DOI:
10.1039/C8RA01995A
(Paper)
RSC Adv., 2018,
8, 20603-20611
Treatment of dinitrodiazophenol industrial wastewater in heat-activated persulfate system†
Received
6th March 2018
, Accepted 11th May 2018
First published on 5th June 2018
Abstract
Heat-activated persulfate oxidation process was investigated as the treatment of dinitrodiazophenol industrial wastewater to degrade refractory pollutants and improve biodegradability. By studying the effects of 4 factors and carrying out orthogonal tests and scale-up experiments, optimal treatment conditions (temperature 90 °C, reaction time 75 min, PS dosage 20.0 g L−1 and initial pH ∼2.0) were obtained. The results showed that under these conditions, COD and color removal efficiencies were 99.22% and 99.99%, respectively. Moreover, an increase in BOD5/COD ratio (from 0 to 0.31) indicates significantly improved biodegradability. Dinitrodiazophenol dosage was measured by high performance liquid chromatography, which showed that dinitrodiazophenol removal efficiency reached 99.99%. Furthermore, the degradation process was analyzed by ultraviolet-visible spectra and Fourier transform infrared spectra. The former demonstrated that aromatic compounds in the system were destroyed during mineralization and the latter indicated that nitro groups on the benzene ring could be oxidized to nitrate. After verification test of the free radicals, mechanism of heat-activated persulfate system was assumed to be that SO4˙− and ·OH function together and SO4˙− predominate. To conclude, the heat-activated PS oxidation technology performs effectively in treatment of DDNP wastewater and expands applications of sulfate-radical-based advanced oxidation technology in industrial-wastewater treatment.
1. Introduction
Dinitrodiazophenol (DDNP), described as C6H2(NO2)2N2O, is a nitro derivative of phenol. As a common primary explosive, it has received extensive attention for its metal-free composition and excellent detonation performance.1 DDNP has chemical stability, and can be produced by simple synthetic procedures using raw materials available from abundant sources. Chemical synthesis is a major method to manufacture DDNP. Generally, industrial wastewater of DDNP is generated from manufacturing processes such as reduction, diazotization and washing. Approximately 200–300 kg wastewater is produced for every 1 kg DDNP.2 Wastewater is highly colored and contains complex ingredients, including many persistent organic pollutants (POPs), such as diazophenol and nitrophenol.
Referring to molecular structure of DDNP (Fig. 1), it has two rings and each of them constitutes a conjugate large pi bond.3 When the rings combine together, a large conjugate system is formed. In other words, an integrally closed electron-cloud is formed, which stabilizes the aromatic hydrocarbon. However, the two nitro substituent groups, with high electronegativity, on the benzene ring yield potential for electron attraction. Thus, the electron-cloud density on the benzene ring decreases. As a result, this substance can explode readily. The existence of a diazo and two nitro groups increases its biotoxicity significantly, making it difficult to biodegrade completely. Also, nitrophenol is among the priority pollutants listed by USEPA owing to its characteristics such as high toxicity and the potential danger of carcinogenicity, teratogenicity and mutagenicity to mankind. Therefore, it is of vital importance to dispose DDNP wastewater properly. Several treatment methods work well (i.e., supercritical oxidation,4 electro-catalysis5 and adsorption6), but these are either expensive or generate massive sludge, leaving DDNP wastewater in the environment. Hence, a practical and cost-effective treatment method is required urgently.
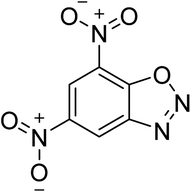 |
| Fig. 1 Molecular structure of DDNP. | |
Recently, emerging oxidation technologies, which are based on sulfate radicals generated by peroxymonosulfate7–9 and persulfate (PS),10 have become popular for eliminating refractory organic pollutants in wastewater. Thanks to the previous efforts made by other researchers, there are some applications for organic pollutants disposal by activated persulfate, such as aniline, phenol,11 p-nitroaniline,12 and bisphenol A.13 Y. Deng and Ezyske14 had effectively applied heat-activated sulfate radical-advanced oxidation process (SR-AOP) for simultaneous removal of refractory organic contaminants and ammonia in landfill leachate. DDNP is an organic contaminant and has functional groups, such as phenyl group, phenolic group or nitro group, which is in common with pollutants mentioned above. In addition, the sulfate radical (SO4˙−, +2.60 V vs. NHE (normal hydrogen electrode)) with powerful oxidation ability, comparatively close to hydroxyl radical (·OH, +2.80 V vs. NHE),15 is able to oxidize organic compounds vigorously in acidic, neutral and alkaline solution. Therefore, DDNP industrial wastewater might be treated effectively by persulfate. Although the treatment processes15–17 based on sulfate radicals are advancing and popular, their applications to DDNP industrial wastewater are rare.
In this study, first, the effects of influent initial pH, PS dosage, temperature and operating time on removal efficiency under heterogeneous conditions were studied by determining COD and color as an evaluation index. Then, after the orthogonal test, the optimized condition was obtained and applied in a scale-up experiment. The results verified the feasibility of this technology for application in treatment of a large amount of sewage in practice. Ultraviolet-Visible (UV-Vis), Fourier Transform Infrared (FTIR) and quenching experiments of free-radical active oxidative substances were performed to explain the mechanism of treatment of DDNP industrial wastewater. This study is aimed to provide a foundation for the rapid degradation of Persistent Organic Pollutants (POPs) in DDNP industrial wastewater and make the process practicable.
2. Material and methods
2.1 Experimental water sample
The DDNP industrial wastewater, used in this study was obtained from a detonator enterprise in southwest China. The waste is brownish red. COD, BOD5, DDNP concentration, pH and discharge were 15
601.8 times, 5854 ± 50 mg L−1, 0, 2600 ± 50 mg L−1, 2.0 ± 0.5 and 5000 t/a, respectively.
2.2 Instruments and drugs
Experimental instruments. High Performance Liquid Chromatography (HPLC) (Agilent 1200, America); FTIR spectrophotometer (Bruker VERTEX 70, Germany); scanning UV-Vis spectrophotometer (Perkin-Elmer Lambda 950, America); universal UV-Vis spectrophotometer (Puyuan Alpha 1500, shanghai, China); a double-hole digital thermostat water-bath (TAISITE DK-98-IIA, Tianjin, China); biochemical incubator (TAISITE SPX-250BIII, Tianjin, China); microwave rapid COD detector (APL MD-6, Chengdu, China); acidometer (Fangzhou pHS-3C+, Chengdu, China); analytical balance (Sartorius BS 124 S, Germany).
Experimental reagents. Potassium persulfate from Merck (Germany), sulfuric acid (H2SO4), sodium hydroxide (NaOH), ethyl alcohol (ETOH), tert-butyl alcohol (TBA) and other chemicals from Chengdu Kelong chemical reagent factory were of analytical grade.
2.3 Methods
2.3.1 Single factor experiments and scale-up experiment. First, 100 mL DDNP wastewater sample with adjusted initial pH was prepared and placed in a water bath with stirring and reflux condensation by a spherical reflux condenser. Second, PS was added as an oxidant when the water temperature rose to a set value and stabilized. Then, the reaction was kept running at a fixed temperature and time. On completion of the reaction time, the effluent was taken out and cooled in cold water. Following this, COD, color and PS concentration of the effluent were detected. Due to the residual PS in the effluent, the detected COD value was virtually larger than the actual COD. According to the previous study, which showed that there is an increase of 29.79 mg in COD for every 1 g K2S2O8,18 we detect the residual persulfate in the effluent water sample and plotted a persulfate concentration standard curve (see Fig. SM-1†). From the abovementioned results, it can be concluded that interference of persulfate in the process of COD determination could be eliminated.
2.3.2 Mixed orthogonal test design. An orthogonal test was used to optimize the operating conditions based on a single-factor experiment. According to the design principle of the orthogonal test program and a test plan of the common orthogonal table,19 the mixed orthogonal test plan was made (see Table 1). This test includes investigating four factors (initial pH, PS dosage, temperature and reaction time) and each of them had three levels. In all, nine experiments were carried out (L9(34)).
Table 1 Mixed orthogonal test design
Factor |
Level |
1 |
2 |
3 |
A (pH) |
7.0 |
8.0 |
9.0 |
B (PS dosage)/g |
20.0 |
22.0 |
24.0 |
C (temperature)/°C |
70 |
80 |
90 |
D (operating time)/min |
45 |
60 |
75 |
2.3.3 Scale-up experiment. Under the optimized operating conditions, which were established from the orthogonal test, the experiment was repeated five times, with the treatment sample increased to 20 L of wastewater. Then, the removal efficiency of COD, BOD, color and DDNP were detected and calculated to recheck the optimal reaction conditions obtained by the mixed orthogonal test and verify the feasibility of this treatment.
2.4 Analytical methods
A microwave-digestion-titration was used to determine the Chemical Oxygen Demand (COD). Dilution and inoculation were chosen to determine the Biochemical Oxygen Demand after 5 days (BOD5). The UV-Vis absorption spectra of water samples treated for different times were carried out in 10 mm quartz cuvettes from 190 to 550 nm by using a UV-Vis spectrometer.20 The FTIR spectrometer and KBr pellets were used to obtain the FTIR spectra, in which both the influent and the effluent were scanned between the wavenumbers of 4000 to 300 cm−1. HPLC was used to analyze the DDNP quantitatively, and the column was eluted with a mixture containing 35% water and 65% acetonitrile with a flow rate of 0.1 mg L−1. The color number (CN) was calculated with the following formula (eqn (1)), where A436, A525 and A620 are the absorbances of the samples at 436 nm, 525 nm and 620 nm, respectively, as detected by a universal UV-Vis spectrometer. |
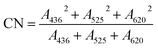 | (1) |
3. Results and discussion
In literature, it has been reported that treatment efficiency of PS oxidation technology is usually influenced by certain operating parameters, such as PS dosage, initial pH, temperature and operating time. Therefore, effect of these factors on the treatment of DDNP industrial wastewater must be investigated.
3.1 Effect of single parameter
3.1.1 Effect of PS dosage. PS dosage is a key factor that affects DDNP wastewater treatment. Under the condition of influent COD of 1560 mg L−1, operating time of 60 min, initial pH of 2.0 approximately and temperature of 75 °C, the effect of PS dosage on the removal efficiency of DDNP wastewater treatment was investigated. Fig. 2(a) presents an increasing trend in the removal efficiency of COD and color as the PS dosage was increased, which is consistent with the observations21 in the study of sulfamethoxazole degradation by microwave-activated persulfate. When the PS dosage was increased from 10.0 g L−1 to 24.0 g L−1, the COD and color removal efficiency increased from 19.28% and 84.84–98.95% and 95.80%, respectively. Moreover, a significant effect of increase in PS dosage on color removal was observed. When the PS dosage was below 22.0 g L−1, the increase in color removal efficiency was conspicuous, whereas above 22.0 g L−1, it improved slightly (from 98.80% to 98.95%). Moreover, COD removal efficiency continued to increase steadily and also worked well at the PS dosage of 22.0 g L−1. Therefore, according to the results obtained herein, PS dosage of 22.0 g L−1 was selected for investigating the effect of initial pH, temperature and operating time on pollutants degradation in the subsequent experiments.
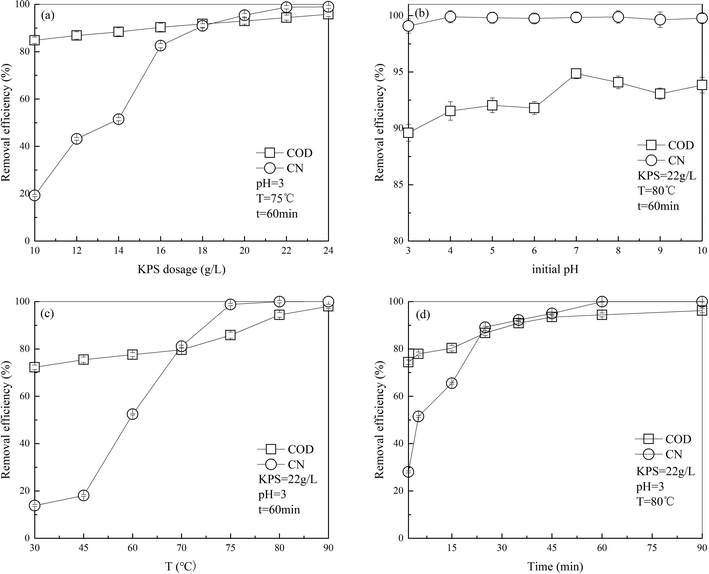 |
| Fig. 2 Effect of (a) PS dosage, (b) initial pH, (c) operating time and (d) temperature on COD and color removal of DDNP industrial wastewater. | |
The results can be explained from two aspects. One is that since S2O82−, activated by heat, can resolve into SO4˙− (eqn (2)),22 which has stronger oxidative ability than persulfate (S2O82−, +2.01 V vs. NHE),23 SO4˙− plays an important role in the oxidation of refractory organics. The initial concentration of SO4˙− increases with an increased in PS dosage; as a consequence, the oxidation effectiveness improves, which could enhance the removal efficiencies of COD and color. However, it was reported in the literature that as the concentration of SO4˙− increases to some extent, the growth of oxidative effectiveness tends to be gradual because the substrate concentration in the system is limited. This result agrees with the observations reported by other researchers24,25 for the degradation of Azo dye acid orange 7 by persulfate oxidation. The other explanation is that additional oxidant is generally good to accelerate the degradation of organics for most organic pollutants. However, in the persulfate system, the increase in removal efficiency slows down slightly while the persulfate exceeds a specific concentration. This is because in presence of excess PS, with which the SO4˙− in solution can react, SO42− is generated. Also, additional SO4˙− can react with itself.26 These reactions (eqn (3) and (4)) consume both PS and SO4˙− and thus, the COD and color removal efficiency decreases.
|
SO4˙− + S2O2−8 → SO2−4 + S2O8˙−, k = 6.1 × 105 M−1 S−1
| (3) |
|
SO4˙− + SO4˙− → S2O2−8, k = 4 × 108 M−1 S−1
| (4) |
3.1.2 Effect of initial pH. Under the condition of influent COD of 1560 mg L−1, PS dosage of 22.0 g L−1, operating time of 60 min and temperature of 80 °C, the effect of initial pH on the removal efficiency of DDNP wastewater treatment was investigated. Fig. 2(b) indicates that the change of initial pH from 3.0 to 10.0 barely influenced the color removal and had a small effect on COD removal. When the pH was less than 7.0, the COD removal efficiency increased by 5.26% with increase in pH. In contrast, the treatment efficiency decreased by 0.76% when the pH exceeded 7.0. It could be concluded that a variation in initial pH matters only slightly for the DDNP wastewater treatment efficiency. Moreover, PS is advantageous for applications in a wide range of pH conditions since the COD and color removal efficiency from initial pH 3.0 to 10.0 exceeded 89%. From abovementioned results, an initial pH of 7 was selected in the subsequent experiments to investigate the effect of temperature and operating time on the pollutants degradation.Results in this part should be discussed in three aspects as the species of free radicals in the system varied with pH condition. During the reactions, SO4˙− was generated by persulfate. It has been reported that SO4˙− together with OH− could produce ·OH in a secondary reaction (eqn (5)).27 In some studies, SO4˙− predominated in acidic solution; SO4˙− and ·OH coexisted in neutral and weakly alkaline solution and ·OH predominated in strongly alkaline solution (pH above 12.0).28–30
|
SO4˙− + OH− → SO2−4 + ˙OH
| (5) |
In acidic solution, the removal efficiency of COD at an initial pH of 3.0 was less than that at an initial pH of 7.0, which could result from the additional SO4˙− generated catalytically by persulfate at excessively rapid speed in acidic solution.31 As a result, the SO4˙− concentration was so large that the probability of noneffective collision between free radicals, such as SO4˙− and S2O82− mentioned in 3.1.1, far exceeded that between free radicals and organic compounds. In addition, S2O82− is reported as a SO4˙− scavenger, particularly in acidic solution.30,32 Thus, the rate of reaction dropped slightly in acidic solution.
More ·OH exists in alkaline solution at pH 9.0 than in neutral solution at pH 7.0. Also, we know that sulfate radical has strong ability of oxidation with higher oxidation-reduction potential (ORP) of 2.50–3.10 V, exceeding that of hydroxyl radical (1.90–2.70 V).15 Thus, it is hypothesized that oxidation capability of the alkaline system is lower than that in neutral condition, which agrees well with the observations reported by Luan et al. for bisphenol A decomposition.33 The COD removal efficiency was slightly lower in alkaline solution than in neutral solution. However, the COD removal efficiency increased slightly as the pH increased from 9.0 to 10.0. This could be because the amount of ·OH rose greatly under strongly alkaline conditions. Hence, the oxidation capability improved again and the COD removal efficiency increased.
In neutral solution, SO4˙− and ·OH coexist in desired concentrations, thus maximizing the oxidation effectiveness. Therefore, an initial pH of 7 was selected in the sequent experiments.
3.1.3 Effect of temperature. Under the condition of influent COD of 1560 mg L−1, PS dosage of 22.0 g L−1, initial pH of 7.0 and an operating time of 60 min, the effect of temperature on the removal efficiency of DDNP wastewater treatment was investigated. Fig. 2(c) shows that the removal efficiency increases with an increase in temperature from 30 °C to 90 °C. Because the removal efficiency was steady above 80 °C, and because the free radicals were exhausted, the velocity of the process was reduced. Hence, a reaction temperature of 80 °C was chosen as the optimum in the subsequent experiments to investigate the effect of operating time on the pollutants degradation.The results could be explained as follows: in the limited temperature range, higher temperature was more likely to break the bond and hence, more SO4˙− would be generated (see eqn (3)); thus, the removal efficiency is proportional to the concentration of oxidative free radical. However, this result does not imply that high-temperature degradation suits all organic compounds. Similar to the other factors mentioned above, a tailored temperature is key to heat-activated persulfate oxidation technology.33,34 For example, it has been discovered that as the temperature increases, excessive free radicals would be generated in the system, which could quench each other and reduce the removal efficiency.35 This result is in accordance with that reported by Hori et al. for perfluorocarboxylic acids decomposition.36 Peyton, in his study on total organic carbon determination of wastewater by persulfate,31 presented that excessively high temperature released free radicals so rapidly that high mineralization efficiency was unexpected.
3.1.4 Effect of reaction time. Under the conditions of influent COD of 1560 mg L−1, PS dosage of 22.0 g L−1, initial pH of 7.0 and a temperature of 80 °C, the effect of time on the removal efficiency of DDNP wastewater treatment was investigated. Fig. 2(d) shows that the removal efficiencies of COD and color increased with time. At 60 min, the color and COD removal efficiency of the DDNP wastewater were 99.96% and 94.40%, respectively. During the first 20 min, the COD removal efficiency increased rapidly and the treatment efficiency was good. After 60 min, increase in COD removal efficiency was extremely slow. The operating time is important in many aspects of wastewater treatment, such as the size of the treatment pond, the duration of the energy cost and the investment. Thus, optimal time-design yields better results. Thus, 60 min was selected as a suitable operating time in the sequent experiments for further investigation.These results may be due to a mass of SO4˙− generated by the high concentration of persulfate, which accelerated the reaction at the beginning. Then, as the reaction proceeded, the reaction slowed down with consumption of persulfate and SO4˙− and the removal efficiency remained the same.
3.2 Optimization by mixed orthogonal test
The range method (intuitive analysis method) and integrated balance method (multiple index evaluation method) were applied to analyze the results from the orthogonal test. Results of the experiment and analysis of the relationships between the four factors (initial pH, PS dosage, temperature and reaction time) referring to the two evaluation standards (COD and color) are listed in Table 2.
Table 2 Results of mixed orthogonal experimentsa
No. |
Factor |
Effluent water |
COD removal efficiency (%) |
Color removal efficiency (%) |
pH |
PS dosage (g L−1) |
Temperature (°C) |
Time (min) |
COD (mg L−1) |
Color (times) |
Kij represents the average value of experiment results of the ith level of the jth factor. |
1 |
7.0 |
20.0 |
70 |
45 |
323.68 |
5541.0 |
79.26 |
87.93 |
2 |
7.0 |
22.0 |
80 |
60 |
110.57 |
119.32 |
92.92 |
99.74 |
3 |
7.0 |
24.0 |
90 |
75 |
22.55 |
8.7488 |
98.56 |
99.98 |
4 |
8.0 |
20.0 |
80 |
75 |
102.39 |
100.9925 |
93.44 |
99.78 |
5 |
8.0 |
22.0 |
90 |
45 |
67.82 |
6.0005 |
95.66 |
99.99 |
6 |
8.0 |
24.0 |
70 |
60 |
282.40 |
5221.7 |
81.91 |
88.62 |
7 |
9.0 |
20.0 |
90 |
60 |
31.22 |
0 |
98.00 |
100.00 |
8 |
9.0 |
22.0 |
70 |
75 |
272.04 |
5041.2 |
82.57 |
89.02 |
9 |
9.0 |
24.0 |
80 |
45 |
134.48 |
373.13 |
91.38 |
99.19 |
Analysis item |
Factor |
A |
B |
C |
D |
COD |
K1 |
90.24 |
90.23 |
81.25 |
88.77 |
K2 |
90.34 |
90.38 |
92.58 |
90.94 |
K3 |
90.65 |
90.62 |
97.41 |
91.52 |
Range |
0.41 |
0.39 |
16.16 |
2.75 |
Best |
A3 |
B3 |
C3 |
D3 |
Priority |
C > D > A > B |
Analysis item |
Factor |
A |
B |
C |
D |
Color |
K′1 |
95.88 |
95.90 |
88.52 |
95.70 |
K′2 |
96.13 |
96.25 |
99.57 |
96.12 |
K′3 |
96.07 |
95.93 |
99.99 |
96.26 |
Range |
0.25 |
0.35 |
11.47 |
0.56 |
Best |
A2 |
B2 |
C3 |
D3 |
Priority |
C > D > B > A |
An analysis of the experimental data shows that the impact of COD and color removal efficiency is of the order C (temperature), D (time), A (initial pH), B (PS dosage) and C, D, B, A, respectively. Clearly, the importance of initial pH and PS dosage conflicts in COD and color removal. According to the specific numeric value of range, except temperature, other factors hardly matter, so parameters of initial pH and PS dosage would be set in an economical way. As a result, the optimum operating conditions were set as initial pH ∼2.0, PS dosage 20 g L−1, temperature 90 °C and operating time 75 min. The conditions of no. 3 and no. 7 experiments are pretty close to the optimum operating conditions. Thus, results of these experiments should be compared to verify effectiveness of the mixed orthogonal experiments.
3.3 Treatment effectiveness under optimized conditions
Under optimum conditions, which were obtained from the orthogonal test, 20 L industrial wastewater was used to carry out the scaled-up repeated experiments. The efficiency is listed in Table 3.
Table 3 Treatment efficiency of DDNP industrial wastewater under optimized conditions
Index |
Influent |
Effluent |
Standarda |
Removal efficiency |
GB 8978-1996 (Integrated wastewater discharge standard) of PRC. |
DDNP/(mg L−1) |
2600.0 |
0 |
1.0 |
100% |
Color |
1 5601.8 |
1.68 |
≤50 |
100% |
COD/(mg L−1) |
5854.9 |
42.83 |
≤60 |
99.22% |
BOD5/(mg L−1) |
0 |
13.4 |
≤20 |
— |
According to the abovementioned results, many types of organic pollutants in the DDNP industrial wastewater can be removed effectively by using advanced oxidation based on persulfate. The removal of DDNP, COD and color were 99.99%, 99.22% and 99.99%, respectively, and the BOD5/COD ratio increased from 0 to 0.31 mg L−1, which indicates that under these conditions, heat-activated advanced oxidation based on persulfate could improve the waste biodegradability significantly, ensuring the safe direct discharge to the specified natural water. Effluent quality reached the primary standard of GB 8978-1996 (Integrated wastewater discharge standard) of PRC. Thus it could be released to specified water (i.e. III-level water listed in GB 3838-2002 (Environmental quality standards for surface water) of PRC and second-class water listed in GB 3097-1997 (sea water quality standard) of PRC).
3.4 Mechanism of DDNP degradation process
Since the DDNP molecule is composed of a phenol, nitro group, diazo group and phenolic hydroxyl group, the variations of special functional groups and special structure with time can be detected by UV-Vis and FTIR analyses.
3.4.1 UV-Vis spectrum analysis. The DDNP molecule has two rings, and each of them constitutes a conjugate large pi bond, resulting in adsorption of DDNP industrial wastewater in the ultraviolet and visible-light regions. Fig. 3 shows the UV-Vis spectra of the influent and the effluent at different times of DDNP industrial wastewater treatment by PS oxidation. For the influent, a broad band between 350 and 420 nm caused by combination of the E2 and B adsorption bands of benzene ring and a maximal peak at 368 nm could be assigned to combination adsorption of chromophonic group (i.e., –NO2) on a larger conjugate system formed by two conjugate systems (benzene ring and –N
N–O–), which leads to red shift (increasing wavelength) and increasing strength of adsorption.20
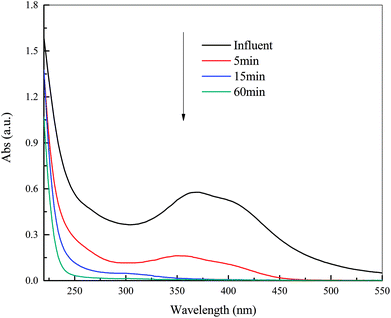 |
| Fig. 3 UV-Vis spectra of DDNP industrial wastewater as a function of time. Condition: temperature of 90 °C, PS dosage of 20.0 g L−1 and initial pH of 2.0. | |
A significant reduction in adsorption band between 350 and 420 nm to zero over time reveals that the characteristic structures in the DDNP industrial wastewater were decomposed step by step with decreasing speed. A blue shift (decreasing wavelength) of maximal adsorption peak to 352 nm after 5 min treatment indicates that the target pollutants degraded. The adsorption band above 275 nm decreased to zero after 60 min of treatment, which indicates that characteristic organic compounds in DDNP industrial wastewater could be decomposed completely by persulfate.
3.4.2 FTIR spectrum analysis. Fig. 4(a) shows the FTIR adsorption spectrum of raw DDNP industrial wastewater. The adsorption band at 3450 cm−1 is attributed to the stretching vibration of –OH with a hydrogen-bond interaction or –OH in –COOH. The weak band at 3095 cm−1 is attributed mainly to the aromatic skeletal stretching vibration. The weaker band at 1068 cm−1 and the comparatively stronger band at 1532 cm−1 are attributed to stretching vibration of the aromatic skeletal. Bands at 739, 706, and 669 cm−1 are attributed to out-of-plane bending vibrations of the aromatic ring. These reveal the existence of significant organic matter with a benzene ring. The band at 1384 cm−1 is attributed to the bending vibration of –CH3 and –CH2. The band at 1343 cm−1 is attributed to the stretching vibration of –NO2. The band at 1137 cm−1 is attributed to the stretching vibration of C–O–H.
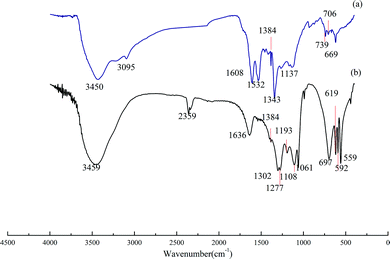 |
| Fig. 4 Infrared absorption spectra of DDNP industrial wastewater before and after oxidation. Condition: temperature of 90 °C, PS dosage of 20.0 g L−1 and initial pH of 2.0. | |
Fig. 4(b) shows the FTIR adsorption spectrum of treated DDNP industrial wastewater. From the large differences between the spectrum in Fig. 4(a) and (b), it can be implied that the compositions of raw wastewater and effluent can be analyzed in five aspects: (a) compared with the standard spectrum, bands that were attributed to aromatic skeletal stretching vibrations at 3095, 1608, and 1532 cm−1 disappeared, which indicates that the benzene ring structure in the DDNP industrial wastewater was destroyed to some degree; (b) the disappearance of the band at 1343 cm−1 is attributed to the stretching vibration of –NO2, and the generation of bands at 1193 and 697 cm−1, implies that the nitro group on the benzene ring was oxidized to a nitric acid compound; (c) reduction of the band at 1384 cm−1, which is attributed to –CH3 and –CH2–, reveals the gradual degradation of the organic compounds by persulfate; also, the generation of a new intensive adsorption band at 2359 cm−1 is attributed to an asymmetric stretching vibration, which indicates that most of the organic compounds in the wastewater are mineralized completely and can be released as CO2 (at pH 2); (d) new bands at 559, 592, 1061, 1277, and 1302 cm−1 are attributed to PS, which reveals a partial oxidant residual, while bands at 619 and 1108 cm−1 indicate the transformation of persulfate into sulfate; (e) the new band at 1193 cm−1 is attributed to the asymmetric stretching vibration of C–O–C, while the other new band at 1636 cm−1 is attributed to a stretching vibration of C
O; thus, it is reasonable to assume that small alcohols dominate the organic composition of the effluent, given that most alcohols are scavengers for sulfate radicals.
3.5 Mechanism of heat-activated PS oxidation process
Ethyl alcohol (ETOH) and tert-butyl alcohol (TBA) were used as scavengers to study the dominant free radicals in heat-activated PS oxidation. Dionysiou et al.37 and Pimentel et al.38 reported that the reaction rate constants of reactions between ETOH and ·OH, ETOH and SO4˙−, TBA and ·OH and TBA and SO4˙− were (0.8–1.0) × 109 M−1 s−1, (0.9–1.3) × 107 M−1 s−1, (3.8–7.6) × 108 M−1 s−1 and (4.0–9.1) × 105 M−1 s−1, respectively. Therefore, ETOH could remove both SO4˙− and ·OH, and also remove ·OH selectively.39,40 The difference in selectivity of SO4˙− and ·OH by the two scavengers was used to verify the existence of SO4˙− and ·OH according to the color removal efficiency of the system that contained each scavenger. Furthermore, we aimed to identify the species that plays a predominant role in the heat-activated PS system. It is not necessary to investigate whether ETOH and TBA are effective in a boiling water system because reflux condensation operations are performed to avoid the mixture volatilization.
With same amount of ETOH and TBA in two separated heat-activated PS systems (molar ratio of PS and the scavengers was 1
:
48.),41 degradation process was expected to be decelerated in different degrees. Results of the scavenging experiments by the two free radicals are shown in Fig. 5.
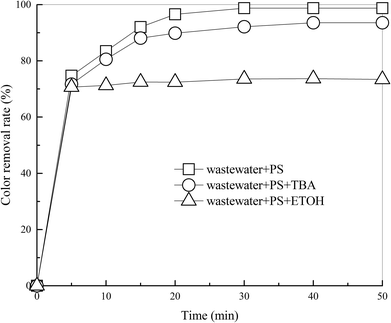 |
| Fig. 5 Effects of TBA and ETOH on DDNP removal efficiency. Conditions: molar ratio of PS and the scavengers was 1 : 48. | |
According to Fig. 5, after 50 min of treatment, addition of TBA moderately reduced the color removal efficiency of industrial wastewater by 5.2% and addition of ETOH reduced it by 25.4%. Results show that ETOH (SO4˙− and ·OH scavenger) could severely inhibit the treatment. This phenomenon provided evidence that hydroxyl or sulfate radicals were involved in the process. Moreover, existence of TBA (·OH scavenger) has a slightly negative impact on the treatment. This indicated that ·OH contributes slightly to the process, so SO4˙− predominates in the heat-activated PS system. This is in accordance with conclusions made by Qi et al.7,16,41 and Zhang et al.
3.6 Analysis of operating cost
The operating cost of heat-activated PS oxidation process mainly contains two parts: the consumption of persulfate potassium (KPS) and power supply for the heater. Under optimal conditions, 20 kg PS would be consumed for treatment of 1 ton DDNP wastewater and the market price for PS (99%) is about 7 CNY/kg on average. The energy requirement of heating up the wastewater from ambient temperature (∼25 °C) to 90 °C was calculated according to specific heat capacity of DDNP wastewater, which is approximately considered equal to that of water (i.e., 4.2 kJ (kg−1 °C−1)). Furthermore, the industrial electricity price is 0.54 CNY/K Wh on average. In all, based on computation, about 180 CNY would be required for the treatment of 1 ton wastewater. In addition, it is remarkable that the effluent can theoretically be used as diluted acid for equipment cleaning and ions recycling since it is acidic and contains abundant NO3− and SO42− ions. Hence, the reuse of the effluent can be favorable for further diminishing the treatment cost.
3.7 Comparison of several techniques
Achievements made in DDNP wastewater treatment in recent years are listed in Table 4. These methods have advantages as well as disadvantages. For example, the combined Fe0/air and Fenton process and combined Fe/Cu/air and Fenton process remove the target pollutants efficiently and are economical for pre-treatment. However, they both take time; thus, reaction containers with same size as those in other treatment process would have lesser throughput. Also, these processes involve complex operations and leave by-products. Sludge, produced during industrial waste water treatment, carries large amounts of POPs such as DDNP and heavy metals, which belong to the category of hazardous waste. Treatment methods of organic–inorganic mixed sludge require further discussion. Moreover, the combined micro-electrolysis and white-rot fungi process costs lesser and removes BOD at the same time. However, it takes more time to cultivate the fungi and strict conditions are required. Compared to the abovementioned methods, the heat-activated PS oxidation has advantages of timesaving, application in a wide range of pH condition and minor sludge generation.
Table 4 Comparison of DDNP industrial wastewater-treatment techniques
Technique |
COD |
Color |
B/C |
Special pollutants |
Cost |
Time/h |
Reference |
Influent mg L−1 |
Removal rate |
Influent/times |
Removal rate |
Effluent mg L−1 |
Influent mg L−1 |
Removal rate |
|
Sludge quantity |
DDNP. Total nitro compound, / represents no data. |
Fe0/air + Fenton + Fe0/air + neutralization |
1250 ± 100 |
78% |
12 500 ± 700 |
98% |
0.27 |
1050 ± 90a |
99.83 |
Low |
Huge |
3.5 |
35 |
Microelectrolysis + white-rot fungi |
2183 |
94% |
/ |
100% |
/ |
2415b |
100% |
Low |
Huge |
108+ |
42 |
1stFe/Cu/air–2ndFenton–3rdFe/Cu/air process |
4740 |
87.1% |
50 000 |
99.9% |
0.58 |
3131 |
100% |
Low |
Huge |
4.5 |
20 |
Heat-activated PS oxidation process |
5854.9 |
99.22% |
15 601.8 |
100% |
0.31 |
2600a |
100% |
Middle |
Zero |
1.25 |
|
4. Conclusions
The optimized treatment condition (temperature 90 °C, reaction time 75 min, PS dosage 20 g L−1 and initial pH ∼2.0) of the heat-activated PS system for DDNP wastewater degradation was obtained from the orthogonal test. Best results were observed during the scale-up experiment under optimized conditions. Removal efficiency of DDNP, COD and color of the waste were almost 99.99%, 99.22% and 99.99%, respectively. The biodegradability improved significantly with the increase in BOD5 (0 to 13.4 mg L−1) and BOD5/COD (0 to 0.31). Furthermore, rational hypotheses of the mechanism were obtained. On the one hand, the UV-Vis adsorption spectrum could confirm that the advanced oxidation process based on persulfate could decompose DDNP industrial wastewater completely. The FTIR adsorption spectrum revealed achievement of organic pollutants degradation in the system by destroying the aromatic compounds during mineralization and oxidizing the nitro group on the benzene ring to nitrate. On the other hand, a verification test of the free radicals reveals that SO4˙− and ·OH participate in the heat-activated PS system, among which SO4˙− predominates. The cost of the treatment is about 180 CNY/t. It is expected that this rapid heat-activated PS oxidation process would be applied in practice as a promising way of advanced treatment of DDNP industrial wastewater.
Conflicts of interest
There are no conflicts to declare.
Acknowledgements
The authors gratefully acknowledge the financial support of the Key Laboratory of Special Waste Water Treatment (SWWT2015-4).
References
- M. H. Huynh, M. A. Hiskey, T. J. Meyer and M. Wetzler, Proc. Natl. Acad. Sci. U. S. A., 2006, 103, 5409–5412 CrossRef PubMed
. - J. Zhai and Y. Wang, Procedia Environ. Sci., 2013, 18, 632–637 CrossRef
. - G. Holl, T. M. Klapoetke, K. Polborn and C. Rienäcker, Propellants, Explos., Pyrotech., 2003, 28, 153–156 CrossRef
. - Z. L. Meng, Y. C. Liu and T. Chai, Chin. J. Explos. Propellants, 2007, 30, 77–80 Search PubMed
. - R. G. Fan, C. E. Li, Y. X. Bai, D. Q. Huang, L. W. Fang and Q. C. Wang, Ind. Water Treat., 2011, 49, 251–257 Search PubMed
. - T. L. Li, X. B. Guo, Y. Y. Li, J. Jin and G. S. Deng, Chem. Bioeng., 2008, 5, 62–65 Search PubMed
. - C. Qi, X. Liu, J. Ma, C. Lin, X. Li and H. Zhang, Chemosphere, 2016, 151, 280 CrossRef PubMed
. - C. Qi, X. Liu, C. Lin, H. Zhang, X. Li and J. Ma, Chem. Eng. J., 2017, 315, 201–209 CrossRef
. - Z. H. Wang, R. T. Bush, L. A. Sullivan, C. C. Chen and J. S. Liu, Environ. Sci. Technol., 2014, 48, 3978–3985 CrossRef PubMed
. - L. W. Matzek and K. E. Carter, Chemosphere, 2016, 151, 178–188 CrossRef PubMed
. - P. Avetta, A. Pensato, M. Minella, M. Malandrino, V. Maurino, C. Minero, K. Hanna and D. Vione, Environ. Sci. Technol., 2014, 49, 15883–15891 Search PubMed
. - Y. S. Zhao, C. Sun, J. Q. Sun and R. Zhou, Sep. Purif. Technol., 2015, 142, 182–188 CrossRef
. - X. Jiang, Y. Wu, P. Wang, H. Li and W. Dong, Environ. Sci. Pollut. Res. Int., 2013, 20, 4947–4953 CrossRef PubMed
. - Y. Deng and C. M. Ezyske, Water Res., 2011, 45, 6189–6194 CrossRef PubMed
. - W. D. Oh, Z. Dong and T. T. Lim, Appl. Catal., B, 2016, 194, 169–201 CrossRef
. - C. Qi, X. Liu, L. Yang, C. Lin, J. Ma, X. Li and H. Zhang, J. Hazard. Mater., 2017, 328, 98–107 CrossRef PubMed
. - B. T. Zhang, Y. Zhang, Y. G. Teng and M. Fan, Crit. Rev. Environ. Sci. Technol., 2015, 45, 1756–1800 CrossRef
. - Z. Wang, Y. S. Lu, Z. L. Wu and Z. W. Zhang, Ind. Water Treat., 2014, 34, 78–81 Search PubMed
. - R. J. Liang, Carbohydr. Polym., 2008, 73, 558–563 CrossRef PubMed
. - Y. Yuan, P. Cao, B. Lai, P. Yang and Y. Zhou, RSC Adv., 2016, 6, 35539–35549 RSC
. - C. Qi, X. Liu, C. Lin, H. Zhang, J. Ma, H. Tan and W. Ye, Chem. Eng. J., 2014, 249, 6–14 CrossRef
. - D. A. House, Chem. Rev., 1961, 62, 185–203 CrossRef
. - M. Ahmad, A. L. Teel and R. J. Watts, Environ. Sci. Technol., 2013, 47, 5864–5871 CrossRef PubMed
. - C. Liang, C. J. Bruell, M. C. Marley and K. L. Sperry, Chemosphere, 2004, 55, 1213–1223 CrossRef PubMed
. - S. Yang, X. Yang, X. Shao, R. Niu and L. Wang, J. Hazard. Mater., 2011, 186, 659–666 CrossRef PubMed
. - X. Y. Yu, Z. C. Bao and J. R. Barker, ChemInform, 2004, 35, 295–308 Search PubMed
. - M. S. Tsao and W. K. Wilmarth, J. Phys. Chem., 1959, 63, 346–353 CrossRef
. - K. C. Huang, R. A. Couttenye and G. E. Hoag, Chemosphere, 2002, 49, 413 CrossRef PubMed
. - C. Liang and H. W. Su, Ind. Eng. Chem. Res., 2009, 48, 472–475 Search PubMed
. - Y. Gao, N. Gao, Y. Deng, Y. Yang and Y. Ma, Chem. Eng. J., 2012, 195–196, 248–253 CrossRef
. - G. R. Peyton, Mar. Chem., 1993, 41, 91–103 CrossRef
. - S. Y. Yang, Y. Xin, W. Ping, S. Liang and W. Y. Zhang, Mod. Chem. Ind., 2009, 29, 13–19 Search PubMed
. - H. Luan, L. Yang, S. Lu, L. Miao and K. Dong, Chin. J. Environ. Eng., 2016, 10, 2459–2464 Search PubMed
. - A. Ghauch and A. M. Tuqan, Chem. Eng. J., 2012, 183, 162–171 CrossRef
. - Y. Yuan, B. Lai and Y. Y. Tang, Chem. Eng. J., 2016, 283, 1514–1521 CrossRef
. - H. Hori, A. Yamamoto, E. Hayakawa, S. Taniyasu, A. Nobuyoshi Yamashita, S. Kutsuna, H. Kiatagawa and R. Arakawa, Environ. Sci. Technol., 2005, 39, 2383 CrossRef PubMed
. - G. P. Anipsitakis and D. D. Dionysiou, Environ. Sci. Technol., 2004, 38, 3705 CrossRef PubMed
. - M. Pimentel, N. Oturan, M. Dezotti and M. A. Oturan, Appl. Catal., B, 2008, 83, 140–149 CrossRef
. - Y. Liao, G. Liu, L. Zhao, D. Kong and J. Lu, Acta Sci. Circumstantiae, 2014, 34, 931–937 Search PubMed
. - J. F. Zhang, X. Yang, W. Zheng, L. R. Kong and L. H. Wang, Chin. J. Environ. Sci., 2008, 29, 1239 Search PubMed
. - C. Qi, X. Liu, W. Zhao, C. Lin, J. Ma, W. Shi, Q. Sun and H. Xiao, Environ. Sci. Pollut. Res., 2015, 22, 4670–4679 CrossRef PubMed
. - L. Li and X. C. Zhang, J. Anhui Univ. Sci. Technol., 2007, 27, 39–42 CrossRef
.
Footnote |
† Electronic supplementary information (ESI) available. See DOI: 10.1039/c8ra01995a |
|
This journal is © The Royal Society of Chemistry 2018 |
Click here to see how this site uses Cookies. View our privacy policy here.