DOI:
10.1039/C7RA13237A
(Paper)
RSC Adv., 2018,
8, 9941-9945
β-Carotene: a natural osteogen to fabricate osteoinductive electrospun scaffolds†
Received
11th December 2017
, Accepted 26th February 2018
First published on 12th March 2018
Abstract
β-Carotene (βC) as a natural osteogenic material was incorporated in PCL electrospun mats to fabricate scaffolds for bone tissue engineering. These scaffolds successfully supported the attachment and proliferation of mesenchymal stem cells (MSCs). Seeded scaffolds were calcinated during 21 days of cell culture in a non-differential medium, which showed the osteodifferentiation of MSCs. Expression of RUNX2, SOX9, and osteonectin proved the osteoinductive effect of incorporated β-carotene on the differentiation of MSCs to osteoblasts without using any external osteogenic differential agent. However, the cells did not pass the early phase of osteogenesis and were still osteochondro-progenitor after 21 days of incubation. Thus, the fabricated fibrous scaffolds are potential candidates for direct bone tissue engineering.
Introduction
Bone fractures caused by osteoporosis affect about one-third of women and one-fifth of men aged over 50 years in the world1 and are the main reason for disability and suffering in the elderly population. Women, particularly postmenopausal women, are extremely vulnerable to osteoporosis.2 Despite the healability of bone tissue, restoration of some fractures and damage through normal physiological processes is very slow, particularly when the defect size is greater than the healing capacity of bone tissue.3 Thus, bone tissue engineering has attracted increasing interest for constructing biological substitutes that allow growth and proliferation of host cells in injured and diseased bone to restore and maintain normal function.4–7
Mesenchymal stem cells (MSCs), as a main source of adult stem cells, have found numerous applications in tissue engineering and cell therapy due to their ability to self-reproduce and differentiate into a range of cells and tissues such as bone, cartilage, muscle, tendon, ligament, and fat.8–11 MSCs have been the focus of recent studies in bone tissue engineering, in which they are seeded in a scaffold and induced to generate new osteogenic cells via osteoinductive cues.12–14 Appropriate bioactive cues encapsulated within the scaffolds can not only regulate cell adhesion and proliferation, but also enhance osteogenic differentiation. For example, incorporation of hydroxyapatite (HA) into the formulations of scaffolds enhanced the osteoinductivity15,16 since bone is primarily composed of HA17. Furthermore, encapsulation of drugs such as dexamethasone,18,19 simvastatin,20 bone morphogenetic proteins21 and ascorbate-2-phosphate19 within scaffolds and their sustained release improved the osteogenic differentiation of seeded MSCs.
β-Carotene (βC) is the most abundant precursor of vitamin A in the human diet and found in dark-green and orange fruits and vegetables.22,23 It is a natural bioactive component with electrical activity24,25 due to its conjugated double bonds (Fig. 1a). It can reduce bone loss in elder adults.26,27 The ability of β-carotene to induce osteogenic differentiation of stem cells has been reported.28–30 Although there are some recent articles about encapsulation of β-carotene within electrospun fibers,31–33 based on our literature survey, no research has been reported on the incorporation of β-carotene within electrospun scaffolds for tissue engineering applications.
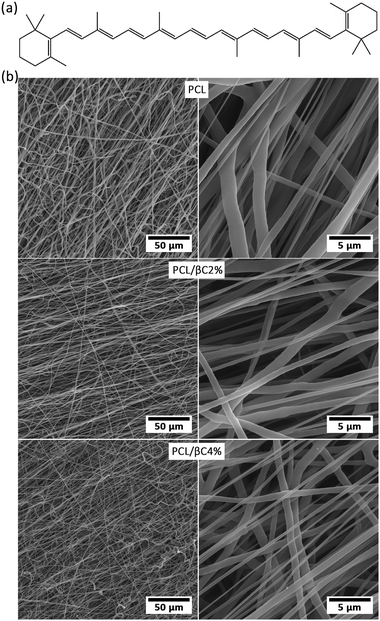 |
| Fig. 1 (a) The molecular structure of β-carotene. (b) SEM images of the fabricated random fibrous mats. | |
This research aims to fabricate PCL fibrous mats containing β-carotene as bone tissue engineering scaffolds with the ability to self-differentiate MSCs to osteoblasts without using any osteogenic differential agent. Polycaprolactone (PCL) was chosen as a polymer with high mechanical strength, lack of toxicity and low cost34–36 for fabricating scaffolds via an electrospinning process. First, the morphology and biocompatibility of the mats for bone tissue engineering were evaluated and then, their performance in simulating the appropriate environment for the differentiation of MSCs to osteoblasts was studied.
Experimental
All experimental details, including materials, electrospinning process, instruments, and biological assays, are provided in the electronic supplementary information (ESI†).
Results and discussion
Fabrication of scaffolds
Electrospinning is a proficient technique for the fabrication of a porous substrate with high surface area, similar to extracellular matrix (ECM); such a porous substrate is suitable for cell attachment and proliferation.18,37 PCL fibrous mats containing β-carotene (0, 2 and 4 wt%, called PCL, PCL/βC2% and PCL/βC4%, respectively) were prepared via electrospinning process. SEM images (Fig. 1b) show that the fabricated mats consisted of randomly oriented fibers with an average diameter of 1.64 ± 1.42, 2.52 ± 1.49 and 1.87 ± 0.98 μm. The pore size of mats was 6.45, 5.15 and 2.34 μm, respectively. The porosity of the mats was 49.3, 52.8 and 48.2%. The mats contained large interconnected cavities, which are well-suited to provide the appropriate biological conditions for guiding MSCs to osteoblasts.
The chemical structure of the electrospun mats was investigated by FTIR spectroscopy (Fig. S1–S3 in ESI†). The FTIR spectra of all PCL mats were the same as that of virgin PCL, which indicated that no degradation or decomposition occurred during the electrospinning process. Due to the low content of β-carotene, no corresponding peak was observed in the FTIR spectra of the mats.
Biocompatibility
Scaffolds designed for tissue engineering should be biocompatible, so that cells can attach to these scaffolds and proliferate on them. Thus, the viability of MSCs (104 cell per well) on the scaffolds (0.5 × 0.5 cm2) after 24, 48, and 72 hours was evaluated. Optical microscope images (Fig. 2a) display that spindle-shaped MSCs have proliferated and moved toward the scaffold. The MTT results (Fig. 2b) indicate no cytotoxicity effects for the scaffolds up to 72 hours; the cell viability of MSCs considerably improved (p < 0.001) on the third day. Incorporation of β-carotene within mats did not significantly change (p > 0.05) the viability of seeded MSCs. Moreover, the cell proliferation on the scaffolds was similar to that on the tissue culture plate used as the control.
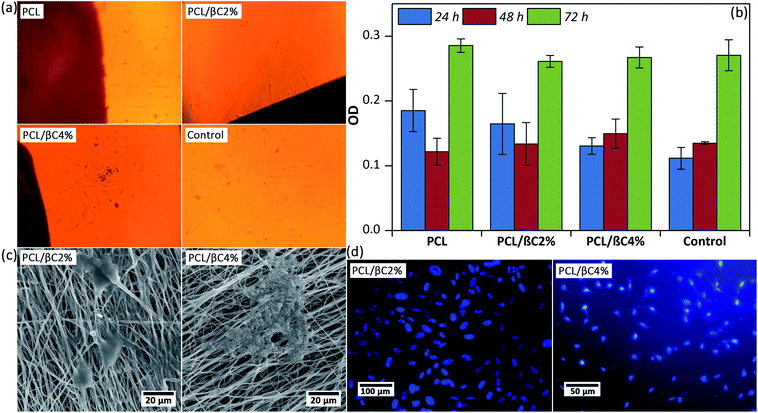 |
| Fig. 2 (a) Optical microscopic images of MSCs (104 cells per well) on the scaffolds (0.5 × 0.5 cm2) after 24 hours. Tissue culture plate was used as the control. The magnification is 100. (b) The viability of MSCs on the scaffolds up to 76 hours obtained by MTT assay. (c) SEM images of the MSCs attached on the scaffolds after 48 hours. (d) DAPI staining images of the cell nucleus on the scaffolds after 48 hours. | |
A good attachment of MSCs on the scaffold after 48 hours was observed in SEM images (Fig. 2c), in which well-developed cell–cell and cell–matrix interactions are observed. DAPI assay was used after 48 hours to evaluate the adhesion of seeded MSCs with healthy nuclei. These results confirmed that the fabricated scaffolds are biocompatible and can support the attachment and proliferation of MSCs.
Osteoblast differentiation
Recent reports demonstrated the osteogenic effect of retinoic acid38 and β-carotene2,28–30 on cells. Nishide et al.28 studied the role of carotenoids in suppressing osteoclastogenesis. They activate cell signaling via binding to nuclear retinoic acid receptors (RARs). RARs attach to DNA as heterodimers with the retinoid X receptors (RXRs) and consequently modulate retinoic acid-responsive target genes.28,39–41 Wang et al.2 reported that β-carotene exerted an inhibitory effect on osteoclastogenesis and resorption pit formation through the nuclear factor-kappa B (NF-κB) pathway. To study the osteoinductive effect of β-carotene-containing mats on the differentiation of MSCs, scaffolds (PCL/βC2% and PCL/βC4%, 0.5 × 0.5 cm2) were seeded with cells (104 cell per well) and kept in DMEM with 10% FBS as a non-differential medium for up to 21 days. For comparison, L-ascorbic acid (10 mM) and dexamethasone (1 mM) as external osteogenic differential agents15 were added to the cell culture medium used for the pure PCL scaffold. Optical microscopy images (Fig. 3a) shows the morphology change in osteodifferentiated cells on PCL/βC2% and PCL/βC4% scaffolds after 21 days as compared to that on the tissue culture plate used as the control. A similar change was observed for cells on pure PCL scaffold cultured in the presence of external differential agents.
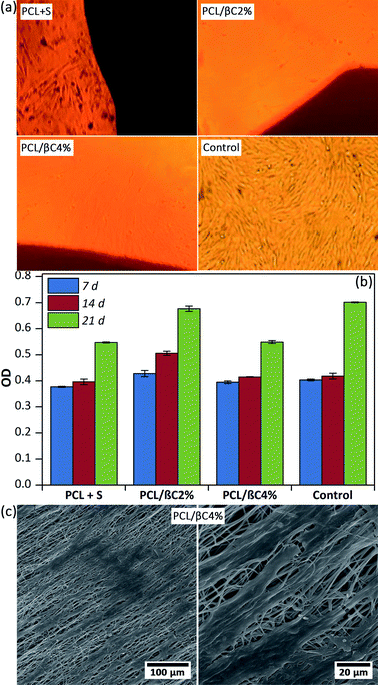 |
| Fig. 3 (a) Optical microscopic images of differentiated cells (104 cell per well) on the scaffolds (0.5 × 0.5 cm2) after 21 days. Tissue culture plate was used as the control. S was L-ascorbic acid (10 mM) and dexamethasone (1 mM). The magnification is 100. (b) The viability of the cells on the scaffolds up to 21 days obtained by MTT assay. (c) SEM images of the cells on PCL/βC4% scaffold after 14 days. | |
The viability of cells on scaffolds during 21 days culture was evaluated by MTT assay (Fig. 3b). The number of cells on the scaffolds increased for up to 21 days, confirming their metabolic activity during this period. The viability of cells on PCL/βC4% scaffold significantly decreased during the third week (p < 0.001) as compared to that on PCL/βC2% scaffold and the control; this can be attributed to the differentiation of MSCs to osteoblasts. SEM images (Fig. 3c) show that the morphology of seeded MSCs on PCL/βC4% completely changed to osteoblast after 14 days.
Alizarin red staining was performed to qualitatively evaluate the formation of mineralized matrix on the scaffolds. The results indicated the absorption of alizarin red dye on β-carotene containing scaffolds, particularly PCL/βC4%, due to the calcium deposed by osteodifferentiated cells (Fig. 4a). The intensity of absorbed dye increased over 21 days, indicating the progress of osteodifferentiation of cells during this period.
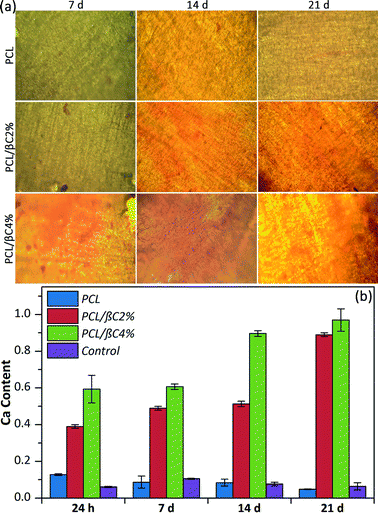 |
| Fig. 4 (a) Alizarin red staining of the scaffolds seeded with MSCs cells (104 cells per well). The magnification is 200. (b) Calcium content of the scaffolds seeded with MSCs. Tissue culture plate was used as the control. | |
The quantity of calcium generated by the osteodifferentiated cells on scaffolds was measured via calcium content assay (Fig. 4b). The calcium content on scaffolds containing β-carotene increased over 21 days without using any external osteogenic differential agents, demonstrating the osteogenic osteoinductive effect of incorporated β-carotene for seeded MSCs. Moreover, low calcium content was detected for pure PCL scaffold, similar to the tissue culture plate, showing no differentiation of MSCs to osteoblasts.
The osteoinductivity of β-carotene within scaffolds for MSCs was assessed by evaluating the expression of RUNX2 and SOX9 genes in cells through reverse transcription polymerase chain reaction (RT-PCR). To this end, the total RNA of the cells was isolated and converted into cDNA by reverse transcriptase enzyme and polymerase chain reaction was then performed on the cDNA. The expression of β2M was recorded as a control gene. RUNX2 is the most important gene for following the osteogenic differentiation of MSCs.42 RT-PCR data (Fig. 5a) show the expression of RUNX2 (band at 81 bp) for both PCL/βC2% and PCL/βC4% scaffolds after 7 and 14 days, confirming the osteoinductive effect of β-carotene within scaffolds on the differentiation of seeded MSCs. Nishide et al.28 also reported that β-carotene increased RUNX2 expression in the MC3T3-E1 cell line. SOX9 is another gene expressed only in the early phase of osteogenesis; thus, when the cells pass this phase, SOX9 will no longer be expressed.42 RT-PCR data (Fig. 5a) display the expression of SOX9 (band at 74 bp) for both PCL/βC2% and PCL/βC4% scaffolds even after 14 days, which indicates that the cells did not pass the early phase of osteogenesis and were still osteochondro-progenitor.
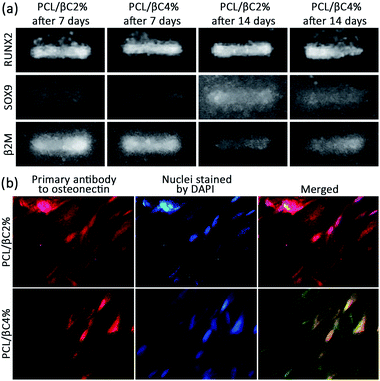 |
| Fig. 5 (a) RT-PCR results for expression of RUNX2 and SOX9 of osteodifferentiated cells (106 cells per well) on scaffolds (0.5 × 0.5 cm2). The expression of β2M was recorded as a control gene. (b) ICC results for expression of osteonectin of osteodifferentiated cells on scaffolds after 21 days. The magnification is 400. | |
Finally, the expression of osteonectin gene, a differentiation marker for bone cells,43 was evaluated to approve the osteogenic differentiation of MSCs on PCL/βC2% and PCL/βC4% scaffolds. For this purpose, immunocytochemistry (ICC) assay was performed on cells seeded on scaffolds for 21 days. The fluorescence microscopic images (Fig. 5b) demonstrate the expression of osteonectin in a large number of cells, confirming the differentiation of seeded MSCs to osteoblasts as a result of the osteoinductive effect of incorporated β-carotene without any external differential agent.
It is worth mentioning that no degradation was observed in the PCL scaffolds during 21 days immersion in growth medium. Therefore, β-carotene should be released from the mats through a diffusion-out phenomenon before expressing its osteoinductive effect on the MSCs.
Conclusion
The β-carotene-containing fibrous mats can be used as an excellent scaffold for bone tissue engineering to provide an appropriate environment for the osteodifferentiation of MSCs. The as-fabricated scaffolds were biocompatible and support the attachment and proliferation of seeded MSCs. Calcium content and ICC results confirmed the osteoinductive effect of β-carotene incorporated in the scaffolds on the differentiation of MSCs to osteoblasts without using any external osteogenic differential agent. However, RT-PCR results demonstrated that the cells did not pass the early phase of osteogenesis and were still osteochondro-progenitor after 21 days incubation.
Conflicts of interest
There are no conflicts of interest to declare.
Acknowledgements
Authors gratefully acknowledge the financial support for this study by Iran National Science Foundation (INSF, Project no. 95829362).
References
- O. Johnell and J. A. Kanis, Osteoporosis Int., 2006, 17, 1726–1733 CrossRef CAS PubMed
. - F. Wang, N. Wang, Y. Gao, Z. Zhou, W. Liu, C. Pan, P. Yin, X. Yu and M. Tang, Life Sci., 2017, 174, 15–20 CrossRef CAS PubMed
. - Y. Liu, G. Wu and K. de Groot, J. R. Soc., Interface, 2010, 7, S631–S647 CrossRef CAS PubMed
. - W. Jun, W. Peng, J. Dianming, L. Hong, L. Cong, L. Xing, Q. Xiangyang, C. Yujiang and L. Ming, RSC Adv., 2017, 7, 54306–54312 RSC
. - L. Tao, L. Zhonglong, X. Ming, Y. Zezheng, L. Zhiyuan, Z. Xiaojun and W. Jinwu, RSC Adv., 2017, 7, 54100–54110 RSC
. - A.-M. Yousefi, P. F. James, R. Akbarzadeh, A. Subramanian, C. Flavin and H. Oudadesse, Stem Cells Int., 2016, 2016, 6180487 CrossRef PubMed
. - A. R. Amini, C. T. Laurencin and S. P. Nukavarapu, Critical Reviews in Biomedical Engineering, 2012, 40, 363–408 CrossRef PubMed
. - C.-W. Kao, P.-H. Cheng, P.-T. Wu, S.-W. Wang, I. C. Chen, N.-C. Cheng, K.-C. Yang and J. Yu, RSC Adv., 2017, 7, 51343–51351 RSC
. - Z. Hao, Y. Ma, J. Wu, X. Li, H. Chen, J. Shen and H. Wang, RSC Adv., 2017, 7, 50200–50209 RSC
. - I. Pountos, D. Corscadden, P. Emery and P. V. Giannoudis, Injury, 2007, 38, S23–S33 CrossRef PubMed
. - D. Baksh, L. Song and R. S. Tuan, J. Cell. Mol. Med., 2004, 8, 301–316 CrossRef CAS PubMed
. - N. Zhao, Y. Wang, L. Qin, Z. Guo and D. Li, RSC Adv., 2017, 7, 43186–43196 RSC
. - Z. Mao, Z. Fang, Y. Yang, X. Chen, Y. Wang, J. Kang, X. Qu, W. Yuan and K. Dai, RSC Adv., 2017, 7, 24607–24615 RSC
. - W. L. Grayson, S. Bhumiratana, C. Cannizzaro and G. Vunjak-Novakovic, in Mesenchymal Stem Cell Assays and Applications, Methods in Molecular Biology (Methods and Protocols), ed. M. Vemuri, L. Chase and M. Rao, Humana Press, 2011, vol. 698, pp. 231–241 Search PubMed
. - B. Chuenjitkuntaworn, T. Osathanon, N. Nowwarote, P. Supaphol and P. Pavasant, J. Biomed. Mater. Res., 2016, 104, 264–271 CrossRef PubMed
. - J. Qian, W. Xu, X. Yong, X. Jin and W. Zhang, Mater. Sci. Eng., Proc. Conf., 2014, 36, 95–101 CrossRef CAS PubMed
. - B. C. Isenberg and J. Y. Wong, Mater. Today, 2006, 9, 54–60 CrossRef CAS
. - A. K. Gaharwar, S. M. Mihaila, A. A. Kulkarni, A. Patel, A. D. Luca, R. L. Reis, M. E. Gomes, C. van Blitterswijk, L. Moroni and A. Khademhosseini, J. Controlled Release, 2014, 187, 66–73 CrossRef CAS PubMed
. - H. Kim, H. W. Kim and H. Suh, Biomaterials, 2003, 24, 4671–4679 CrossRef CAS PubMed
. - J. B. Lee, J. E. Kim, D. A. Balikov, M. S. Bae, D. N. Heo, D. Lee, H. J. Rim, D.-W. Lee, H.-J. Sung and I. K. Kwon, Macromol. Biosci., 2016, 16, 1027–1103 CrossRef CAS PubMed
. - C. Li, C. Vepari, H.-J. Jin, H. J. Kim and D. L. Kaplan, Biomaterials, 2006, 27, 3115–3124 CrossRef CAS PubMed
. - R. S. Parker, FASEB J., 1996, 10, 542–551 CrossRef CAS PubMed
. - W. Miki, Pure Appl. Chem., 1991, 63, 141–146 CrossRef CAS
. - A. Masek, E. Chrzescijanska, K. Diakowska and M. Zaborski, Int. J. Electrochem. Sci., 2015, 10, 3372–3386 CAS
. - G. Leatherman, E. N. Durantini, D. Gust, T. A. Moore, A. L. Moore, S. Stone, Z. Zhou, P. Rez, Y. Z. Liu and S. M. Lindsay, J. Phys. Chem. B, 1999, 20, 4006–4010 CrossRef
. - A.-M. Wu, C.-Q. Huang, Z.-K. Lin, N.-F. Tian, W.-F. Ni, X.-Y. Wang, H.-Z. Xu and Y.-L. Ch, J. Bone Miner. Res., 2014, 29, 2032–2039 CrossRef CAS PubMed
. - M. Sugiura, M. Nakamura, K. Ogawa, Y. Ikoma and M. Yano, PLoS One, 2012, 7, e52643 CAS
. - Y. Nishide, Y. Tousen, M. Tadaishi, M. Inada, C. Miyaura, M. C. Kruger and Y. Ishimi, Int. J. Environ. Res. Public Health, 2015, 12, 13750–13761 CrossRef CAS PubMed
. - M. Tadaishi, Y. Nishide, Y. Tousen, M. C. Kruger and Y. Ishimi, J. Clin. Biochem. Nutr., 2014, 54, 109–115 CrossRef CAS PubMed
. - C. K. Park, Y. Ishimi, M. Ohmura, M. Yamaguchi and S. Ikegami, J. Nutr. Sci. Vitaminol., 1997, 43, 281–296 CrossRef CAS PubMed
. - D. Semnani, M. Nasari and A. Fakhrali, Polym. Bull., 2018 DOI:10.1007/s00289-017-2141-9
. - R. P. Reksamunandar, D. Edikresnha, M. M. Munir, S. Damayanti and Khairurrijala, Procedia Eng., 2017, 170, 19–23 CrossRef CAS
. - I. Peinado, M. Mason, A. Romano, F. Biasioli and M. Scampicchio, Appl. Surf. Sci., 2016, 370, 111–116 CrossRef CAS
. - A. Huang, Y. Jiang, B. Napiwocki, H. Mi, X. Peng and L.-S. Turng, RSC Adv., 2017, 7, 43432–43444 RSC
. - C. Zhou, X. Zhou and X. Su, RSC Adv., 2017, 7, 39718–39725 RSC
. - L. D. Shea, D. Wang, R. T. Franceschi and D. J. Mooney, Tissue Eng., Part A, 2000, 6, 605–617 CrossRef CAS PubMed
. - W. J. Li, C. T. Laurencin, E. J. Caterson, R. S. Tuan and F. K. Ko, J. Biomed. Mater. Res., 2002, 60, 613–621 CrossRef CAS PubMed
. - D. Mattinzoli, P. Messa, A. Corbelli, M. Ikehata, C. Zennaro, S. Armelloni, M. Li, L. Giardino and M. P. Rastaldi, Eur. Cells Mater., 2012, 24, 403–425 CrossRef CAS PubMed
. - A. C. Green, T. J. Martin and L. E. Purton, J. Steroid Biochem. Mol. Biol., 2016, 155, 135–146 CrossRef CAS PubMed
. - M. Yamaguchi, J. Biomed. Sci., 2012, 19, 36 CrossRef CAS PubMed
. - S. A. Ross, P. J. McCaffery, U. C. Drager and L. M. De Luca, Physiol. Rev., 2000, 80, 1021–1054 CrossRef CAS PubMed
. - N. Fratzl-Zelman and F. Varga, in Principles of Bone and Joint Research, ed. P. Pietschmann, Springer, Switzerland, 2017, pp. 17–31 Search PubMed
. - G. Jundt, K.-H. Berghäuser, J. D. Termine and A. Schulz, Cell Tissue Res., 1987, 248, 409–415 CrossRef CAS PubMed
.
Footnote |
† Electronic supplementary information (ESI) available: Experimental details and FTIR spectra. See DOI: 10.1039/c7ra13237a |
|
This journal is © The Royal Society of Chemistry 2018 |
Click here to see how this site uses Cookies. View our privacy policy here.