DOI:
10.1039/C8RA02183J
(Paper)
RSC Adv., 2018,
8, 19827-19834
Near infrared light activatable PEI-wrapped bismuth selenide nanocomposites for photothermal/photodynamic therapy induced bacterial inactivation and dye degradation†
Received
12th March 2018
, Accepted 1st May 2018
First published on 30th May 2018
Abstract
The inactivation of bacteria and the degradation of organic pollutants by engineered nanomaterials (NMs) are very effective approaches for producing safe and clean drinking water. The development of new NMs which can act as NIR light mediated antimicrobial agents as well as photocatalytic agents is highly desired. In this study, a novel Bi2Se3 nanoplates (NPs) NM was prepared by a high-temperature reaction (colloidal synthesis) followed by wrapping of the surface with polyethyleneimine (PEI) through electrostatic interactions. The developed Bi2Se3 NPs/PEI exhibited excellent NIR light activated antimicrobial properties for bacterial eradication and efficient photocatalytic properties for organic dye degradation. The results showed that upon 808 nm laser irradiation the engineered Bi2Se3 NPs/PEI eradicated ∼99% of S. aureus and ∼97% of E. coli bacteria within 10 minutes of irradiation through combined dual-modal photothermal therapy (PTT) and photodynamic therapy (PDT) via the generation of heat and reactive oxygen species, respectively. The contributions of PTT and PDT were found to be in a ratio of nearly 4
:
1 in the killing of both species of bacteria. In addition, Bi2Se3 NPs/PEI also acted as an excellent photocatalyst under illumination by a halogen lamp equipped with a 700–1100 nm band pass filter to achieve degradation efficiencies of ∼95% for methylene blue and ∼93% for Rhodamine B within 3 and 4 h, respectively. To the best of our knowledge, this is the first demonstration of these NIR light activated antimicrobial properties, photodynamic properties and photocatalytic properties mediated by Bi2Se3 NPs.
Introduction
There are growing concerns about drinking water safety, requiring the removal of contaminants such as toxic pathogenic microorganisms like Staphylococcus aureus (S. aureus) and Escherichia coli (E. coli) as well as organic pollutants.1 The conventional method for treating bacterial infections is the usage of antibiotics.2 However, the overusage of antibiotics inevitably leads to the development of antibiotic-resistant bacterial strains, which potentially becomes a serious threat to public health.3 Therefore, it is necessary to develop novel antibacterial materials and/or new approaches for the effective killing of pathogenic bacteria. In recent times, phototherapy, including photothermal therapy (PTT)4,5 and photodynamic therapy (PDT)6 has attracted great attention as a capable antimicrobial technique due to its high therapeutic efficiency and minimal side effects.
In PTT, photoabsorbing agents are delivered to the target bacteria, and are then irradiated with a near infrared (NIR, 700–1100 nm) light and the absorbed optical energy is converted into heat energy to cause cell damage and subsequent bacterial eradication.7 Various photothermal agents (PTA) such as gold nanomaterials (NMs),8,9 graphene based NMs,10,11 and organic nanoparticles12 have been explored for efficient photothermal bacterial eradication. Nevertheless, it is highly desirable to develop and engineer new PTAs that can effectively target and destroy bacteria.
Alternatively, PDT is an approach which involves the use of photosensitizers (PSs), light, and molecular oxygen (3O2) to induce the formation of singlet oxygen (1O2) and other reactive oxygen species (ROS), which are able to kill pathogenic bacteria.13 In this method, organic dyes, for example phthalocyanines, porphyrins, and chlorins etc., have been used as PSs. However, the adverse effects of these dyes, such as skin infections and oral disinfection, limit their clinical applicability.14 In addition, most of these PSs are typically activated by using UV/visible light, which has limited tissue penetration depths. Recently, a few studies have focused on the design of new PSs that can generate ROS and destroy bacteria upon NIR light irradiation.13,15–17 There is an urgent need to develop and engineer new PSs that can effectively target and destroy bacteria.
Of late, dual-modal phototherapeutics have been developed that combine PTT and PDT and exhibit synergistically enhanced therapeutic efficacy compared to PTT or PDT alone in cancer treatment.18 However, the exploration of dual-mode phototherapeutics in bacterial eradication is still in its infancy stage.19 Therefore, the development of dual-modal antimicrobial agents with bacteria targeting ability to simultaneously deliver both PTT and PDT under single laser irradiation for bacterial eradication is highly significant.
Similar to bacteria, organic pollutants in waste water also adversely affect human health.20 Currently, NM based photocatalytic technology for the degradation of organic pollutants has been regarded as one of the most promising routes for the decontamination of waste water.21 This photocatalytic technology is a process in which photoenergy converts into chemical energy and generates ROS for dye degradation.22 Until now, various NMs have been investigated for photocatalytic activity, including Bi2WO6/BiVO4,22 Bi2O3,21 poly(methyl methacrylate)/TiO2,23 Ag nanoparticles/BiVO4,24 and CNT/AgI.25 However, most of these photocatalysts require UV light/visible light to be activated. Therefore the development of photocatalytic agents that can be activated by NIR light which has high occupation of the solar spectrum is more desirable.26 Previously, up-conversion nanoparticles have been reported for NIR light mediated photocatalytic activity; however it took a long time for dye degradation to occur.27 Therefore, there is much need to develop new NIR mediated photocatalytic agents with lower degradation times and good water solubilities.
In recent times, among various transition metal chalcogenides bismuth selenide (Bi2Se3) NMs28,29 have been explored in biomedical applications such as bioimaging,30 radiation therapy,31 and PTT32 of cancer. However, the photothermal bacterial killing ability of Bi2Se3 NMs has not been explored yet. In addition, the NIR light mediated photodynamic properties and photocatalytic properties of Bi2Se3 NMs have not been explored to date.
It is well known that bacterial cells are negatively charged due to the high proportion of anionic phospholipids in their cell walls; this leads to the strong affinity between bacteria and positively charged surfaces through electrostatic interaction. Previously, it has been reported that materials of which the surfaces were engineered with cationic polyethyleneimine (PEI) exhibited a bacteria targeting ability towards both Gram negative (E. coli) and Gram positive bacteria (S. aureus) through electrostatic interaction and enhanced antibacterial efficacy.33 In addition, surface engineering with PEI provides good dispersion of materials in polar solvents such as water and buffers.34–36
Herein, we report the synthesis and application of PEI-wrapped Bi2Se3 nanoplates (Bi2Se3 NPs/PEI) as a novel bacteria targeting dual-modal phototherapeutic agent for combined PTT and PDT mediated bacterial eradication as well as a novel NIR mediated photocatalytic agent (Scheme 1). In this study, the engineering of Bi2Se3 NPs with PEI conferred the NPs with bacteria targeting ability through electrostatic interaction along with good water dispersion. This Bi2Se3 NPs/PEI material exhibited high antibacterial efficacy through combined PTT and PDT upon a single irradiation with an 808 nm laser. In addition, the developed Bi2Se3 NPs/PEI also demonstrated excellent NIR light mediated photocatalytic activity for degradation of methylene blue (MB) and rhodamine B (RhB). To the best of our knowledge, this is the first report exploring the NIR light mediated antimicrobial properties, photodynamic properties and photocatalytic activity of Bi2Se3 NPs.
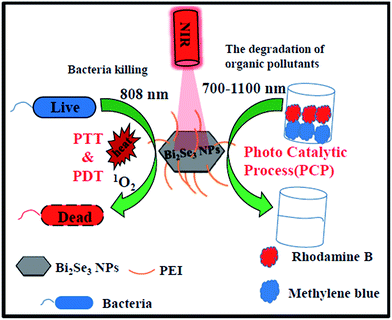 |
| Scheme 1 Schematic for NIR light mediated antibacterial and photocatalytic activity of Bi2Se3 NPs/PEI. | |
Experimental section
Synthesis
Bi2Se3 NPs were prepared according to the previously reported method with slight modifications.37 Briefly, appropriate amounts of Bi(NO3)3 (1 mM) and Na2SeO3 (1.5 mM) were mixed and dissolved in ethylene glycol (35 mL) with vigorous stirring for 1 h. The mixed solution was subsequently refluxed at 260 °C for 5 h. Finally, the reaction mixture was cooled to room temperature and isopropyl alcohol was added to precipitate the Bi2Se3 NPs. The precipitate was washed with acetone and DI water several times, to remove any unreacted chemicals as well as ethylene glycol, and was further dried in an oven for 6 h at 50 °C. To prepare the aqueous solution containing dispersed Bi2Se3 NPs/PEI, as-prepared Bi2Se3 NPs (50 mg) was dissolved in DI water (50 mL) by sonication for 10 min. PEI (20 μL) was slowly added. The solution was subjected to continuous stirring for 24 h at room temperature. The obtained Bi2Se3 NPs/PEI was washed several times with DI water to remove unbound PEI.
NIR light mediated photothermal effect
Different concentrations (10, 30, 50, and 80 ppm) of Bi2Se3 NPs/PEI and Bi2Se3 NPs (80 ppm) were dispersed in PBS solution followed by irradiation with an 808 nm laser at 1 W cm−2 for 10 min. To investigate the effect of power density, a Bi2Se3 NPs/PEI solution (80 ppm) was irradiated at different laser power densities (0.5, 1, and 1.5 W cm−2). The solution temperature was recorded every 2 min using a thermocouple to monitor the temperature change. The calculation of photothermal conversion efficiency was detailed in the ESI.†
NIR light mediated singlet oxygen generation
The generation of 1O2 in the presence of Bi2Se3 NPs or Bi2Se3 NPs/PEI upon laser irradiation was measured by recording the absorbance change of DPBF.18 In a typical experiment, appropriate amounts of Bi2Se3 NPs or Bi2Se3 NPs/PEI (1 mg mL−1) and DPBF (0.08 mM) were mixed in ethanol (2 mL) contained in a quartz cuvette followed by irradiation with an 808 nm laser at 1 W cm−2. The DPBF (λabs = 410 nm) readily reacted with 1O2 and formed a bleached adduct which caused a decrease in absorbance. The change in absorbance of the solution at 410 nm upon laser irradiation was recorded every 2 min over a period of 12 min using a UV-vis spectrophotometer.
The generation of 1O2 in the bacterial solution (both E. coli and S. aureus) was tested using DCFH-DA and a confocal fluorescence assay. In this experiment, DCFH-DA (10 μL) was added to a bacterial solution containing Bi2Se3 NPs/PEI (80 ppm). The solution was irradiated with an 808 nm laser for 10 min and visualized under a CLSM confocal microscope (Zeiss, LSM 700). A blue colour indicated the presence of bacteria and a green colour indicated the generation of 1O2 in the bacteria solution.
NIR light mediated antibacterial activity
The antibacterial activity of the as-prepared Bi2Se3 NPs and Bi2Se3 NPs/PEI was evaluated using Gram-positive S. aureus (BCRC 11863) and Gram-negative E. coli (BCRC 11509) as test organisms. All glassware and required equipment was sterilized in an autoclave at 120 °C before microbiological experiments. S. aureus and E. coli cultures were grown on nutrient agar for 24 h, then transferred into an Erlenmeyer flask containing nutrient broth (Scharlau Chemie, Spain) at an initial optical density at 600 nm (OD 600) of 0.1, and allowed to grow at 37 °C for 16 h. The bacteria were spun down by centrifugation and the resultant pellet was washed twice with a saline solution (0.9%) to yield a final bacterial concentration of ∼106 to 107 CFU mL−1. Different concentrations of Bi2Se3 NPs/PEI (10, 30, 50, and 80 ppm) were incubated at 37 °C with bacterial solution (1 mL) in separate vials for 30 min. The bacteria along with the captured Bi2Se3 NPs (80 ppm) or Bi2Se3 NPs/PEI were spun down by centrifugation and the supernatant containing free Bi2Se3 NPs/PEI was discarded. The bacteria–Bi2Se3 NPs or –Bi2Se3 NPs/PEI pellet was dispersed in PBS and irradiated with an 808 nm laser light (1 W cm−2) for 10 min. The distance was maintained at 7 cm between the bacterial solution and the laser source. An appropriate aliquot of the suspension (100 μL) was collected, and diluted about 50 times. An appropriate amount of the diluted solution (100 μL) was plated on an agar plate and incubated at 37 °C for 24 h before the bacteria colonies were counted.
Electron microscopy measurement
Scanning electron microscopy (SEM) was performed to investigate the morphological changes of E. coli and S. aureus upon laser irradiation by following a reported procedure.10 The bacteria were incubated with Bi2Se3 NPs/PEI (80 ppm) and irradiated for 10 min using an 808 nm laser. After irradiation the control and irradiated bacteria were centrifuged and the supernatant was discarded. The bacterial pellet was dispersed and fixed with formaldehyde (4%) for 30 min, then dehydrated and sputter coated with platinum, ready for SEM imaging.
Fluorescence assay
After irradiation, the control and irradiated bacteria were stained with PI (10 μg mL−1) for 10 min and stained with DAPI (3 μg mL−1) for 5 min in the dark. The live and dead bacteria were visualized with a CLSM confocal microscope (Zeiss, LSM 700).
Assessment of photocatalytic activity
The photocatalytic activity of the as-prepared Bi2Se3 NPs/PEI was evaluated by the degradation of organic pollutants, MB and RhB dyes, under NIR light irradiation. The aqueous stock solutions of MB and RhB (10 μg mL−1) in DI water were prepared. Then, Bi2Se3 NPs/PEI (5 mg) was added to the MB and RhB solutions (20 mL) and the suspensions were sonicated for 5 min, followed by stirring for 30 min in a dark environment to allow equilibrium of adsorption/desorption. The obtained suspensions were examined under NIR light irradiation (300 W high pressure halogen lamp, 700–1000 nm wavelength region). In this experiment the 808 nm laser was replaced by a halogen lamp because the former had a small laser spot which cannot be expanded, leading to experimental difficulties in covering the whole area of the vessel (20 mL contents with dyes and Bi2Se3 NPs/PEI). The average temperature during the experiments was maintained at 30 °C. Total irradiation time was 3 h and 4 h for MB and RhB, respectively. At every 30 min interval, a suspension solution (2.5 mL) was taken from the reacting suspension, centrifuged, and the UV-vis absorbance spectrum was recorded (Model Lambda 35, Perkin-Elmer). The as-prepared Bi2Se3 NPs/PEI was recovered from the suspension by centrifugation at 6000 rpm for 4 min and reused to determine the efficiency. The same experiments were carried out by adding 10 μg mL−1 of each organic dye into a tap water sample. The percentage of dye degradation was calculated by the following formula: |
Dye degradation (%) = [(C0 − Ct/C0)] × 100
| (1) |
where C0 is the initial concentration of the MB and RhB solutions and Ct is the concentration of the dye solution after 30 min of NIR light irradiation.
The photocatalytic stability test
A photocatalytic stability of Bi2Se3 NPs/PEI experiment was performed. Herein, after completion of the photocatalytic reaction, the photocatalyst was centrifuged. The residue was collected and subsequently washed with DI water and ethanol, and dried in an oven at 60 °C. The recycled photocatalyst was reused for a second cycle of the degradation study with fresh MB and RhB solutions. This process was repeated 3 times.
Results and discussion
Characterization
The morphologies and nanostructures of the as-prepared Bi2Se3 NPs were investigated by scanning electron microscopy (SEM) and transmission electron microscopy (TEM). The SEM images (Fig. 1a and b) depicted the layered structure of the Bi2Se3 NPs with an average size within 0.8–0.9 μm. The NPs appeared with good hexagonal morphology with a planar dimension, and some were formed in an intermediate stage and showed truncated trigonal morphology. The curves and outlines of overlap between two nanoplates in the image could be clearly distinguished. The hexagonal morphology of the Bi2Se3 NPs was further confirmed by low magnification bright field TEM (Fig. 1c), and Bi2Se3 NPs form a layered chalcogenide with a rhombohedral crystal structure (inset in Fig. 1b).
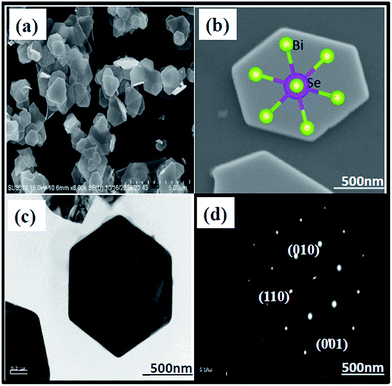 |
| Fig. 1 Images of Bi2Se3 NPs: (a) SEM image under low magnification, (b) high magnification (inset demonstrating the rhombohedral crystal structure), (c) TEM image, and (d) SAED image. | |
The selected area electron diffraction (SAED) pattern (Fig. 1d) can be indexed to have 6-fold symmetry with a [001] zone axis pattern, which is in good agreement with a previous report.38 Energy dispersive X-ray analysis (EDXA) was performed to determine the chemical composition of the Bi2Se3 NPs. The EDXA mapping (Fig. S1a–c†) confirmed the presence of Bi and Se elements with an approximate atomic ratio of 2
:
3. The EDXA spectrum (Fig. S1d†) also confirmed the presence of Bi with peaks at 1.9 and 2.52 keV, and Se with a peak at 1.47 keV. Additionally, the powder XRD pattern (Fig. 2a) correlated well with the standard Bi2Se3 pattern (JCPDS no. 89-2008) and exhibited characteristic diffraction peaks at 2θ = 30.1°, 41.3°, 44.5°, 48.7°, 67.6°, and 79.7°, which can be indexed to the (101), (015), (1010), (110), (0015), (1115), and (222) planes of Bi2Se3 NPs, respectively. These results were in accordance with a previous report.37
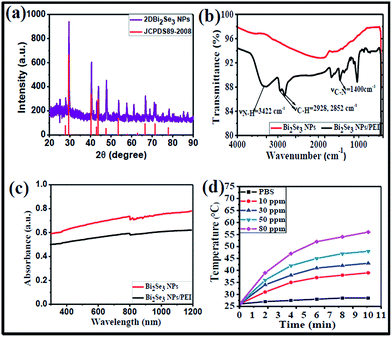 |
| Fig. 2 The spectra of Bi2Se3 NPs: (a) powder XRD, (b) FTIR, (c) UV-vis-NIR absorption, and (d) temperature profiles of Bi2Se3 NPs/PEI at different concentrations upon exposure to 808 nm NIR light at 1 W cm−2. | |
In order to achieve good water dispersibility, the surface of Bi2Se3 NPs was wrapped with a cationic polymer, polyethyleneimine (PEI), via electrostatic interactions. The successful surface modification was confirmed by Fourier transform infrared (FTIR) spectra (Fig. 2b). The Bi2Se3 NPs/PEI exhibited bands at 3422, 2928, and 2852 cm−1, which are attributed to N–H, C–H, and C–H vibrational frequencies, respectively; whereas Bi2Se3 NPs did not show any characteristic vibrational frequencies. In addition, the presence of the C–N stretching frequency at 1400 cm−1 revealed the existence of abundant amine groups in the Bi2Se3 NPs/PEI.39 Furthermore, zeta-potential measurements (Fig. S2a†) indicated that the negatively charged surface of Bi2Se3 NPs (−33.2 ± 2.56 mV) is converted to a positively charged surface (35 ± 2.56 mV) after wrapping with PEI. These results evidence the successful wrapping of Bi2Se3 NPs with PEI. The as-prepared Bi2Se3 NPs/PEI exhibited good dispersibility in water as well as in phosphate buffered solution (PBS) (Fig. S2b(i–iv)†). The optical properties of Bi2Se3 NPs and Bi2Se3 NPs/PEI were investigated with UV-vis-NIR spectra ranging from 300–1200 nm (Fig. 2c), both exhibiting high absorbance in the range of interest, from 700 to 1200 nm, supporting their potential NIR light-induced properties.
NIR light mediated properties
Owing to the high absorbance in the NIR region, the photothermal properties of Bi2Se3 NPs and different concentrations of Bi2Se3 NPs/PEI were investigated upon irradiation with an 808 nm laser at 1 W cm−2 for 10 min. The temperature elevation profile of both Bi2Se3 NPs and Bi2Se3 NPs/PEI (Fig. S3a†) exhibited high and concentration dependent photothermal effects. Detailed temperature profiles of Bi2Se3 NPs/PEI at different concentrations (Fig. 2d) showed that the temperature was elevated with increasing Bi2Se3 NPs/PEI concentration and the highest tested concentration of 80 ppm elevated the temperature to 56 °C. This temperature is sufficient enough to cause the ablation of bacteria by inhibiting essential intracellular reactions and denaturing proteins/enzymes.10,12 Furthermore, the temperature profiles of 80 ppm Bi2Se3 NPs/PEI irradiated with an 808 nm laser at (0.5, 1.0 and 1.5) W cm−2 for 10 min (Fig. S3b†) also revealed that the temperature elevates with increased power density. In addition, the photothermal conversion efficiency (η) of Bi2Se3 NPs/PEI was calculated as 32.07%. The results indicated that Bi2Se3 NPs/PEI can be effectively used as a photothermal therapy reagent.
Some metal chalcogenide nanoparticles have been reported to function as photothermal agents and generate singlet oxygen (1O2) upon laser irradiation and act as PDT agents.17 We therefore investigated the 1O2 generation ability of Bi2Se3 NPs/PEI upon irradiation by an 808 nm laser by measuring the change in the absorbance of 1,3-diphenylisobenzofuran (DPBF). DPBF is known to bleach via an oxidation reaction with 1O2 to form an endoperoxide product, and thus can be used as an indicator for 1O2 generation.18 The change in the absorbance of DPBF as a function of irradiation time in the presence/absence of NIR light was shown in Fig. S5.† The results reveals that in the absence of light there is no significant change in the absorbance of DPBF (Fig. S5a and c†). However, in the presence of light both samples containing Bi2Se3 NPs/PEI and Bi2Se3 NPs exhibited a steady drop in absorbance of DPBF at 410 nm with increased irradiation time (Fig. S5b and c†), indicating that the bleaching was caused by 1O2. These results confirm the 1O2 generation ability of both Bi2Se3 NPs and Bi2Se3 NPs/PEI.
However, the 1O2 generation efficiency of Bi2Se3 NPs was slightly less than that of Bi2Se3 NPs/PEI (Fig. S5c†), and was attributed to the poor water solubility of Bi2Se3 NPs. These results indicate that Bi2Se3 NPs/PEI has potential for use in PDT. The potential of the 1O2 generation ability of Bi2Se3 NPs/PEI was further tested with bacteria solutions. The 2,7-dichlorofluorescein diacetate (DCFH-DA) fluorescent probe was used to test for the presence of 1O2 inside bacteria. When the bacterial solution was irradiated by an 808 nm laser, some green fluorescence of DCFH-DA was observed (Fig. S6a and b†), indicating that Bi2Se3 NPs/PEI can generate ROS upon irradiation by an 808 nm laser. ROS can destroy the cytoplasmic membrane and lead to DNA destruction, resulting in effective killing of bacteria. To the best of our knowledge, this is the first demonstration of the ROS generation ability of Bi2Se3 NPs upon NIR light excitation.
NIR light mediated dual-modal antibacterial activity
Inspired by the photoirradiation results, we evaluated the effect of photoirradiation in the use of PTT and PDT together in the killing of S. aureus and E. coli, which were first incubated with different concentrations of Bi2Se3 NPs/PEI for 30 min followed by photoirradiation using an 808 nm laser for 10 min. The photoirradiation killing effect on the bacteria was determined by a colony counting method on agar plates after incubation for 24 h. The photoirradiation killing effect (Fig. 3 and S4†) was dependent on both the Bi2Se3 NPs/PEI concentrations and laser power intensities. The killing efficiency was directly proportional to the concentration of Bi2Se3 NPs/PEI and more than 97% of both S. aureus and E. coli were killed at 80 ppm which was attributed to the strong PTT effect and 1O2 generation ability. The absence of either the laser or the Bi2Se3 NPs/PEI did not cause significant bacteria death, proving that the observed bacterial killing proceeds via the photothermal effect and the generation of 1O2.
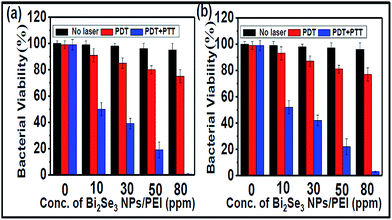 |
| Fig. 3 Bacterial viability in percentage under PDT alone and PTT + PDT for (a) S. aureus and (b) E. coli in comparison to the control (no laser). | |
The extent of the respective and combined effect of PDT and PTT on bacterial death was evaluated. An irradiation experiment was performed at 4 °C (to minimize the PTT effect) to estimate the contribution from PDT alone under the assumption that the PDT effect is independent of the incubation temperature. A series of bacterial solutions containing different concentrations of Bi2Se3 NPs/PEI ranging from 10 to 80 ppm were prepared and tested for their bacterial killing effects. Using the bacteria plate count method without the laser, with the laser at 4 °C and with the laser at room temperature conditions, the contributions from the laser, PDT, and PDT + PTT were evaluated for their effect on bacteria death respectively. The obtained results indicated that the killing efficiency of PDT alone was ∼21% and ∼19% for S. aureus (Fig. 3a) and E. coli (Fig. 3b), respectively. In addition, the killing efficiency of PTT alone was obtained from the difference between the killing efficiency of PTT + PDT and PDT alone, which was ∼78% and ∼76% for S. aureus and E. coli, respectively.
To further verify the bacterial survival rate upon laser irradiation, a fluorescence-based bacterial viability assay10 was conducted. Briefly, propidium iodide (PI) was used for the labelling of dead bacteria with red fluorescence; whereas 4,6-diamidino 2-phenylindole (DAPI) was used for live bacteria labelling with blue fluorescence. The fluorescence images of S. aureus (Fig. 4a) and E. coli (Fig. 4c) clearly evidence an abundant number of DAPI-labelled live bacteria in the absence of PTT + PDT. Meanwhile, PI-labelled dead bacteria became more obvious in the Bi2Se3 NPs/PEI solution (Fig. 4b and d) after 10 minutes of irradiation by an 808 nm laser. SEM images were also taken to evaluate the morphological changes of both S. aureus and E. coli before and after treatment with Bi2Se3 NPs/PEI. The control S. aureus and E. coli retained a complete and undamaged round-shape (Fig. 5a) and rod-shape (Fig. 5c) structure, respectively. However, the Bi2Se3 NPs/PEI + NIR treated bacteria (Fig. 5b and d) both exhibited a damaged and groove-like structure. The excellent capturing capability towards both bacteria walls of Bi2Se3 NPs/PEI is presumably due to the positive charge of PEI. In combination with the improved PDT and PTT effects upon NIR light irradiation, the obtained results show that the Bi2Se3 NPs/PEI material acts as a promising PDT + PTT agent in comparison to some other reported materials (Table S1†).
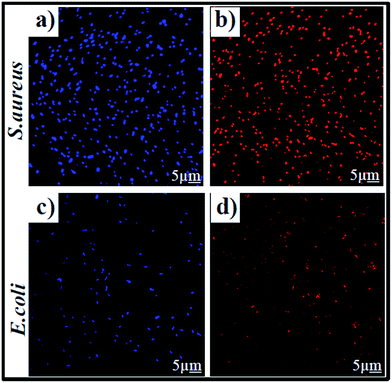 |
| Fig. 4 Fluorescence microscopy (confocal) images showing the PTT and PDT cytotoxicity for S. aureus and E. coli after 10 minutes of irradiation by an 808 nm laser at 1 W cm−2 with the Bi2Se3 NPs/PEI control (a, c) and the combined PTT and PDT effect on the bacteria (b, d). | |
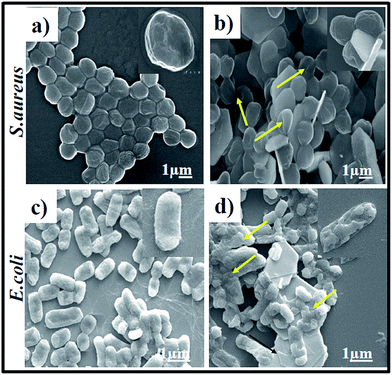 |
| Fig. 5 SEM images of S. aureus ((a) control, (b) Bi2Se3 NPs/PEI + NIR); E. coli ((c) control, (d) Bi2Se3 NPs/PEI + NIR) (conc. of Bi2Se3 NPs/PEI = 80 ppm, 808 nm laser, 1 W cm−2, 10 min). The yellow arrow highlights the damaged and groove-like structures. | |
Photocatalytic degradation of MB and RhB
The photocatalytic activities of Bi2Se3 NPs and Bi2Se3 NPs/PEI were investigated based on the degradation of model organic pollutants such as MB and RhB dyes under NIR light irradiation. The photodegradation activities were monitored by measuring the absorbances at the characteristic wavelengths of ∼664 nm and ∼540 nm for MB and RhB, respectively. A comparison study between the control group (i.e. dyes with Bi2Se3 NPs/PEI in the dark) and experimental groups (i.e. dyes with NIR light + Bi2Se3 NPs/PEI) was performed based on the respective degradation rates. The obtained time-evolved UV-vis spectra of MB and RhB demonstrate that Bi2Se3 NPs/PEI in the dark did not induce any significant changes in the absorbances of MB or RhB (Fig. S7a and c†). However, for the groups treated with NIR light decreases in the absorbances of MB at 664 nm and RhB at 540 nm with increasing irradiation time were observed (Fig. S7b and d†), demonstrating the photocatalytic activity of Bi2Se3 NPs/PEI on MB and RhB. To estimate the residual concentrations of MB and RhB dyes after photodegradation, the C/C0 ratio was plotted vs. the irradiation time, where C and C0 are the concentrations of the dyes at a time t and t = 0, respectively. The results reveal that the decrease in concentration of both MB (Fig. 6a) and RhB (Fig. 6b) is negligible when treated with Bi2Se3 NPs/PEI in the dark. However, when treated with NIR + Bi2Se3 NPs or NIR + Bi2Se3 NPs/PEI, the degradation of both MB and RhB increases significantly with increasing irradiation time. Degradation rates of ∼95% for MB and ∼93% for RhB were found after 3 h and 4 h, respectively. Both solutions became faded. The kinetic data for photodegradation of MB and RhB in the presence of Bi2Se3 NPs and Bi2Se3 NPs/PEI were investigated corresponding to the Langmuir–Hinshelwood model.40 The degradation kinetics data were fitted with a pseudo-first order kinetic equation, where C0 and Ct are the initial concentrations of MB and RhB before irradiation and at any time t under light irradiation respectively, and k is the apparent rate constant (min−1). The regression curves of ln(C0/Ct) vs. t were straight lines, indicating pseudo-first order kinetics, which can describe the photocatalytic degradation of MB and RhB (Fig. 6c and d). Nevertheless, the Bi2Se3 NPs exhibited slightly less photocatalytic activity than Bi2Se3 NPs/PEI, ascribed to its poor water solubility.
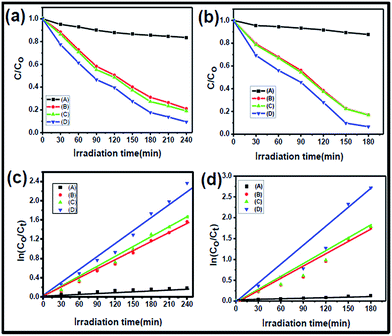 |
| Fig. 6 (a) Dye + Bi2Se3 NPs/PEI, (b) dye + Bi2Se3 NPs + NIR light, (c) dye + Bi2Se3 NPs/PEI + NIR light, and (d) dye + Bi2Se3 NPs/PEI. All in DI water except (c) in tap water; photocatalytic degradation of (A) MB and (B) RhB. Reaction kinetics for (C) MB and (D) RhB. | |
Furthermore, to investigate the ability of the Bi2Se3 NPs/PEI nanocomposites for removal of organic dyes from water, we added 10 μg mL−1 of each organic dye into tap water samples. The effect of the presence of common anions such as sulfate, carbonate, and bicarbonate on the photocatalytic degradation of organic dyes is shown in Fig. 6c. It is observed that the rate of degradation decreases in the presence of anions, which may be due to inhibition of hydroxyl radicals.41 These anions may hinder the active sites on the Bi2Se3 NPs surface.
The schematic of the mechanism for the photodegradation of MB and RhB with NIR + Bi2Se3 NPs/PEI (Fig. S8†) illustrates that when a mixed solution of the photocatalyst and dyes is irradiated with NIR light with 700–1000 nm wavelength, the e− in the VB is excited to the CB of the Bi2Se3 NPs/PEI. The excited e− will react with the adsorbed O2 molecules and form ·O2− radicals.35 Alternatively, the h+ in the VB of the Bi2Se3 NPs are taken by OH− derived from the aqueous solution and form ·OH radicals in the mixed solution. These radicals, O2− and ·OH, act as oxidizing agents and react with both dye molecules and decompose them into CO2, H2O, and other small molecules,42–45 avoiding the recombination of h+ and e− during the photocatalytic process. The complete degradation process of MB and RhB in the presence of Bi2Se3 NPs/PEI as a photocatalyst may be explained with the help of the following equations:
|
Bi2Se3 NPs/PEI + photons (hν, 700–1100 nm) → (e−) CB + (h+) VB
| (3) |
|
OH− + h+ → ˙OH (radical)
| (5) |
|
O2 + e− → O2−˙ (radical)
| (6) |
MB/RhB + (Bi2Se3 NPs/PEI) + O2−˙ → degradation products was monitored at 660 to 664 nm and 540 nm, respectively. MB/RhB + (Bi2Se3 NPs/PEI) + ˙OH → degradation products was monitored at 660 to 664 nm and 540 nm, respectively.
In addition to the photocatalytic activity, the stability of photocatalysts is also very important for practical applications. Reusability was evaluated by using a recycling test of the photocatalytic degradation of MB and RhB in four repeated cycles using Bi2Se3 NPs and Bi2Se3 NPs/PEI under identical experimental conditions. The results showed that the degradation efficiency for Bi2Se3 NPs/PEI was ∼91 and ∼89% for MB and RhB, respectively, after four cycles which was ∼10% better than that of the Bi2Se3 NPs (Fig. S9†), and this suggests that the prepared Bi2Se3 NPs/PEI possessed good activity and stability. Further, the SEM indicated that there were no observable morphological changes in the recycled photocatalyst (Fig. S10†). Moreover, the photodegradation efficiency of the recycled photocatalysts were investigated and it was observed that there were no significant changes (Fig. S9†). Good photocatalytic activity in the degradation of dyes with remarkable stability demonstrates that Bi2Se3 NPs/PEI could act as a reliable photocatalyst in comparison to other reported materials (Table S2†). To the best of our knowledge, this is the first demonstration of the NIR light mediated photocatalytic ability of Bi2Se3 NPs.
Conclusions
In summary, we have engineered a novel material, Bi2Se3 NPs/PEI, that can be used as a NIR light activated dual-modal phototherapeutic agent for eradication of pathogenic bacteria as well as for photodegradation of organic pollutants. The developed Bi2Se3 NPs/PEI exhibited good hexagonal morphology with a planar dimension. Also, the Bi2Se3 NPs/PEI demonstrated excellent absorption characteristics in the NIR wavelength region. The photothermal conversion efficiency of Bi2Se3 NPs/PEI was calculated as 32.07%. The Bi2Se3 NPs/PEI also generated singlet oxygen upon NIR laser irradiation. Furthermore, the dual-modal phototherapeutic effects mediated by Bi2Se3 NPs/PEI induced high percentages of bacterial cellular deaths for both E. coli (97% at 80 ppm) and S. aureus (99% at 80 ppm). The relative contributions of PTT and PDT were found to be near to a 4
:
1 ratio in killing both bacteria. To the best of our knowledge, this is the first report that demonstrates the photodynamic therapeutic abilities of Bi2Se3 NPs/PEI in killing pathogenic bacteria. In addition, Bi2Se3 NPs/PEI also showed good NIR light activated photocatalytic properties for the degradation of organic pollutants such as MB and RhB. The degradation efficiencies were ∼95% for MB and ∼90% for RhB after 3 h and 4 h, respectively. Overall, the novel Bi2Se3 NPs/PEI material is a promising antibacterial agent and good photocatalyst for bacterial infections and drinking water purification. Finally, the developed Bi2Se3 NPs/PEI preferentially adsorbed cationic dyes via strong electrostatic interactions. Future expansion of its application to anionic dyes like methyl orange and Congo red, and the possible drop in adsorption efficiencies and the degradation efficiencies for these dyes must be considered.
Conflicts of interest
There are no conflicts to declare.
Acknowledgements
We are grateful for the financial support of the Ministry of Science and Technology, Taiwan (MOST 104-2113-M-007-008-MY3 and MOST 104-2923-M-007-002-MY3) and the National Tsing Hua University for this work.
References
- Y. Tsai, P. Cheng and T. Pan, J. Agric. Food Chem., 2010, 58, 1207–1212 Search PubMed.
- Y. W. Wang, Y. Y. Fu, L. J. Wu, J. Li, H. H. Yang and G. N. Chen, J. Mater. Chem. B, 2013, 1, 2496–2501 RSC.
- C. J. Jeong, S. M. Sharker, I. In and S. Y. Park, ACS Appl. Mater. Interfaces, 2015, 7, 9469–9478 Search PubMed.
- S. Chen, F. Tang, L. Tang and L. Li, ACS Appl. Mater. Interfaces, 2017, 9, 20895–20903 Search PubMed.
- Y. Jin, J. Deng, J. Yu, C. Yang, M. Tong and Y. Hou, J. Mater. Chem. B, 2015, 3, 3993–4000 RSC.
- X. Liu, G. Yang, L. Zhang, Z. Cheng and X. Zhu, Nanoscale, 2016, 8, 15323–15339 RSC.
- S. Wang, A. Riedinger, H. Li, C. Fu, H. Liu, L. Li, T. Liu, L. Tan, M. J. Barthel, G. Pugliese, F. De Donato, M. S. D’Abbusco, X. Meng, L. Manna, H. Meng and T. Pellegrino, ACS Nano, 2015, 9, 1788–1800 CrossRef PubMed.
- T. J. Ondera and A. T. Hamme, J. Mater. Chem. B, 2014, 2, 7534–7543 RSC.
- A. Gharatape, M. Milani, S. H. Rasta, M. P. Moghaddam, S. A. Kandjani, S. Davaran and R. Salehi, RSC Adv., 2016, 6, 110499–110510 RSC.
- M. C. Wu, A. R. Deokar, J. H. Liao, P. Y. Shih and Y. C. Ling, ACS Nano, 2013, 7, 1281–1290 CrossRef PubMed.
- M. Sinha, G. Gollavelli and Y. C. Ling, RSC Adv., 2016, 6, 63859–63866 RSC.
- C. Korupalli, C. C. Huang, W. C. Lin, W. Y. Pan, P. Y. Lin, W. L. Wan, M. J. Li, Y. Cang and H. W. Sung, Biomaterials, 2017, 116, 1–9 CrossRef PubMed.
- D. R. Rice, H. Gan and B. D. Smith, Photochem. Photobiol. Sci., 2015, 14, 1271–1281 Search PubMed.
- Z. Lim, J. L. Cheng, T. W. Lim, E. G. Teo, J. Wong, S. George and A. Kishen, Aust. Dent. J., 2009, 54, 108–114 CrossRef PubMed.
- B. Z. Ristic, M. M. Milenkovic, I. R. Dakic, B. M. T. Markovic, M. S. Milosavljevic, M. D. Budimir, V. G. Paunovic, M. D. Dramicanin, Z. M. Markovic and V. S. Trajkovic, Biomaterials, 2014, 35, 4428–4435 CrossRef PubMed.
- J. A. R. Herrera, J. Sanabria, F. Machuca, C. F. Dierolf, C. Pulgarin and G. Orellana, J. Sol. Energy Eng., 2007, 129, 135–140 CrossRef.
- P. Kalluru, R. Vankayala, C. S. Chiang and K. C. Hwang, Angew. Chem., Int. Ed., 2013, 52, 12332–12336 CrossRef PubMed.
- G. Gollavelli and Y. C. Ling, Biomaterials, 2014, 35, 4499–4507 CrossRef PubMed.
- X. Huang, G. Chen, J. Pan, X. Chen, N. Huang, X. Wang and J. Liu, J. Mater. Chem. B, 2016, 4, 6258–6270 RSC.
- H. Kusic, N. Koprivanac and A. L. Bozic, J. Photochem. Photobiol., A, 2013, 252, 131–144 CrossRef.
- X. Zhong, Z. Dai, F. Qin, J. Li, H. Yang, Z. Lu, Y. Liang and R. Chen, RSC Adv., 2015, 5, 69312–69318 RSC.
- P. Ju, Y. Wang, Y. Sun and D. Zhang, Dalton Trans., 2016, 45, 4588–4602 RSC.
- A. Salabat and F. Mirhoseini, Photochem. Photobiol. Sci., 2015, 14, 1637–1643 Search PubMed.
- A. Y. Booshehri, S. C. K. Goh, J. Hong, R. Jiang and R. Xu, J. Mater. Chem. A, 2014, 2, 6209–6217 Search PubMed.
- Y. Xu, S. Huang, H. Ji, L. Jing, M. He, H. Xu, Q. Zhang and H. Li, RSC Adv., 2016, 6, 6905–6914 RSC.
- W. P. Qin, D. S. Zhang, D. Zhao, L. L. Wang and K. Z. Zheng, Chem. Commun., 2010, 46, 2304–2306 RSC.
- Y. Tang, W. Di, X. Zhai, R. Yang and W. Qin, ACS Catal., 2013, 3, 405–412 CrossRef.
- S. D. Yang, L. Yang, Y. X. Zheng, W. J. Zhou, M. Y. Gao, S. Y. Wang, R. J. Zhang and L. Y. Chen, ACS Appl. Mater. Interfaces, 2017, 9, 29295–29301 Search PubMed.
- B. Das, N. S. Das, S. Sarkar, B. K. Chatterjee and K. K. Chattopadhyay, ACS Appl. Mater. Interfaces, 2017, 9, 22788–22798 Search PubMed.
- X. D. Zhang, J. Chen, Y. Min, G. B. Park, X. Shen, S. S. Song, Y. M. Sun, H. Wang, W. Long, J. Xie, K. Gao, L. Zhang, S. Fan, F. Fan and U. Jeong, Adv. Funct. Mater., 2014, 24, 1718–1729 CrossRef.
- G. Song, C. Liang, X. Yi, Q. Zhao, L. Cheng, K. Yang and Z. Liu, Adv. Mater., 2016, 28, 2716–2723 CrossRef PubMed.
- H. Zhang, C. X. Liu, X. L. Qi, X. Dai, Z. Fang and S. C. Zhang, Nat. Phys., 2009, 5, 438–442 CrossRef.
- K. A. Gibney, I. Sovadinova, A. I. Lopez, M. Urban, Z. Ridgway, G. A. Caputo and K. Kuroda, Macromol. Biosci., 2012, 12, 1279–1289 CrossRef PubMed.
- J. S. M. Nithya and A. Pandurangan, RSC Adv., 2014, 4, 32031–32046 RSC.
- S. Taranejoo, J. Liu, P. Verma and K. Hourigan, J. Appl. Polym. Sci., 2015, 42096, 1–8 Search PubMed.
- M. Jager, S. Schubert, S. Ochrimenko, D. Fischer and U. S. Schubert, Chem. Soc. Rev., 2012, 41, 4755–4767 RSC.
- C. Dun, C. A. Hewitt, H. Huang, J. Xu, D. S. Montgomery, W. Nie, Q. Jiang and D. L. Carroll, ACS Appl. Mater. Interfaces, 2015, 7, 7054–7059 Search PubMed.
- J. Zhang, Z. Peng, A. Soni, Y. Zhao, Y. Xiong, B. Peng, J. Wang, M. S. Dresselhaus and Q. Xiong, Nano Lett., 2011, 11, 2407–2414 CrossRef PubMed.
- S. Subramanian and D. P. Padiyan, Mater. Chem. Phys., 2008, 107, 392–398 CrossRef.
- B. F. Luo, D. G. Xu, D. Li, G. L. Wu, M. M. Wu, W. D. Shi and M. Chen, ACS Appl. Mater. Interfaces, 2015, 7, 17061–17069 Search PubMed.
- O. Legrini, E. Oliveros and A. M. Braun, Chem. Rev., 1993, 93, 671–698 CrossRef.
- L. Liu, J. Liu and D. D. Sun, Catal. Sci. Technol., 2012, 2, 2525–2532 Search PubMed.
- Y. Xu, S. Huang, H. Ji, L. Jing, M. He, H. Xu, Q. Zhang and H. Li, RSC Adv., 2016, 6, 6905–6914 RSC.
- S. G. Kumar and K. S. R. Koteswara Rao, RSC Adv., 2015, 5, 3306–3351 RSC.
- C. Sushma and S. G. Kumar, Inorg. Chem. Front., 2017, 4, 1250–1267 RSC.
Footnote |
† Electronic supplementary information (ESI) available. See DOI: 10.1039/c8ra02183j |
|
This journal is © The Royal Society of Chemistry 2018 |
Click here to see how this site uses Cookies. View our privacy policy here.