DOI:
10.1039/C8RA00291F
(Paper)
RSC Adv., 2018,
8, 7832-7838
Antiacetylcholinesterase triterpenes from the fruits of Cimicifuga yunnanensis†
Received
11th January 2018
, Accepted 10th February 2018
First published on 19th February 2018
Abstract
Two new cycloartane triterpenes, cimyunnin E (1), containing a unique oxaspiro[4.4]nonanedione moiety based on rings D and E, together with cimicifine B (2), a 25,26,27-trinortriterpene featuring a pyridine ring E, were purified from the fruits of Cimicifuga yunnanensis. Their structures were elucidated by spectroscopic methods and ECD (electronic circular dichroism calculations). Compounds 1 and 2 showed significant acetylcholinesterase (AChE) inhibition with IC50 values of 1.58 and 3.87 μM, respectively. In addition, they noticeably enhanced the neurite outgrowth of nerve growth factor (NGF) mediated PC12 cells at a concentration of 10 μM.
Introduction
Plants of the Cimicifuga genus, such as C. racemosa, C. foetida, C. dahurica, C. heracleifolia, C. simplex, and C. japonica, are well-known herbal medicines in Europe, the United States, and East Asia.1–4 In the past few decades, more than 300 9,19-cycloartane triterpenes (CTs), which are considered to be the main active chemical constituents of this genus, have been reported.5–30 Moreover, biological evaluations revealed these CTs possessed a variety of activities, such as cytotoxicity,5,12–14 antiosteoporotic,31 anti-AIDS,32 immunosuppression,33 anti-angiogenic,6 and anti-Alzheimer.34
It is worth mentioning that previous studies had mainly focused on the roots of aforementioned Cimicifuga spp.5–30 In an attempt to further explore structurally and biologically interesting CTs from this genus, we started to study some rare species, such as C. yunnanensis and C. frigida, particularly, on the fruits and flowers of these plants. As a result, a number of novel bioactive CTs were discovered. Cimyunnin A, a CTs possessed an unique cyclopentenone ring G from the fruits of C. yunnanensis, was considered as an anti-angiogenic leading structure.6 In addition, cimifrigines A–G, a series of cytotoxic CTs featured by an unusual oxime group at C-15, were identified from the flowers of C. frigida.35 Be motivated by these findings, we continually carried out phytochemical and pharmaceutical studies on the fruits of C. yunnanensis. Consequently, another two novel triterpenes, cimyunnin E (1) and cimicifine B (2), were isolated and identified (Fig. 1). Compound 1 represents the first example of CTs with an unprecedented oxaspiro[4.4]nonanedione unite formed in ring D and E. While, compounds 2 is a trinortriterpene containing a pyridine ring E. Significantly, biological evaluations revealed that compounds 1 and 2 were strong AChE inhibitors with IC50 values of 1.58 and 3.87 μM, respectively (the IC50 value of positive control tacrine is 0.17 μM, see Table S1 and Fig. S22†). Moreover, the neuronal differentiation of NGF-mediated PC12 cells were also enhanced by compounds 1 and 2 at the concentration of 10 μM (Table S2 and Fig. S23†). Described herein are the isolation, structure elucidation, and biological activities of these compounds.
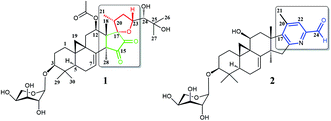 |
| Fig. 1 Structures of compounds 1 and 2. | |
Results and discussion
Cimyunnin E (1), white powder, had the molecular formula C37H54O12 as determined by the HRESIMS at m/z 713.3515 [M + Na]+ (calcd 713.3513), corresponding to 11 double-bond equivalents. The IR spectrum showed absorptions attributable to OH (3425 cm−1), carbonyl (1745 cm−1) and olefinic (1603 cm−1) groups, respectively. In the 1H NMR spectrum (Table 1), downfield shifted cyclopropane methylene signals at δH 0.69 and 1.04 (each 1H, d, J = 3.6 Hz) and an anomeric proton resonance at δH 4.76 (d, J = 7.1 Hz) were observed. The 13C (Table 1) and HMQC spectra revealed the existence of two olefinic carbons at δC 117.0 (C-7, d) and 140.5 (C-8, s), an ester carbonyl group at δC 170.1 (s), two carbonyl carbons at δC 199.9 (C-15, s) and 204.6 (C-16, s), and six oxygenated carbon atoms at δC 87.6 (C-3, d), 67.2 (C-12, d), 87.4 (C-17, s), 78.3 (C-23, d), 79.4 (C-24, d), and 72.1 (C-25, s), respectively. These data suggested that 1 was a highly oxygenated CTs glycoside with a six-ring skeleton.
Table 1 1H and 13C NMR data of compounds 1 and 2 (δ in ppm, J in Hz)
Position |
1 |
2 |
δHb |
δCc |
δHb |
δCc |
Signals overlapped. Recorded at 600 MHz in pyridine-d5. Recorded at 150 MHz in pyridine-d5. |
1 |
1.57a, 1.23 m |
29.9 t |
2.76 m, 1.70 m |
27.2 t |
2 |
2.29 m, 1.88 m |
29.2 t |
2.42 brd (11.8), 2.13 brd (12.8) |
29.6 t |
3 |
3.44 dd (11.5, 3.7) |
87.6 d |
3.61 dd (11.5, 4.1) |
88.2 d |
4 |
|
40.2 s |
|
40.6 s |
5 |
1.18 m |
41.4 d |
1.38a |
43.7 d |
6 |
1.94 m, 1.53 m |
21.5 t |
2.00 m, 1.81 m |
21.9 t |
7 |
6.60 d (6.9) |
117.0 d |
5.36 d (7.0) |
115.2 d |
8 |
|
140.5 s |
|
145.8 s |
9 |
|
20.7 s |
|
27.5 s |
10 |
|
28.0 s |
|
29.3 s |
11 |
2.76 dd (15.8, 7.8), 1.42 brd (16.3) |
35.3 t |
4.62 m |
62.8 d |
12 |
6.20 d (7.6) |
67.2 d |
3.08 dd (13.4, 9.5), 2.47 brd (11.3) |
43.8 t |
13 |
|
46.8 s |
|
49.2 s |
14 |
|
54.9 s |
|
51.1 s |
15 |
|
199.9 s |
3.32 d (15.4), 3.01 d (15.4) |
43.6 t |
16 |
|
204.6 s |
|
165.2 s |
17 |
|
87.4 s |
|
148.4 s |
18 |
1.37 s |
18.5 q |
1.38 s |
23.7 q |
19 |
1.04 d (3.6), 0.69 d (3.6) |
26.8 t |
2.04 d (3.7), 1.08a |
18.7 t |
20 |
2.55 m |
37.0 d |
|
142.8 s |
21 |
1.29 brd (8.3) |
18.6 q |
2.22 s |
17.9 q |
22 |
2.92 dd (20.2, 8.3), 1.93 m |
38.7 t |
7.66 s |
123.1 d |
23 |
4.54 m |
78.3 d |
|
151.4 s |
24 |
3.74 brd (6.3) |
79.4 d |
10.22 s |
193.7 s |
25 |
|
72.1 s |
|
|
26 |
1.58 s |
28.1 q |
|
|
27 |
1.50 s |
25.8 q |
|
|
28 |
1.67 s |
27.0 q |
1.06 s |
28.3 q |
29 |
1.30 s |
25.5 q |
1.42 s |
25.8 q |
30 |
1.00 s |
13.9 q |
1.15 s |
14.3 q |
3-Ara |
|
|
|
|
1′ |
4.76 d (7.1) |
107.3 d |
4.84 d (7.0) |
107.3 d |
2′ |
4.45 t (7.9) |
72.8 d |
4.47 m |
72.8 d |
3′ |
4.16 dd (8.9, 2.6) |
74.5 d |
4.18 m |
74.5 d |
4′ |
4.30 brs |
69.5 d |
4.31a |
69.4 d |
5′ |
4.28 brd (12.6), 3.78 brd (12.6) |
66.8 t |
4.30 m, 3.79 m |
66.7 t |
12-OCOCH3 |
2.12 s |
|
|
|
12-OCOCH3 |
|
21.4 q |
|
|
12-OCOCH3 |
|
170.1 s |
|
|
The detailed 1D and 2D NMR analyses established the planar structure of 1. The 1H–1H COSY (Fig. 2) spectrum disclosed that 1 has partial structures –CH2CH2CH– (due to C-1 to C-3), –CHCH2CH– (for C-5 to C-7), and –CH2CH– (for C-11 to C-12), that were compatible for rings A, B, and C of the CTs with a pair of double bond at C-7 and C-8.27,29 The existence of the downfield shifted cyclopropane methylene (δH 0.69 and 1.04) further supported this deduction. The acetoxy group was located to C-12 on the basis of the HMBC correlation of H-12 (δH 6.20) and the ester carbonyl group (δC 170.1). Similarly, the sugar unit was attached to C-3 by the HMBC coupling between the anomeric proton (δH 4.76) and C-3 (δC 87.6). In addition, the sugar obtained after acid hydrolysis was identified as L-arabinose by comparing its TLC and specific rotation with a standard. Thus, ring A, B, C, F, and the sugar unit of 1 was constructed as shown (Fig. 2).
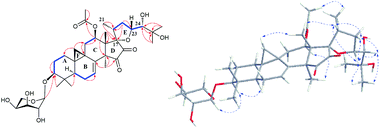 |
| Fig. 2 Major HMBC ( ), 1H–1H COSY ( ), and ROESY ( ) correlations of compound 1. | |
The spin system –CH3CHCH2CHCH– due to Me-21, C-20, C-22, C-23 and C-24 was also deduced from 1H–1H COSY correlations (Fig. 2). In addition, HMBC correlations from CH3-21 (δH 1.29) and H-22 (2.92, 1H) to the oxygenated quaternary carbon at δC 87.4 (C-17) indicated the linkage of C-20 and C-17 (Fig. 2). Similarly, the connection of C-24 to the isopropanol group (C-25, C-26, and C-27) was elucidated by the HMBC couplings of H-24 (δH 3.74) CH3-26 (δH 1.58) and CH3-27 (δH 1.50) to the hydroxyl substituted quaternary carbon at δC 72.1 (C-25). By now, there were still one oxygen atom and two carbonyl carbons (C-15, δC 199.9; C-16, δC 204.6) need to be assigned and four degree of unsaturation unaccounted for, requiring another two rings in the final structure. In the HMBC spectrum, correlations of CH3-28 (δH 1.67) to C-14 (δC 54.9) and C-15 (δC 199.9) were observed, indicating the connection between C-14 and C-15. Similarly, the connection of C-13 and C-17 was deduced from HMBC correlations of CH3-18 (δH 1.37) to C-13 (δC 46.8), and C-17 (δC 87.4), respectively. Thus, to fulfill the unsaturation requirement, the molecular weight, and the chemical shift of C-15 and C-16, C-17 was connected to C-16 and C-23 was linked to C-17 by an oxygen atom (based on HMBC correlations, C-17 and C-23 could be connected by the carbonyl carbon C-16. Thus, ring D would be a five-membered lactone ring. In that case, the chemical shift for C-15 should around δC 170.0). Therefore, an unique 15,16-cyclopentanedione-17-spiro-17,23-oxolane moiety was established in 1. Finally, the planar structure of 1 was constructed as shown (Fig. 2).
In the ROESY spectrum (Fig. 2), correlations of H-5 (biogenetically α-oriented)/H-3, H-3/H-1′, and Me-28 (biogenetically α-oriented)/H-12 indicated the α-orientation of H-1′, H-3, and H-12. Our efforts to make fine crystals from 1 failed which precluded the possibility to determine the absolute configuration directly by X-ray crystallography. However, the diagnostic ROESY couplings of Me-18 (biogenetically β-oriented) to H-20, H-20 to H-23, and acetoxy methyl to Me-21 (biogenetically α-oriented) were observed, which help to determine β-orientation of H-20 and H-23 and the conformation of ring E as shown (Fig. 2). Therefore, the relative configuration of C-17, C-20, and C-23 of 1 was assigned as S*, R*, and R*, respectively. For the 17R*, 20S*, and 23S* stereoisomer of 1, those correlations would not be observed (except H-20 to H-23, Fig. S21†), which further confirmed this deduction. On the basis of ROESY correlations of H-23/H-24, H-24/H-22, and H-23/Me-27 and the 3JH,H value of H-23 and H-24 (6.3 Hz), the Newman projection of C-23/C-24 coupling system was established as shown (Fig. 3), which help to establish the S* configuration of C-24. Finally, the ECD calculation was applied to determine the absolute configuration of 1. As shown in Fig. 4, spectrum calculated for the 17S, 20R, 23R, and 24S one was nearly identical with the experimental data of 1 over the whole range of wavelengths under investigation, whereas the stereoisomer exhibited very different ECD behaviour between 250–300 nm. Therefore, the absolute configurations of C-17, C-20, C-23, and C-24 of 1 were determined as S, R, R, and S, respectively.
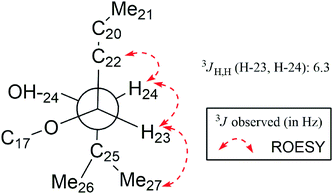 |
| Fig. 3 The Newman projection of C-23/C-24 coupling system of 1. | |
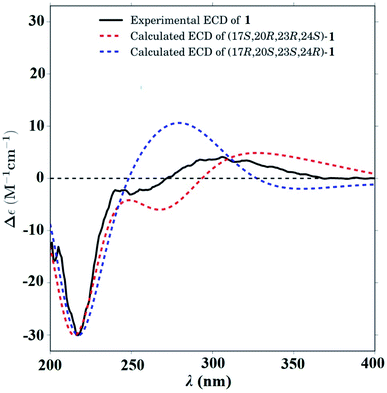 |
| Fig. 4 Calculated ECD spectra for -(17S,20R,23R,24S)-1 (red dashed line) and -(17R,20S,23S,24R)-1 (blue dashed line). Experimental CD spectrum of 1 (black solid line). | |
The molecular composition of cimicifine B (2), C32H43NO7, was deduced from HRESIMS ([M + Na]+, m/z 576.2931), indicating 12 degrees of unsaturation. In the 1H NMR spectrum (Table 1) of 2, signals due to an extremely downfield shifted cyclopropane methylene at δH 1.08 (overlapped) and 2.04 (d, J = 3.7 Hz), an anomeric proton at δH 4.84 (d, J = 7.0 Hz), two olefinic hydrogen atoms at δH 5.37 (d, J = 7.0 Hz) and 7.66 (s), five tertiary methyl groups at δH 1.06–1.42, and an active hydrogen signal at δH 10.22, were observed. The 13C NMR and DEPT (Table 1) spectra displayed signals for a carbonyl group (δC 193.7, C-24), a hydroxylmethine (δC 62.8, C-11), two trisubstituted olefinic carbons (δC 115.2, C-7; δC 123.1, C-22), and five tetrasubstituted olefinic groups (δC 145.8, C-8; δC 165.2, C-16; δC 148.4, C-17; δC 142.8, C-20; δC 151.4, C-23). Therefore, aforementioned data suggested that 2 was a trinortriterpene glycoside and a six-ring structure, which included an unsaturated azacycle, was required to fulfill the unsaturation requirement.
Extensive analyses of 2D NMR spectra revealed the conformation of ring A, B, C, D, and F of 2 as shown (Fig. 5), which was similar to those of known compounds.5–30 In the 1H–1H COSY spectrum (Fig. 5), the correlations of H-5 (δH 1.38) to H-6 (δH 1.81 and 2.00, each 1H) and H-6 to the olefinic proton (δH 5.37, H-7) indicated a pair of double bond at C-7 and C-8. The 1H–1H COSY association of the proton (δH 4.62) of hydroxymethine (δC 62.8, C-11) to H-12 (δH 2.47 and 3.08, each 1H), together with the extremely downfield shifted proton signals of C-19 determined the location of a hydroxyl group at C-11. In the HMBC spectrum (Fig. 5), the couplings of H-15 (δH 3.01 and 3.32, each 1H) to C-16 (δC 165.2) and C-17 (δC 148.4), and Me-18 (δH 1.38) to C-17 (δC 148.4) indicated the double bond between C-16 and C-17. Further analysis of HMBC spectrum revealed the correlations of Me-21 (δH 2.22) to C-20 (δC 142.8) and C-17 (δC 148.4), and H-22 (δH 7.66) to C-20 (δC 142.8) and C-23 (δC 151.4), indicating the linkage of C-17/C-20/C-22/C-23. In addition, the connection between C-23 and C-24 was elucidated by the HMBC correlation of H-22 (δH 7.66) to the carbonyl carbon (δC 193.7, C-24). Thus, to fulfill the double-bond equivalents, a pyridine ring E should be fused to ring D through C-16 and C-17. Similarly, to fulfill the molecular weight of 2 an aldehyde group was assigned to C-24, which was further supported by the HMBC correlation of an active hydrogen (δH 10.22) and C-23 (δC 151.4). Finally, by the same way as that of 1, the sugar was identified as L-arabinose and located at C-3. The orientations of H-3 and H-11 were ascribed by the ROESY correlations (Fig. 5) between H-5 (biogenetically α-oriented) and H-3, and H-11. Thus, the structure of 2 was determined as shown.
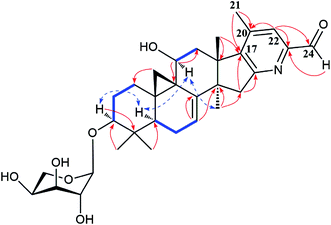 |
| Fig. 5 Major HMBC ( ), 1H–1H COSY ( ), and ROESY ( ) correlations of compound 2. | |
Hypothetically, prevention of acetylcholine (Ach) hydrolysis could increase the efficiency of cholinergic transmissions, which has been reported to be associated with the onset of Alzheimer's disease (AD).36 Thus, enhancement of ACh levels by potent AChE inhibitors in the brain has been considered to be an effective approach for treating AD.37–39 Although prominent biological activities of CTs from Cimicifuga spp. have been reported, to date, however, anti-AChE knowledge of those chemical constituents is mainly not yet involved. Thus, the AChE inhibitory activities of compounds 1 and 2 were evaluated using the Ellman method.40 Unexpectedly, compound 1 exhibited significant inhibition on AChE with an IC50 value of 1.58 μM (Table S1 and Fig. S22†). Similarly, compound 2 showed noticeable inhibitory effect on AChE, having an IC50 value of 3.87 μM. Tacrine was used as the positive control and had an IC50 value of 0.17 μM.
AD is a type of neurodegenerative diseases. Any agent with neurotrophic activity may benefit AD. Therefore, the effects of 1 and 2 to stimulate NGF-mediated neurite outgrowth on PC12 cells were further evaluated. As a result, 1 and 2 obviously increased the neuronal differentiation at a concentration of 10 μM. The differentiation rates are 15.34% and 11.72% for 1 and 2, respectively, compared with 4.17% of the negative control and 19.18% of the positive control (Table S2 and Fig. S23†).
Conclusions
In our continual investigation on the fruits of C. yunnanensis, another two unusual CTs were obtained. Cimyunnin E (1) is the first CTs possessing an oxaspiro[4.4]nonanedione moiety in ring D and E. While, cimicifine B (2) is a trinortriterpene contains a fused pyridine ring E. Significantly, these two compounds showed potent anti-AChE effects and neurotrophic activities. Therefore, the bioactivities of cimyunnin E and cimicifine B deserve further study. In summary, once again, novel active constituents were isolated from fruits of C. yunnanensis, the more sophisticated parts of this genus, such as pollen and vegetative organ are worth studying in future.
Experimental section
General experimental procedures
A JASCO P-1020 digital polarimeter was applied to record optical rotations, using MeOH as solvent. 1D and 2D NMR spectra were performed on Bruker DRX-500 and Avance III-600 MHz spectrometers (Bruker, Zűrich, Switzerland) with solvent signal as internal reference. ESIMS and HRESIMS were run on an Agilent G6230 TOF MS (Agilent Technologies, Palo Alto, USA). Infrared spectra were tested on a Shimadzu IR-450 instrument with KBr pellets. Column chromatography (CC) was run on Silica gel (200–300 mesh, Qingdao Marine Chemical, Inc.), and Lichroprep RP-18 (40–63 μm, Merck). Semipreparative HPLC was carried out on an Agilent 1100 liquid chromatography system using an YMC-Pack 10 mm × 250 mm column (Pro C18 RS). Precoated TLC plates (200–250 μm thickness, silica gel 60 F254, Qingdao Marine Chemical, Inc.) were used for thin-layer chromatography. The spots in TLC were visualized by heating after spraying with 10% aq. H2SO4.
Plant material
The fruits of Cimicifuga yunnanensis (1.5 kg) were collected from Bomi County, Tibet, China, in September 2013. Prof. Wang Zongyu, Kunming Institute of Botany, Chinese Academy of Sciences, identified the species. A voucher specimen (KUN no. 201309007) has been deposited at the State Key Laboratory of Phytochemistry and Plant Resources in West China, Kunming Institute of Botany, Chinese Academy of Sciences, PR China.
Extraction and isolation
MeOH (6 L, 3 times, 7 days each) was used to extract the dried and milled fruits of Cimicifuga yunnanensis (1.5 kg) at room temperature. MeOH was evaporated under vacuum at 50 °C to afford the extract (156.3 g). The extract gave fractions A (28.2 g), B (31.3 g), C (29.5 g), D (27.3 g) and E (12.7 g) by silica gel CC (3.0 kg, 10 × 150 cm) eluted with CHCl3–MeOH [100
:
0 (6 L), 50
:
1 (10 L), 10
:
1 (10 L), 5
:
1 (7 L), 0
:
100 (4 L)]. Subsequently, five sub-fractions (C.1–C.5) were obtained through RP-18 CC (1000 g, 12 × 60 cm), gradiently eluted with MeOH–H2O from 0
:
100 to 100
:
0. Fractions (C.3.1–C.3.4) were obtained by further RP-18 CC (eluted with MeOH–H2O, gradient from 40
:
60 to 85
:
15) on fraction C.3. Compound 1 (3.5 mg) was purified from fraction C.3.2 (1.4 g) by silica gel CC (40 g, 3 × 40 cm) eluted with CHCl3–Me2CO (gradient from 10
:
1 to 5
:
1), and then repeated semipreparative HPLC (eluted with CH3CN–H2O, gradient from 50
:
50 to 65
:
35). Fraction C.2 (3.9 g) yielded compound 2 (2.6 mg) by silica gel CC (40 g, 3 × 60 cm) eluting with CHCl3–Me2CO from 20
:
1 gradient to 10
:
1 and repeated semipreparative HPLC (eluted with CH3CN–H2O, gradient from 55
:
45 to 70
:
30).
(3β,12β,17S,20R,23R,24S)-17,23-Epoxy-15,16-dione-12-acetoxy-3,25-dihydroxy-7-en-9,19-cycloart-3-O-α-larabinopyranoside (1). White powder; [α]24D = −304.0 (c 0.10, MeOH); IR (KBr): νmax 3425, 2927, 2853, 1745, 1603, 1460, 1382, 1242, 1085, 989 cm−1; UV (MeOH) λmax (log
ε): 205 (0.28), 250 (0.04); see Table 1 for 1H NMR (600 MHz, C5D5N) and 13C NMR (150 MHz, C5D5N) data; positive ESIMS [M + Na]+ m/z 713; positive HRESIMS [M + Na]+ m/z 713.3515 (calcd for C37H54NaO12, 713.3513).
(3β,11β)-11-Hydroxy-16,23-nitrilo-7,17(20),22-trien-24-formyl-25,26,27-trinor-9,19-cycloart-3-O-α-larabinopyranoside (2). White powder; [α]19D = −4.8 (c 0.13, MeOH); IR (KBr): νmax 3426, 2927, 2854, 1707, 1630, 1599, 1451, 1383, 1259, 1070, 589 cm−1; UV (MeOH) λmax (log
ε): 203 (0.55), 273 (0.13); see Table 1 for 1H NMR (600 MHz, C5D5N) and 13C NMR (150 MHz, C5D5N) data; positive ESIMS [M + Na]+ m/z 576; positive HRESIMS [M + Na]+ m/z 576.2931 (calcd for C32H43NNaO7, 576.2937).
Hydrolysis and identification of the sugar units in compounds 1 and 2
The MeOH solution (3 mL) of each compound (1.5 mg) was refluxed with 0.5 N HCl (2 mL) for 2 h. CHCl3 (3 × 6 mL) was used to extract the reaction mixture after diluting with H2O. A monosaccharide was given by neutralizing each aqueous layer with Ag2CO3 and filtering the precipitate. The monosaccharide from compounds 1 and 2 had an Rf (EtOAc–CHCl3–MeOH–H2O, 3
:
2
:
2
:
1) and specific rotation of [α]20D +85.4 (c 0.08, MeOH) corresponding to those of L-arabinose (Sigma-Aldrich).
Acetylcholinesterase inhibitory activity
Acetylcholinesterase (AChE) inhibitory activities of compounds 1 and 2 were assayed by the spectrophotometric method developed by Ellman et al.40 with slightly modification. S-Acetylthiocholine iodide, 5,5′-dithio-bis-(2-nitrobenzoic) acid (DTNB, Ellman's reagent), and acetylcholinesterase derived from human erythrocytes were purchased from Sigma Chemical. Compounds 1 and 2 were dissolved in DMSO. The reaction mixture (totally 200 μL) containing phosphate buffer (pH 8.0), test compound (50 μM for preliminary screening; 100, 50, 30, 10, 3, 1, and 0.2 μM for IC50 value assay), and acetylcholinesterase (0.02 U mL−1) was incubated for 20 min (30 °C). Then, the reaction was initiated by the addition of 40 μL of solution containing DTNB (0.625 mM) and acetylthiocholine iodide (0.625 mM) for AChE inhibitory activity assay. The hydrolysis of acetylthiocholine was monitored at 405 nm every 30 seconds for one hour. Tacrine was used as positive control with final concentration of 0.333 μM for preliminary screening and 2, 1, 0.5, 0.2, 0.04, 0.008, and 0.0016 μM for IC50 value assay. All the reactions were performed in triplicate. The percentage inhibition was calculated as follows: % inhibition = (NC − S)/NC × 100 (NC (negative control) is the activity of the enzyme without test compound and with 2% DMSO and S is the activity of enzyme with test compound and the final concentration of DMSO is 0.1%). Inhibition curves were obtained for each compound by plotting the percent inhibition versus the logarithm of inhibitor concentration in the assay solution. The linear regression parameters were determined for each curve and the IC50 values extrapolated. The same procedure was applied for the positive control tacrine.
Neurite outgrowth-promoting activity
The neurotrophic activities of the tested compounds were examined according to an assay using PC12 cells as reported.41 Briefly, PC12 cells (purchased from Kunming institute of zoology) were maintained in F12 medium (Ham's F12K, Gibco's reagent) supplemented with 12.5% horse serum (HS, Hyclone's reagent), and 2.5% fetal bovine serum (FBS, Hyclone's reagent), and incubated at 5% CO2 and 37 °C. Tested compounds were dissolved in DMSO. For the neurite outgrowth-promoting activity bioassay, PC12 cells were seeded at a density of 5 × 104 cells per mL in 48-well plate coated with poly-L-lysine (sigma's reagent). After 24 h, the medium was changed to that containing 10 μM of each test compounds plus 5 ng mL−1 NGF (sigma's reagent), or different concentrations of NGF (50 ng mL−1 for the positive control, 5 ng mL−1 for the negative control). The final concentration of DMSO was 0.05%, and the same concentration of DMSO was added into the negative control. After 72 h incubation, the neurite outgrowth was assessed under a phase contrast microscope. Neurite processes with a length equal to or greater than the diameter of the neuron cell body were scored as neurite bearing cells. The ratio of the neurite-bearing cells to total cells (with at least 100 cells examined per view area; 5 viewing area per well) was determined and expressed as a percentage.
ECD calculation
The theoretical calculations were carried out using Gaussian 09.42 Structures were first optimized at PM6 using semi-empirical theory method and then optimized at HF/6-31G(d) theory level. Room-temperature equilibrium populations were calculated according to Boltzmann distribution law (eqn. (1)). The conformers with Boltzmann-population of over 1% were chosen and further optimized at B3LYP/6-311G(d,p) in methanol using the IEFPCM model (Table S3†). Vibrational frequency analysis confirmed the stable structures. |
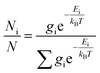 | (1) |
where Ni is the number of conformer i with energy Ei and degeneracy gi at temperature T, and kB is Boltzmann constant. Under the same condition, the ECD calculation was conducted using time-dependent density functional theory (TD-DFT). Rotatory strengths for a total of 30 excited states were calculated. The ECD spectrum was simulated in SpecDis43 by overlapping Gaussian functions for each transition according to (eqn. (2)): |
 | (2) |
where σ represents the width of the band at 1/e height, and ΔEi and Ri are the excitation energies and rotatory strengths for transition i, respectively. Parameters of σ and UV-shift for enantiomers were 0.5 eV and 1 nm, respectively.
Conflicts of interest
There are no conflicts of interest to declare.
Acknowledgements
This project was supported by the National Natural Science Foundation of China (81302670 and U1132604) and the Foundation of State Key Laboratory of Phytochemistry and Plant Resources in West China, Kunming Institute of Botany, CAS (P2015-KF03).
Notes and references
- E. Liske and P. Wustenberg, Menopause, 1998, 5, 250–255 CrossRef.
- D. J. McKenna, K. Jones, S. Humphrey and K. Hughes, Altern. Ther., 2001, 7, 93–100 CAS.
- S. Nobuko, K. Mutsuo, T. Harukuni, M. Teruo, E. Fumio, N. Hoyoku, N. Masahiro, S. Yohiro and H. L. Kuo, Bioorg. Med. Chem., 2005, 13, 1403–1408 CrossRef PubMed.
- Chinese Pharmacopoeia Commission, The Pharmacopoeia of Chinese People's Republic, ed. Y. Li, The Chemical Industry Publishing House, Beijing, China, 2010, vol. 1, pp. 68–69 Search PubMed.
- Y. Nian, H. Zhu, W. R. Tang, Y. Luo, J. Du and M. H. Qiu, J. Nat. Prod., 2013, 76, 896–902 CrossRef CAS PubMed.
- Y. Nian, J. Yang, T. Y. Liu, Y. Luo, J. H. Zhang and M. H. Qiu, Sci. Rep., 2015, 5, 9026–9031 CrossRef CAS PubMed.
- J. X. Li and Z. Y. Yu, Curr. Med. Chem., 2006, 13, 2927–2951 CrossRef CAS PubMed.
- L. Wu, Z. L. Chen, Y. Su, Q. H. Wang and H. X. Kuang, Chin. J. Nat. Med., 2015, 13, 81–89 CAS.
- Y. Su, L. Wu, G. Mu, Q. Wang, B. Yang, G. Cheng and H. X. Kuang, Bioorg. Med. Chem., 2017, 25, 4917–4923 CrossRef CAS PubMed.
- C. N. Lv, F. Yang, R. L. Qin, Z. Y. Qi, W. R. Zhou and J. C. Lu, Bioorg. Med. Chem. Lett., 2017, 27, 3305–3309 CrossRef CAS PubMed.
- N. M. Bao, Y. Nian, W. H. Wang, X. L. Liu, Z. T. Ding and M. H. Qiu, Phytochem. Lett., 2015, 12, 200–202 CrossRef CAS.
- W. H. Wang, Y. Nian, Y. J. He, L. S. Wan, N. M. Bao, G. L. Zhu, F. Wang and M. H. Qiu, Tetrahedron, 2015, 71, 8018–8025 CrossRef CAS.
- N. M. Bao, Y. Nian, G. L. Zhu, W. H. Wang, L. Zhou and M. H. Qiu, Fitoterapia, 2014, 99, 191–197 CrossRef CAS PubMed.
- Y. Nian, H. Y. Wang, L. Zhou, J. Su, Y. Li and M. H. Qiu, Planta Med., 2013, 79, 60–69 CAS.
- Y. Nian, H. Y. Wang, J. Su, L. Zhou, G. Feng, Y. Li and M. H. Qiu, Tetrahedron, 2012, 68, 6521–6527 CrossRef CAS.
- Y. Nian, X. M. Zhang, Y. Li, Y. Y. Wang, J. C. Chen, L. Lu, L. Zhou and M. H. Qiu, Phytochemistry, 2011, 72, 1473–1481 CrossRef CAS PubMed.
- Y. Nian, Y. L. Zhang, J. C. Chen, L. Lu, C. Qing and M. H. Qiu, J. Nat. Prod., 2010, 73, 93–98 CrossRef CAS PubMed.
- Y. Nian, J. C. Chen, L. Lu, X. M. Zhang and M. H. Qiu, Helv. Chim. Acta, 2009, 92, 112–120 CrossRef CAS.
- L. Lu, J. C. Chen, Y. Li, C. Qing, Y. Y. Wang, Y. Nian and M. H. Qiu, Chem. Pharm. Bull., 2012, 60, 571–577 CrossRef CAS PubMed.
- H. Y. Wang, Y. Nian, C. Y. Ma, Y. B. Song, L. Zhou and M. H. Qiu, Chin. J. Chem., 2012, 30, 1265–1268 CrossRef CAS.
- D. S. Li, Y. Nian, Y. Sun and M. H. Qiu, Helv. Chim. Acta, 2011, 94, 632–638 CrossRef CAS.
- L. R. Sun, J. Yan, L. Zhou, Z. R. Li and M. H. Qiu, Molecules, 2011, 16, 5701–5708 CrossRef CAS PubMed.
- L. Lu, J. C. Chen, H. J. Song, Y. Li, Y. Nian and M. H. Qiu, Chem. Pharm. Bull., 2010, 58, 729–733 CrossRef CAS PubMed.
- L. Lu, J. C. Chen, H. J. Song, Y. Nian and M. H. Qiu, Molecules, 2009, 14, 1578–1584 CrossRef CAS PubMed.
- L. R. Sun, J. Yan, Y. Nian, L. Zhou, H. J. Zhang and M. H. Qiu, Molecules, 2008, 13, 1712–1721 CrossRef CAS PubMed.
- L. R. Sun, C. Qing, Y. L. Zhang, S. Y. Ji, Z. R. Li, S. J. Pei, M. H. Qiu, M. L. Gross and S. X. Qiu, Beilstein J. Org. Chem., 2007, 3, 1–6 CrossRef PubMed.
- L. R. Sun, J. Yan, L. Lu, S. J. Pei, Z. R. Li, L. Zhou, X. M. Zhang and M. H. Qiu, Helv. Chim. Acta, 2007, 90, 1313–1318 CrossRef CAS.
- Z. Ali, S. L. Khan, R. S. Pawar, D. Ferreira and I. K. Khan, J. Nat. Prod., 2007, 70, 107–110 CrossRef CAS PubMed.
- C. Dan, Y. Zhou, Y. Deng, S. L. Peng, L. S. Ding, M. L. Gross and S. X. Qiu, Org. Lett., 2007, 9, 1813–1816 CrossRef CAS PubMed.
- J. C. Gao, F. Huang, J. C. Zhang, G. Y. Zhu, M. S. Yang and P. G. Xiao, J. Nat. Prod., 2006, 69, 1500–1502 CrossRef CAS PubMed.
- J. X. Li, J. Liu, C. C. He, Z. Y. Yu, Y. Du, S. Kadota and H. Seto, Maturitas, 2007, 58, 59–69 CrossRef CAS PubMed.
- N. Sakurai, J. H. Wu, Y. Sashida, Y. Mimaki, T. Nikaido, K. Koike, H. Itokawa and H. Lee, Bioorg. Med. Chem. Lett., 2004, 14, 1329–1332 CrossRef CAS PubMed.
- J. H. Lee, T. D. Cuong, S. J. Kwack, J. H. Seok, J. K. Lee, J. Y. Jeong, M. H. Woo, J. S. Choi, H. K. Lee and B. S. Min, Planta Med., 2012, 78, 1391–1394 CrossRef CAS PubMed.
- M. A. Findeis, F. Schroeder, T. D. McKee, D. Yager, P. C. Fraering, S. P. Creaser, W. F. Austin, J. Clardy, R. Wang, D. Selkoe and C. B. Eckman, ACS Chem. Neurosci., 2012, 3, 941–951 CrossRef CAS PubMed.
- Y. Nian, H. Yan, X. N. Li, L. Zhou and M. H. Qiu, RSC Adv., 2017, 7, 38557–38564 RSC.
- A. Enz, R. Amstutz, H. Boddeke, G. Gmelin and J. Malonowski, Prog. Brain Res., 1993, 98, 431–445 CrossRef CAS PubMed.
- E. K. Perry, Br. Med. Bull., 1986, 42, 63–69 CrossRef CAS PubMed.
- R. T. Bartus, L. D. Dean, B. Beer and A. S. Lippa, Science, 1982, 217, 408–417 CAS.
- G. Benzi and A. Morreti, Eur. J. Pharmacol., 1998, 346, 1–13 CrossRef CAS PubMed.
- G. L. Ellman, K. D. Courtney, V. J. Andres and R. M. Featherstone, Biochem. Pharmacol., 1961, 7, 88–95 CrossRef CAS PubMed.
- L. A. Greene and A. S. Tischler, Proc. Natl. Acad. Sci. U. S. A., 1976, 73, 2424–2428 CrossRef CAS.
- M. J. Frisch, G. W. Trucks, H. B. Schlegel, G. E. Scuseria, M. A. Robb, J. R. Cheeseman, G. Scalmani, V. Barone, B. Mennucci, G. A. Petersson, H. Nakatsuji, M. Caricato, X. Li, H. P. Hratchian, A. F. Izmaylov, J. Bloino, G. Zheng, J. L. Sonnenberg, M. Hada, M. Ehara, K. Toyota, R. Fukuda, J. Hasegawa, M. Ishida, T. Nakajima, Y. Honda, O. Kitao, H. Nakai, T. Vreven, J. A. Montgomery Jr, J. E. Peralta, F. Ogliaro, M. Bearpark, J. J. Heyd, E. Brothers, K. N. Kudin, V. N. Staroverov, R. Kobayashi, J. Normand, K. Raghavachari, A. Rendell, J. C. Burant, S. S. Iyengar, J. Tomasi, M. Cossi, N. Rega, J. M. Millam, M. Klene, J. E. Knox, J. B. Cross, V. Bakken, C. Adamo, J. Jaramillo, R. Gomperts, R. E. Stratmann, O. Yazyev, A. J. Austin, R. Cammi, C. Pomelli, J. W. Ochterski, R. L. Martin, K. Morokuma, V. G. Zakrzewski, G. A. Voth, P. Salvador, J. J. Dannenberg, S. Dapprich, A. D. Daniels, O. Farkas, J. B. Foresman, J. V. Ortiz, J. Cioslowski and D. J. Fox, Gaussian 09 Revision D.01, Gaussian Inc, Wallingford CT, USA, 2009 Search PubMed.
- T. Bruhn, A. Schaumlffl, A. Schaumlffl, Y. Hemberger, Y. Hemberger, G. Bringmann and G. Bringmann, SpecDis: quantifying the comparison of calculated and experimental electronic circular dichroism spectra, Chirality, 2013, 25, 243–249 CrossRef CAS PubMed.
Footnotes |
† Electronic supplementary information (ESI) available: 1D and 2D NMR spectra, HRESIMS, UV, IR, and OR spectra of new compounds 1 and 2, and ECD data of 1. See DOI: 10.1039/c8ra00291f |
‡ Yin Nian, Ni-Hong Lu, and Xiao-Ling Liu contributed equally. |
|
This journal is © The Royal Society of Chemistry 2018 |