ##p < 0.01 vs. NC group; *p < 0.05, **p < 0.01 vs. Model group. | |
3.7 Effects of PO on protein expression of CYP2E1, NF-κB p65, SREBP-1c, SCD-1, FAS, AMPK, PPAR-α, CPT-1
Compared with NC group, the results revealed that the protein expressions of AMPK, PPAR-α and CPT-1 were markedly inhibited by 29.9% (1.32 ± 0.17 vs. 0.92 ± 0.10), 15.9% (1.46 ± 0.01 vs. 1.23 ± 0.07), and 11.9% (1.26 ± 0.07 vs. 1.11 ± 0.06) while CYP2E1, NF-κB p65, SREBP-1c, SCD-1 and FAS were noticeably enhanced by 55.7% (0.80 ± 0.21 vs. 1.25 ± 0.27), 39.8% (0.82 ± 0.03 vs. 1.15 ± 0.07), 51.4% (0.69 ± 0.04 vs. 1.04 ± 0.07), 34.6% (0.88 ± 0.06 vs. 1.19 ± 0.05), 22.5% (0.94 ± 0.01 vs. 1.15 ± 0.05) after repeatedly exposing to alcohol (Fig. 8 and 9, p < 0.05). However, PO administration (100, 200 and 400 mg kg−1) were significantly increase the protein level of AMPK, PPAR-α and CPT-1 (all p < 0.05). Meanwhile, PO pretreatment also (100, 200 and 400 mg kg−1) exerted dose-dependent suppression of the protein level of CYP2E1, NF-κB p65, SREBP-1c, SCD-1 and FAS (all p < 0.05). When compared to the Model group, PO at 400 mg kg−1 elevated expressions of AMPK, PPAR-α and CPT-1 by 42.9%, 28.7%, 34.0% as well as reduced expressions of CYP2E1, NF-κB p65, SREBP-1c, SCD-1 and FAS by 47.3%, 35.3%, 32.4%, 25.3% and 27.0%, respectively.
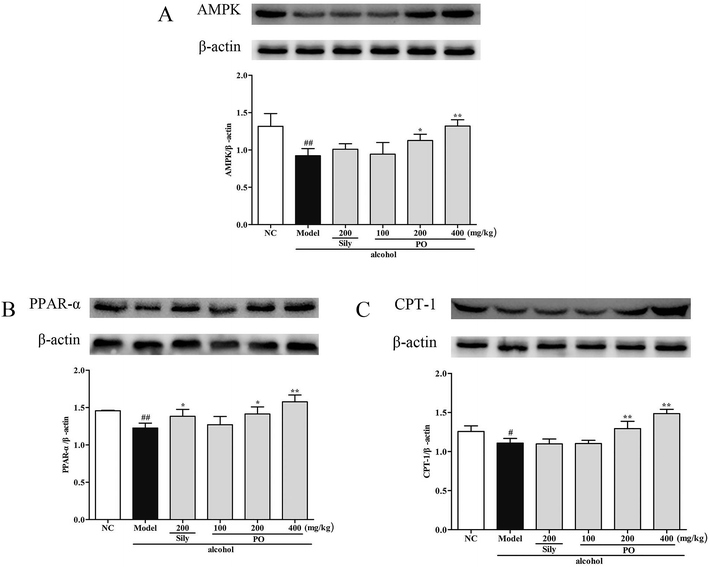 |
| Fig. 8 Effect of PO on protein expression of (A) AMPK, (B) PPAR-α and (C) CPT-1. Values were presented as the mean ± SD. #p < 0.05, ##p < 0.01 vs. NC group; *p < 0.05, **p < 0.01 vs. Model group. | |
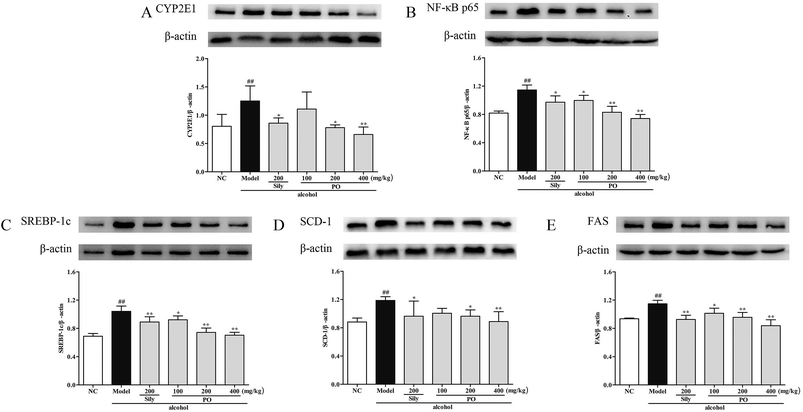 |
| Fig. 9 Effect of PO on protein expression of (A) CYP2E1, (B) NF-κB p65, (C) SREBP-1c, (D) SCD-1 and (E) FAS. Values were presented as the mean ± SD. ##p < 0.01 vs. NC group; *p < 0.05, **p < 0.01 vs. Model group. | |
4. Discussion
When alcohol enters into the organism, 90–98% of it is metabolized in liver. Excessive alcohol challenge results in irreparable hepatotoxicity and even death. Alterations of serum enzymes and hepatic histopathology can visually reflect physiological status of liver. Serum ALT, AST, ALP and LDH are particular biochemical indicators applied to assess liver function. Under the normal conditions, ALT, AST, ALP and LDH mainly spread in the liver cells, but they will be released into the bloodstream when hepatocytic membrane is attacked by exogenous poisons.30 Consequently, the augments in serum ALT, AST and ALP activity often signify possible liver injury, and the elevated LDH, a glycolytic ferment, implies acute hepatitis. In our present study, administration of alcohol to the rats led to hepatic toxicity as evidenced by an apparent ascension in serum ALT, AST, ALP and LDH compared to control group, which was in congruent with previous reports.31 Nevertheless, PO pretreatment could reverse these elevated trends, indicating its improvement in liver disorders. Alcohol also caused severe disruption to the hepatic structure, produced fat vacuoles and stimulated edema and diffusive infiltration of inflammatory cells. However, PO could significantly rehabilitate liver cellularity with explicit lobule and sinusoid, while diminish inflammatory infiltration and focal necrosis. It is revealed that PO might possess potent capacity against ethanol-induced liver damnification.
As a compensatory mechanism, oxidative stress is in a pivotal position of ethanol-caused hepatic lesion.32 Alcohol consumption increases the activity of CYP2E1 which accelerates the metabolism of alcohol and the formation of acetaldehyde.33 This catalytic activation of CYP2E1 enzyme requires molecular oxygen, along with generating a large quantity of harmful compounds—ROS.34 ROS can impair respiratory chain and DNA synthesis, increase the production of fatty substances and further destabilize the balance between oxidation and anti-oxidation.35 GSH, an endogenous antioxidant, can effectively capture superoxide and hydroxyl radicals to build up defenses against oxidative stress.36 The level of GSH represents the anti-oxidant ability of liver, but it can be oxidized as GSSG when being exposed to alcohol.37 The GSH and GSSG pair is an important actuator for redox equilibrium and homeostasis of GSH/GSSG plays a key role in maintaining liver stability.38 Once GSSG overproduces, antioxidase GR will rapidly convert it into GSH. SOD is an indispensable antioxidant enzyme participating in detoxification processes of excessive alcohol intake.34 SOD is the natural predator of oxyradicals, able to scavenge ROS and thus protect the liver from inflammation.39 In this study, pretreatment with PO could reverse the reduction in hepatic antioxidant GSH and antioxidative enzymes GR, SOD as well as GSH/GSSG ratio. Moreover, PO could also suppress the accumulation of ROS and decrease the protein and mRNA expressions of CYP2E1. It suggested that activating antioxidant system to counteract oxidative stress might ascribe to the protective effect of PO against alcoholic injury.
Recent research have found that alcohol might interfere with hepatic lipid metabolism, leading to hepatic lipid accumulation and ultimate steatosis. AMPK and SREBP-1c are associated with lipometabolic process and are able to regulate fatty acid biosynthesis.40 AMPK is a central component of protein kinase, which harmonize lipid and glucose in liver.41 Activated AMPK functions as a sensor of energy status that can coordinate anabolic metabolism and catabolism.42 However, when exposed to alcohol, the effect of AMPK signaling pathway on preventing steatosis might be weakened and steatosis would aggravate by ROS.43 Thus, AMPK provides an especially promising pharmacological target for ALD treatment. Reduced AMPK also causes a decrease in its downstream, PPAR-α and CPT-1, which are widely expressed in adipose liver.44 PPAR-α, a member of the nuclear hormone receptor family, is able to regulate hepatocellular fat formation. It has been elaborated that PPAR-α-knockout mice developed severe metabolic disorders and lipid aggregation after alcohol feeding.45,46 CPT-1, a central enzyme, can accelerate fatty acids crossing into mitochondrial inner membrane and activate rapid fat oxidation process.47 Excessive amount of alcohol severely prohibits CPT-1 gene and protein expression, which is strongly linked with hepatic lipidosis.48 PPAR-α and CPT-1 jointly established a protective barrier, contributing to alleviate fatty liver and reduce FFA delivery. In this experiment, we demonstrated that excessive alcohol could markedly lower the hepatic expressions of AMPK, PPAR-α and CPT-1, implying extensive cell lipid storage. These trends, however, were significantly reversed by PO supplement that implied PO exerting potent therapeutic efficacy on ethanol-induced liver failure.
SREBP-1c is able to impair glycolipid balance and induce lipogenesis via regulating lipid metabolic enzymes in hepatocytes. As shown in previous evidence, it can be up-regulated by highly expressed TNF-α, further inducing necrobiosis lipoidica.6,49 Alcohol consumption can stimulate Kupffer cells to generate ROS and subsequently activate NF-κB pathway, bringing on a high expression of TNF-α.50,51 Therefore, once TNF-α is inhibited, it could completely block the development of ethanol-induced hepatotoxicity.52,53 FAS and SCD-1, target genes of SREBP-1c, plays an important role in controlling the synthetic rate of FFA and TG.54,55 It has been reported that inhibiting FAS and SCD-1 could markedly improve ethanol-induced fatty liver.56 According to our findings, alcohol exposure distinctly increased both mRNA and protein expressions of SREBP-1c, NF-κB p65, FAS and SCD-1 and subsequently elevated the levels of TNF-α, FFA and TG. As expected, PO pretreatment could potently regulate these upward trends, suggesting that PO had protective effect against ethanol-induced liver injury via preventing lipogenesis.
The interaction between oxidative stress and lipid metabolism on ethanol-induced hepatotoxicity has been investigated in this study as well. Results indicated that oxidative stress could triggered metabolic dysfunction by impacting ROS activation. Our previous study has reported that PO possesses antioxidant and anti-inflammatory ability.23,24 Recent pharmacological research has also proved n-hexane extractive from Pogostemon patchouli could effectively protect liver against alcoholic injury via modulating the extent of lipid peroxidation and augmenting the antioxidant defense system. In this experiment, the satisfactory protection of PO was first elucidated in the liver injury of rats through raising antioxidant enzyme activity, depressing inflammatory reaction and averting lipid deposition, which was coordinated with predecessors's discoveries. Being a common food-stuff additive with valuable therapeutic effect, PO may be a potential candidate for preventing ethanol-induced liver damage.
According to our GC fingerprint, PO contains abundant sesquiterpenoids including patchouli alcohol, α-guaiene, seychellene, α-patchoulene and β-patchoulene. Patchouli alcohol comprises 41.993% and serves as the major constituent of PO. It has been reported that patchouli alcohol has various pharmacological activities, including anti-oxidation by inhibiting ROS accumulation57 and inflammation via suppressing NF-κB signaling pathway.58,59 Based on the hepatoprotection of PO, we speculate that patchouli alcohol might act as the main active ingredient to play a protective role in liver injury. As a result, we will keep on exploring this active component of PO on ethanol-induced hepatic damage in future study.
5. Conclusion
PO possessed a potent therapeutic effect on ethanol-induced liver injury, as evidenced by relieving oxidative lesions through inhibiting ROS production, and preventing fatty degeneration through accelerating adipose metabolism. This study provided an original perspective that PO was a functional natural agent for the treatment of ethanol-induced liver damage. However, more extraneous experiments are required to further elucidate the hepatoprotective molecular mechanisms of PO and the active ingredients of PO will be explored in ethanol-induced hepatic damage in future study.
Conflicts of interest
The authors declare that they have no conflicts of interest.
Acknowledgements
This work was supported by grants from Science and Technology Major Project of Guangdong Province (No. 2013A022100001), Guangdong Provincial Department of Education Feature Innovation Project (No. 2016KTSCX018), Key Disciplines Construction Projects of High-level University of Guangdong Province.
References
- T. Bai, et al., Betulin alleviated ethanol-induced alcoholic liver injury via SIRT1/AMPK signaling pathway, Pharmacol. Res., 2016, 105, 1–12 CrossRef PubMed.
- J. M. Schwartz and J. F. Reinus, Prevalence and natural history of alcoholic liver disease, Clin. Liver Dis., 2012, 16(4), 659 CrossRef PubMed.
- S. K. Das and D. M. Vasudevan, Alcohol-induced oxidative stress, Life Sci., 2007, 81(3), 177 CrossRef PubMed.
- A. Rocco, et al., Alcoholic disease: Liver and beyond, World J. Gastroenterol., 2014, 20(40), 14652–14659 CrossRef PubMed.
- C. Lu, et al., Ligustrazine prevents alcohol-induced liver injury by attenuating hepatic steatosis and oxidative stress, Int. Immunopharmacol., 2015, 29(2), 613–621 CrossRef PubMed.
- F. Wang, et al., Apigenin protects against alcohol-induced liver injury in mice by regulating hepatic CYP2E1-mediated oxidative stress and PPARα-mediated lipogenic gene expression, Chem.-Biol. Interact., 2017, 275, 171 CrossRef PubMed.
- Y. W. Cao, et al., Protective effects of Penthorum chinense Pursh against chronic ethanol-induced liver injury in mice, J. Ethnopharmacol., 2015, 161, 92–98 CrossRef PubMed.
- D. Wu and A. I. Cederbaum, Oxidative Stress and Alcoholic Liver Disease, Semin. Liver Dis., 2009, 29(02), 141–154 CrossRef PubMed.
- B. Sid, J. Verrax and P. B. Calderon, Role of AMPK activation in oxidative cell damage: Implications for alcohol-induced liver disease, Biochem. Pharmacol., 2013, 86(2), 200–209 CrossRef PubMed.
- A. Louvet and P. Mathurin, Alcoholic liver disease: mechanisms of injury and targeted treatment, Nat. Rev. Gastroenterol. Hepatol., 2015, 12(4), 231–242 CrossRef PubMed.
- S. W. Chae, Function and Activation of NF-kappa B in Immune System, J. Cell Biol., 2005, 33(2), 307–318 Search PubMed.
- M. Frankenberger, et al., Constitutive nuclear NF-kappa B in cells of the monocyte lineage, Biochem. J., 1994, 304(1), 87 CrossRef PubMed.
- E. Abraham, Nuclear factor-kappa B and its role in sepsis-associated organ failure, J. Infect. Dis., 2003, 187, S364–S369 CrossRef PubMed.
- L. Flohe, et al., Redox regulation of NF-kappa B activation, Free Radicals Biol. Med., 1997, 22(6), 1115–1126 CrossRef PubMed.
- M. Yin, et al., Essential role of tumor necrosis factor alpha in alcohol-induced liver injury in mice, Gastroenterology, 1999, 117(4), 942–952 CrossRef.
- T. Nakajima, et al., Peroxisome proliferator-activated receptor alpha protects against alcohol-induced liver damage, Hepatology, 2004, 40(4), 972–980 CrossRef PubMed.
- H. Q. Yin, et al., Differential gene expression and lipid metabolism in fatty liver induced by acute ethanol treatment in mice, Toxicol. Appl. Pharmacol., 2007, 223(3), 225–233 CrossRef PubMed.
- R. B. Ding, et al., Herbal medicines for the prevention of alcoholic liver disease: A review, J. Ethnopharmacol., 2012, 144(3), 457–465 CrossRef PubMed.
- M. K. Swamy and U. R. Sinniah, A Comprehensive Review on the Phytochemical Constituents and Pharmacological Activities of Pogostemon cablin Benth.: An Aromatic Medicinal Plant of Industrial Importance, Molecules, 2015, 20(5), 8521–8547 CrossRef PubMed.
- M. W. Chen, et al., Analysis of Pogostemon cablin from pharmaceutical research to market performances, Expert Opin. Invest. Drugs, 2013, 22(2), 245–257 CrossRef PubMed.
- P. Kraft, C. Weymuth and C. Nussbaumer, Cover Picture: Total Synthesis and Olfactory Evaluation of (1R*,3S*,6S*,7S*,8S*)-3-Hydroxy-6,8-dimethyltricyclo[5.3.1.03,8]undecan-2-one: A New Synthetic Route to the Patchoulol Skeleton (Eur. J. Org. Chem. 6/2006), Eur. J. Org. Chem., 2010, 2006(6), 1341 CrossRef.
- V. R. Preedy, Essential Oils in Food Preservation, Flavor and Safety, 2016 Search PubMed.
- K. H. Woo, et al., Pogostemon cablinas ROS Scavenger in Oxidant-induced Cell Death of Human Neuroglioma Cells. Evidence-based complementary and alternative medicine, eCAM, 2010, 7(2), 239 Search PubMed.
- Y. C. Li, et al., Anti-inflammatory activity of patchouli alcohol isolated from Pogostemonis Herba in animal models, Fitoterapia, 2011, 82(8), 1295–1301 CrossRef PubMed.
- Z. B. Zhang, et al., Anti-inflammatory activity of beta-patchoulene isolated from patchouli oil in mice, Eur. J. Pharmacol., 2016, 781, 229–238 CrossRef PubMed.
- J. L. Liang, et al., Patchoulene Epoxide Isolated from Patchouli
Oil Suppresses Acute Inflammation through Inhibition of NF-kappa B and Downregulation of COX-2/iNOS, Mediators Inflammation, 2017, 1089028 Search PubMed.
- P. P. Dongare, et al., Antihepatotoxic activity of Pogostemon patchouli against alcohol-induced hepatotoxicity in rats, Int. J. Adv. Res., 2013, 1(9), 225–234 Search PubMed.
- Z. A. Zakaria, et al., Methanol extract of Dicranopteris linearis L. leaves impedes acetaminophen-induced liver intoxication partly by enhancing the endogenous antioxidant system, BMC Complementary Altern. Med., 2017, 17(1), 271 CrossRef PubMed.
- Z. H. Ma, et al., Resveratrol improves alcoholic fatty liver disease by downregulating HIF-1 alpha expression and mitochondrial ROS production, PLoS One, 2017, 12(8), 11 Search PubMed.
- H. Singh, et al., Hepatoprotective effect of trans-Chalcone on experimentally induced he, Can. J. Physiol. Pharmacol., 2016, 94(8), 1–9 CrossRef PubMed.
- Q. H. Huang, et al., Polydatin Protects Rat Liver against Ethanol-Induced Injury: Involvement of CYP2E1/ROS/Nrf2 and TLR4/NF-kappa B p65 Pathway, Evidence-Based Complementary and Alternative Medicine, 2017, p. 14 Search PubMed.
- R. Guo and J. Ren, Alcohol and Acetaldehyde in Public Health: From Marvel to Menace, Int. J. Environ. Res. Public Health, 2010, 7(4), 1285–1301 CrossRef PubMed.
- Y. W. Cao, et al., Protective effects of Penthorum chinense Pursh against chronic ethanol-induced liver injury in mice, J. Ethnopharmacol., 2015, 161, 92–98 CrossRef PubMed.
- D. F. Wu and A. I. Cederbaum, Oxidative Stress and Alcoholic Liver Disease, Semin. Liver Dis., 2009, 29(2), 141–154 CrossRef PubMed.
- H. T. Shi, et al., Chlorogenic acid protects against liver fibrosis in vivo and in vitro through inhibition of oxidative stress, Clin. Nutr., 2016, 35(6), 1366–1373 CrossRef PubMed.
- Y. H. Tien, et al., Hepatoprotective and Anti-oxidant Activities of Glossogyne tenuifolia Against Acetaminophen-Induced Hepatotoxicity in Mice, Am. J. Chin. Med., 2014, 42(6), 1385–1398 CrossRef PubMed.
- D. E. Jensen, G. K. Belka and G. C. Du Bois, S-Nitrosoglutathione is a substrate for rat alcohol dehydrogenase class III isoenzyme, Biochem. J., 1998, 331, 659–668 CrossRef PubMed.
- L. Cao, et al., Ratios of biliary glutathione disulfide (GSSG) to glutathione (GSH): a potential index to screen drug-induced hepatic oxidative stress in rats and mice, Anal. Bioanal. Chem., 2013, 405(8), 2635–2642 CrossRef PubMed.
- I. Fridovich, Superoxide anion radical (O-2 radical anion), superoxide dismutases, and related matters, J. Biol. Chem., 1997, 272(30), 18515–18517 CrossRef PubMed.
- H. I. Lee, et al., Scopoletin prevents alcohol-induced hepatic lipid accumulation by modulating the AMPK-SREBP pathway in diet-induced obese mice, Metab., Clin. Exp., 2014, 63(4), 593–601 CrossRef PubMed.
- W. W. Winder and D. G. Hardie, AMP-activated protein kinase, a metabolic master switch: possible roles in Type 2 diabetes, Am. J. Physiol.: Endocrinol. Metab., 1999, 277(1), E1–E10 CrossRef.
- D. Li, et al., Adenosine Monophosphate-activated Protein Kinase Induces Cholesterol Efflux from Macrophage-derived Foam Cells and Alleviates Atherosclerosis in Apolipoprotein E-deficient Mice, J. Biol. Chem., 2010, 285(43), 33499–33509 CrossRef PubMed.
- J. Garcia-Villafranca, A. Guillen and J. Castro, Ethanol consumption impairs regulation of fatty acid metabolism by decreasing the activity of AMP-activated protein kinase in rat liver, Biochimie, 2008, 90(3), 460–466 CrossRef PubMed.
- M. J. Kim, et al., Dietary umbelliferone attenuates alcohol-induced fatty liver via regulation of PPARα and SREBP-1c in rats, Alcohol, 2014, 48(7), 707–715 CrossRef PubMed.
- C. Q. Rogers, J. M. Ajmo and M. You, Adiponectin and alcoholic fatty liver disease, IUBMB Life, 2008, 60(12), 790–797 CrossRef PubMed.
- W. W. Chaung, et al., Suppression of PGC-1alpha by Ethanol: Implications of Its Role in Alcohol Induced Liver Injury, Int. J. Clin. Exp. Med., 2008, 1(2), 161 Search PubMed.
- S. H. Korman, et al., Novel metabolic and molecular findings in hepatic carnitine palmitoyltransferase I deficiency, Mol. Genet. Metab., 2005, 86(3), 337 CrossRef PubMed.
- S. Wada, et al., Fish oil fed prior to ethanol administration prevents acute ethanol-induced fatty liver in mice, J. Hepatol., 2008, 49(3), 441 CrossRef PubMed.
- M. Endo, et al., TNF-alpha induces hepatic steatosis in mice by enhancing gene expression of sterol regulatory element binding protein-1c (SREBP-1c), Exp. Biol. Med., 2007, 232(5), 614 Search PubMed.
- R. G. Thurman, Mechanisms of Hepatic Toxicity II. Alcoholic liver injury involves activation of Kupffer cells by endotoxin, Am. J. Physiol.: Gastrointest. Liver Physiol., 1998, 275(4), G605–G611 CrossRef.
- M. D. Wheeler, et al., The role of kupffer cell oxidant production in early ethanol-induced liver disease and 12, Free Radical Biol. Med., 2001, 31(12), 1544–1549 CrossRef PubMed.
- C. Ji, Q. Deng and N. Kaplowitz, Role of TNF-alpha in ethanol-induced hyperhomocysteinemia and murine alcoholic liver injury, Hepatology, 2004, 40(2), 442–451 CrossRef PubMed.
- D. Keppler, Uptake and Efflux Transporters for Conjugates in Human Hepatocytes, Methods Enzymol., 2005, 400, 531–542 Search PubMed.
- M. You, et al., Ethanol induces fatty acid synthesis pathways by activation of sterol regulatory element-binding protein (SREBP), J. Biol. Chem., 2002, 277(32), 29342–29347 CrossRef PubMed.
- P. Ferré and F. Foufelle, Hepatic steatosis: a role for de novo lipogenesis and the transcription factor SREBP-1c, Diabetes, Obes. Metab., 2010, 12(s2), 83–92 CrossRef PubMed.
- K. Tomita, et al., Pioglitazone prevents alcohol-induced fatty liver in rats through up-regulation of c-Met, Gastroenterology, 2004, 126(3), 873–885 CrossRef.
- X. X. Feng, et al., Effects of topical application of patchouli alcohol on the UV-induced skin photoaging in mice, Eur. J. Pharm. Sci., 2014, 63, 113–123 CrossRef PubMed.
- J. L. Yu, et al., Patchouli alcohol protects against lipopolysaccharide-induced acute lung injury in mice, J. Surg. Res., 2015, 194(2), 537–543 CrossRef PubMed.
- X. Y. Chen, et al., beta-Patchoulene from patchouli oil protects against LPS-induced acute lung injury via suppressing NF-kappa B and activating Nrf2 pathways, Int. Immunopharmacol., 2017, 50, 270–278 CrossRef PubMed.
Footnote |
† These authors contributed equally to this work. |
|
This journal is © The Royal Society of Chemistry 2018 |