DOI:
10.1039/C8RA01987H
(Paper)
RSC Adv., 2018,
8, 15202-15206
Selective catalytic amination of halogenated aldehydes with calcined palladium catalysts
Received
6th March 2018
, Accepted 13th April 2018
First published on 23rd April 2018
Abstract
This work focuses on understanding the influence of the conditions used in the calcination step of palladium catalysts on the performance of this catalyst in the reductive amination of halogen-containing substrates. The results show that increasing the calcination temperatures (from 100 °C to 400 °C) has a detrimental effect on catalytic activity but a strong positive effect on the selectivity (from 45 to 96%), avoiding the undesired dehalogenation reaction. TEM investigation showed that the reason for the different selectivity can be addressed to different Pd mean particles size and particle size distribution. In particular, larger Pd particles obtained at the highest calcination temperature (400 °C) showed the best selectivity to halogenated benzylamines (96%), with a good stability in terms of both activity and selectivity as confirmed by performing recycling tests.
Introduction
The selective conversion of one functional group in a multifunctional feed substrate has been an area of continuous high interest throughout the chemical, pharmaceutical and agrochemical industry.1–5 In particular, halogen atoms are often incorporated next to other functional groups in active ingredients or in precursors of those active ingredients. Moreover, more than 25% of the registered drugs contain a tertiary amine group which make them a very important class of pharmacophores. However, the methods described in literature for the selective synthesis of halogenated tertiary benzylamines mostly involve equimolar amounts of salt formation or suffer from low selectivities. The ability to synthesize these halogenated tertiary amines on an industrial efficient way can open new possibilities for the synthesis of these pharmacophores and agrochemical intermediates.6,7 A widely used synthesis route of these compounds involve the substitution reaction of benzylhalides by a secondary amine with the formation of an equimolar amount of ammonium salts.8–13 The reductive amination reaction of halogenated aldehydes as a way to obtain halogenated benzylamine have also gained interest in the last decades. The synthetic protocol involves the formation of an imine or iminium intermediate upon exposure of a carbonyl compound to a secondary amine followed by an in situ reduction to an alkylated amine (Scheme 1). However, procedures described in literature mostly use a hydride reagent such as sodium borohydride which can results in a high selectivity towards the halogenated tertiary amine14,15 or the use of hydrogen gas as the reducing agent in combination with a metal catalyst which results in no salt formation but suffers from selectivity losses due to dehalogenation.16–19
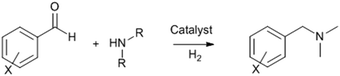 |
| Scheme 1 Scheme of reaction of the reductive amination of halogen substituted benzaldehyde. | |
Therefore, there is a need for a new and readily available catalytic system for the selective amination of halogenated aldehydes to form multifunctional tertiary amines which can be applied in a multi-step synthesis of different end products such as API's or agrochemicals. If a catalytic system could be found that uses hydrogen as the reducing agent, eliminates the formation of salts as by-product and reduces the amount of dehalogenation, an industrial efficient and green synthesis route towards these halogenated tertiary amines becomes feasible.
Palladium has been widely used for the reductive amination of carbonyl compounds.20–22 The drawback of using Pd catalysts in the case of amination of halogen substituted carbonyl compound is its capability to displace the halogen atom by a hydrogen atom. A strategy to avoid the undesired dehalogenation is to modify the Pd active sites. This modification can be done by for example a modification of the support. It has been shown, indeed, that the use of hierarchical-porosity silica monoliths, obtained by post grafting of sulfonic groups as a support in the hydrogenation of halogenated nitro aromatics could decrease the dehalogenation significantly.23 The fixation of palladium nanoparticles inside beta zeolite crystals in the hydrogenation of halogenated nitroarenes also showed a beneficial effect in comparison with conventional palladium nanoparticles supported on zeolite crystals and commercial available Pd/AC catalysts.24 Another way to modify the Pd active sites is by the addition of a second metal. For example, the addition of Ag to Pd can suppress the dehalogenation during the reductive amination of halogen substituted benzaldehyde.25,26 Probably the addition of Ag poisoned the most reactive Pd sites responsible of the dehalogenation. Bair et al. also describes the addition of Pb to a Pd/AC catalyst for the selective synthesis of aromatic haloamines out of aromatic halonitroamines by suppressing the unwanted dehalogenation reaction. While a commercial available Pd/AC catalyst shows almost complete dehalogenation, the addition of Pb could decrease the dehalogenation down to levels of 5%.27 In this work we showed that a similar effect can be obtained by pretreating Pd/AC catalyst at different temperatures before the utilization in the amination of halogen (Cl, Br) substituted benzaldehyde with dimethylamine (DMA).
Experimental
Catalyst treatment
A 5% wt palladium catalyst (Pd/AC) was obtained from the company Evonik (30% wt wet). An amount of 10 g of this catalyst was dried at 80 °C (Pd80/AC) in static air for 3 hours and subsequently heat-treated under a nitrogen flow of 30 mL min−1 at 100, 200 or 400 °C temperatures for 4 hours. The catalysts were labelled as Pd100/AC, Pd200/AC and Pd400/AC, respectively. As a comparison, the catalyst was also calcined at 400 °C without being dried beforehand (Pd400wet/AC).
Catalytic tests
In a typical experiment, a 100 mL autoclave (Parr) was loaded with 2.5 g of a halogen substituted benzaldehyde obtained from the company Sigma Aldrich and 12 mL of dimethyl amine in methanol solution having a 2 M concentration. The reactor was sealed and the gas phase was flushed three times with nitrogen and then pressurized at 5 bar by the addition of nitrogen gas. The reactor was heated to 80 °C and then continuously stirred for 60 minutes at a temperature of 80 °C and a pressure of 5 bar. Then, the reactor was cooled to 30 °C and degassed. 8 mg of the catalyst was added to the autoclave. The autoclave was heated in 15 minutes to 100 °C and hydrogen was added to a final pressure of 40 bar. The reductive amination reaction was allowed to proceed for the indicated time at 100 °C. Then the reactor was cooled down and degassed at room temperature. Subsequently, a sample of the reaction product liquid was taken and analyzed by gas chromatography (GC) using a HP 7820A gas chromatograph equipped with a capillary column HP-5 30 m × 0.32 mm, 0.25 μm film, by Agilent Technologies. Identification of the products was performed using a Thermo Scientific Trace ISQ QD Single Quadrupole GC-MS equipped with a capillary column HP-5 30 m × 0.32 mm, 0.25 × m film, by Agilent Technologies. Recycling tests were carried out under the same experimental conditions. The catalyst was recycled in the subsequent run after filtration without any further treatment.
Characterization
Electron micrographs were obtained using a ZEISS LIBRA200FE microscope equipped with a 200 kV FEG source. Energy-dispersive X-ray spectra (EDS – Oxford INCA Energy TEM 200) and elemental maps were collected along with HAADF-STEM (high angular annular dark field scanning transmission electron microscopy) images. Before the analysis, the samples were suspended in isopropanol and sonicated, then each suspension was dropped onto a holey carbon-coated copper grid (300 mesh) and the solvent was evaporated. Histograms of the particle size distribution were obtained by counting at least 300 particles onto the micrographs; the mean particle diameter (dm) was calculated by using the formula dm = Σdini/Σni where ni was the number of particles of diameter di.
Results and discussion
Commercial 5% palladium catalyst supported on carbon (20% wt wet) was tested in the reductive amination of halogen substituted benzaldehyde with dimethyl amine in methanol solution. The aim of this work is to elucidate the effect of the heat treatment on the morphology and the catalytic activity of a Pd/AC catalyst. Therefore, before utilization, a portion of the catalyst was dried at 80 °C in static air for 3 hours and subsequently heat-treated under a nitrogen flow of 30 mL min−1 at 100, 200 or 400 °C for 4 hours. In comparison, the wet catalyst was calcined at 400 °C without being dried beforehand. The reaction was performed in two steps. In the first step, the proper amount of halogen substituted benzaldehydes and dimethyl amine in methanol solution were added to the reactor and then continuously stirred for 60 minutes at a temperature of 80 °C and a pressure of 5 bar of N2, to form the halogen imine (Scheme 1). Then, the reactor was cooled to 30 °C, degassed and the catalyst was added to the autoclave. The successive reductive amination reaction was performed at 100 °C and at 40 bar of hydrogen. Table 1 reports the results obtained using the palladium catalysts treated at different temperature in the reductive amination of 2-Cl-benzaldehyde with DMA. All the catalysts were very active reaching full conversion after 15 minutes (entry 1–4), except when treated at 400 °C (entry 5 and 6).
Table 1 Reductive amination of 2-Cl-benzaldehyde with DMA using Pd/AC catalystsa
Entry |
Catalyst |
Reaction time (min) |
Conv (%) |
BDMA (%) |
2-Cl-BDMA (%) |
Others (%) |
Reaction conditions: 2.5 g of a halogen substituted benzaldehyde and 12 mL of dimethyl amine in methanol (2 M). 1st step: 80 °C, 5 bar N2. 2nd step: 100 °C, 40 bar H2, 8 mg catalyst. Data from ref. 25. |
1 |
Pd/AC |
15 |
99.9 |
44.8 |
34.4 |
20.5 |
2 |
Pd80/AC |
15 |
99.8 |
44.9 |
34.6 |
20.4 |
3 |
Pd100/AC |
15 |
99.7 |
45.1 |
34.7 |
19.8 |
4 |
Pd200/AC |
15 |
94.3 |
42.4 |
39.3 |
18.0 |
5 |
Pd400/AC |
15 |
44.0 |
2.1 |
97.8 |
— |
30 |
82.3 |
2.4 |
96.2 |
— |
6 |
Pd400wet/AC |
15 |
81.2 |
7.4 |
91.2 |
— |
30 |
98.9 |
12.2 |
87.4 |
— |
7 |
PdCu/ACb |
15 |
98.3 |
0.5 |
98.2 |
0.9 |
8 |
PdAu/ACb |
15 |
95.6 |
1.5 |
96.2 |
— |
9 |
PdNi/ACb |
15 |
80.6 |
0.8 |
96.2 |
— |
10 |
PdAg/ACb |
15 |
72.2 |
— |
98.9 |
— |
Pd400/AC showed a conversion of 44 and 82.3% after 15 and 30 minutes, respectively (entry 5). The calcination carried out on the wet Pd/AC sample affected less the activity being the conversion obtained after 15 min of 81.2% instead of 44% for the catalyst calcined in the dried form (entry 6 vs. 5).
Pd/AC produced benzyldimethylamine (BDMA) as main product (44.8%, entry 1) whereas the selectivity to the desired 2-Cl-benzyldimethylamine (2-Cl-BDMA) was lower (34.4% – entry 1). As expected from previous works, the untreated Pd/AC was efficient in the reductive amination but also promoted the dehalogenation of the aromatic group.25 No changes in the selectivity were observed drying the sample in static air at 80 °C or calcining the sample under N2 flow at 100 °C (34.6 and 34.7% of selectivity to 2-Cl-BDMA, entry 2 and 3), whereas the heat treatment at 200 °C moderately improved the selectivity (39.3%, entry 4).
A much higher selectivity to 2-Cl-BDMA (93.6%) was observed for the catalyst heat-treated at higher temperature (400 °C) (entry 5). Interestingly, calcining the Pd catalyst at 400 °C without a drying step at 80 °C (Pd400wet/AC), a lower selectivity to 2-Cl-BDMA (87.4%, entry 6) compared to Pd400/AC was obtained. Pd/AC catalyst performances in terms of both activity and selectivity appeared to be very sensitive to the initial amount of water during the calcination, water being detrimental in terms of selectivity. Pd400/AC showed results comparable to the best bimetallic systems reported in the literature (Table 1).
To better understand the effect of the heat treatment on the catalytic activity, the morphology of the catalysts was investigated by transmission electron microscopy (TEM). The commercial Pd/AC catalysts showed a mean particle diameter (dm) of 2.1 nm with a narrow particle size distribution (1.0–4.0 nm) (Fig. 1a). The drying step of the sample at 80 °C or the calcination at 100 °C did not affect the morphology of the catalyst (data not shown), whereas the treatment of the Pd catalyst at 200 °C results in a slight increase in the particle size (2.9 nm) and a larger particle distribution (Fig. 1b). Evident changes in the Pd particle sizes were observed when the sample was calcined at 400 °C, with an increase of mean diameter from 2.1 nm to 6.3 nm together with a broadening of the size distribution (2.5–10.0 nm) (Fig. 1c). It is worth noting that the same thermal treatment (calcination at 400 °C) carried out in the presence of water (i.e. without the drying step) leads to a final mean particle diameter of 4.6 nm, evidencing the role of water in limiting the Pd particles growth (Fig. 1d).
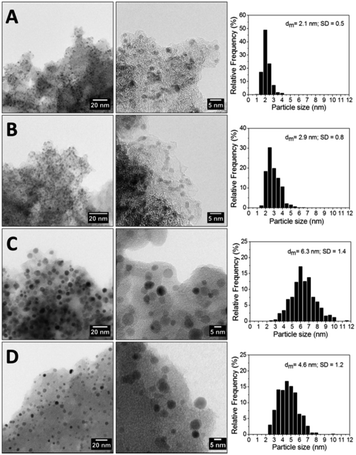 |
| Fig. 1 High resolution TEM micrographs (left side and middle) and histogram of particle size distribution (right side) of Pd/AC (A), Pd200/AC (B), Pd400/AC (C), Pd400wet/AC (D). | |
Correlating the structure of the different catalysts with their activity and above all their selectivity, it clearly appears that the catalytic performances (activity and selectivity) are ruled out from the particle size. The catalysts with larger particles are less active but less prone to remove the halogen group, resulting in a higher selectivity to 2-Cl-BDMA.
Indeed, the following correlations were observed for particle size Pd/AC (2.1 nm) = Pd80/AC (2.1 nm) = Pd100/AC (2.1 nm) < Pd200/AC (2.9 nm) < Pd400wet/AC (4.6 nm) < Pd400/AC (6.3) and for the selectivity to 2-Cl-BDMA, Pd/AC (34.3%) = Pd80/AC (34.6%) = Pd100/AC (34.6%) < Pd200/AC (39.3%) < Pd400wet/AC (91.2%) < Pd400/AC (96.2%).
The long-term stability of the most selective catalyst, Pd400/AC, was then investigated. Recycling tests were carried out by filtering the catalyst and reusing it without any further purification for the next run. The results highlighted the excellent stability for six cycles in terms of both activity and selectivity as shown in Fig. 2.
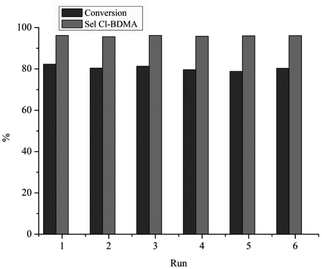 |
| Fig. 2 Stability tests using Pd400/AC in the amination of 2-Cl-benzaldehyde with DMA. | |
Therefore, we extended the study to the amination of different halogen substitutes benzaldehyde with DMA to show the general applicability of our catalytic system (Table 2). The positive effect of calcination at 400 °C has been confirmed in all cases. Selectivity of calcined samples with Cl-derivatives is always over 90% except in the case of 2,4-dichloro. It was also noted that when the Cl substituent was substituted by Br the selectivity (62.5%) and the activity decreased.
Table 2 Reductive amination of halogenated-benzaldehyde with DMA using Pd/AC catalystsa
Substrate |
Time of reaction (min) |
Conv (%) |
BDMA (%) |
Halogenated BDMA (%) |
Others (%) |
Reaction conditions: 2.5 g of a halogen substituted benzaldehyde and 12 mL of dimethyl amine in methanol (2 M). 1st step: 80 °C, 5 bar N2. 2nd step: 100 °C, 40 bar H2, 8 mg catalyst. |
Pd/AC |
2 Cl-aldehyde |
15 |
99.9 |
45.8 |
33.4 |
20.6 |
4 Cl-aldehyde |
15 |
83.1 |
48.3 |
30.2 |
20.3 |
2,4 Cl-aldehyde |
15 |
78.3 |
48.2 |
30.5 |
19.7 |
2 Br-aldehyde |
15 |
74.8 |
58.3 |
25.5 |
14.3 |
![[thin space (1/6-em)]](https://www.rsc.org/images/entities/char_2009.gif) |
Pd400/AC |
2 Cl-aldehyde |
15 |
44.0 |
2.1 |
97.8 |
— |
30 |
82.3 |
2.4 |
96.2 |
0.8 |
4 Cl-aldehyde |
15 |
38.2 |
5.4 |
92.6 |
0.1 |
30 |
78.6 |
6.6 |
91.3 |
0.3 |
2,4 Cl-aldehyde |
15 |
35.9 |
7.4 |
93.2 |
0.5 |
30 |
76.8 |
9.4 |
88.3 |
0.7 |
2 Br-aldehyde |
15 |
36.8 |
27.8 |
62.5 |
7.8 |
30 |
71.4 |
34.1 |
53.6 |
10.1 |
Conclusions
Pd on carbon treated at different temperatures was used for the reductive amination of halogen substituted benzaldehyde with dimethylamine to obtain halogenated benzylamines, important pharmacophores and agrochemical intermediates. The results show that the increase of the calcination temperatures (from 100 °C to 400 °C) has a positive effect on the selectivity toward halogenated benzylamines, avoiding the undesired dehalogenation. A drying pre-treatment at 80 °C appeared fundamental in improving the catalyst selectivity. Correlating the catalytic results with TEM investigation it appeared that there is a clear correlation between catalytic activity and the catalyst morphology. In particular, bigger Pd particles obtained at the highest calcination temperature (400 °C) showed the best selectivity to halogenated benzylamines (92–98%), with a good stability as confirmed performing recycling tests. Pd400/AC showed results comparable to best ones reported in the literature using bimetallic systems.
Conflicts of interest
There are no conflicts to declare.
Notes and references
- M. Baumann and I. R. Baxendale, Beilstein J. Org. Chem., 2015, 11, 1194–1219 CrossRef CAS PubMed.
- A. M. Bezborodov and N. A. Zagustina, Appl. Biochem. Microbiol., 2016, 52, 237–249 CrossRef CAS.
- B. W. Cue and J. Zhang, Green Chem. Lett. Rev., 2009, 2, 193–211 CrossRef CAS.
- M. D. Eastgate, M. A. Schmidt and K. R. Fandrick, Nat. Rev. Chem., 2017, 1, 16 CrossRef.
- K. C. Nicolaou, D. Vourloumis, N. Winssinger and P. S. Baran, Angew. Chem., Int. Ed., 2000, 39, 44–122 CrossRef CAS PubMed.
- Y. Ren, Y. Su, L. Sun, S. He, L. Meng, D. Liao, X. Liu, Y. Ma, C. Liu, S. Li, H. Ruan, X. Lei, X. Wang and Z. Zhang, J. Med. Chem., 2017, 60, 972–986 CrossRef CAS PubMed.
- A. Johansson, C. Löfberg, M. Antonsson, S. von Unge, M. A. Hayes, R. Judkins, K. Ploj, L. Benthem, D. Lindén, P. Brodin, M. Wennerberg, M. Fredenwall, L. Li, J. Persson, R. Bergman, A. Pettersen, P. Gennemark and A. Hogner, J. Med. Chem., 2016, 59, 2497–2511 CrossRef CAS PubMed.
- J. Hartwing, US Pat. 2014/0221691, 2014.
- M. Kurosu and D. C. Crick, Tetrahedron Lett., 2006, 47, 5325–5328 CrossRef CAS.
- Z. Dong, J. Wang and G. Dong, J. Am. Chem. Soc., 2015, 137, 5887–5890 CrossRef CAS PubMed.
- E. Tayama and H. Kimura, Angew. Chem., Int. Ed., 2007, 46, 8869–8871 CrossRef CAS PubMed.
- S. Weisbrod and A. Marx, Synlett, 2010, 787–789 CAS.
- K. Šindelář, J. Holubek, E. Svátek, O. Matoušová, J. Metyšová and M. Protiva, J. Heterocycl. Chem., 1989, 26, 1325–1330 CrossRef.
- S. Bhattacharyya, J. Org. Chem., 1995, 60, 4928–4929 CrossRef CAS.
- M. R. Saidi, R. S. Brown and A. Ziyaei-Halimjani, J. Iran. Chem. Soc., 2007, 4, 194–198 CrossRef CAS.
- F. Mao, D. Sui, Z. Qi, H. Fan, R. Chen and J. Huang, RSC Adv., 2016, 6, 94068–94073 RSC.
- B. Li, J. Zheng, W. Zeng, Y. Li and L. Chen, Synthesis, 2016, 49, 1349–1355 CrossRef.
- A. R. Jadhav, H. A. Bandal and H. Kim, Chem. Eng. J., 2016, 295, 376–383 CrossRef CAS.
- S. Lu, J. Peng, J. Wu, C. Li, X. Cao and H. Gu, Chem. Commun., 2016, 52, 760–763 RSC.
- E. Byun, B. Hong, K. A. De Castro, M. Lim and H. Rhee, J. Org. Chem., 2007, 72, 9815–9817 CrossRef CAS PubMed.
- J. Zhu, J. Zhou, T. Zhao, X. Zhou, D. Chen and W. Yuan, Appl. Catal., A, 2009, 352, 243–250 CrossRef CAS.
- M. M. Dell'Anna, P. Mastrorilli, A. Rizzuti and C. Leonelli, Appl. Catal., A, 2011, 401, 134–140 CrossRef.
- F. Liguori, P. Barbaro, B. Said, A. Galarneau, V. D. Santo, E. Passaglia and A. Feis, ChemCatChem, 2017, 9, 3245–3258 CrossRef CAS.
- J. Zhang, L. Wang, Y. Shao, Y. Wang, B. C. Gates and F.-S. Xiao, Angew. Chem., Int. Ed., 2017, 56, 9747–9751 CrossRef CAS PubMed.
- K. Moonen, B. Vandeputte, D. Scheldeman and K. Dumoleijn, WO 2015032653, 2013.
- C. W. Wigbers, A. G. Altenhoof, M. Siemer, J.-P. Melder and C. Eidamshaus, WO 2014202436, 2013.
- S. G. Hindin, D. L. Bair and D. R. Steele, US Pat. 3.666.813, 1969.
|
This journal is © The Royal Society of Chemistry 2018 |