DOI:
10.1039/C7RA13231J
(Paper)
RSC Adv., 2018,
8, 15698-15702
84% efficiency improvement in all-inorganic perovskite light-emitting diodes assisted by a phosphorescent material†
Received
11th December 2017
, Accepted 9th April 2018
First published on 26th April 2018
Abstract
A novel mixed perovskite emitter layer is applied to design all-inorganic cesium lead halide perovskite light-emitting diodes (PeLEDs) with high electroluminescence (EL) performance, by combining CsPbBr3 with iridium(III)bis[2-(4′,6′-difluorophenyl)pyridinato-N,C2′]-picolinate (FIrpic), where FIrpic is a phosphorescent material with very high internal quantum efficiency (IQE) approaching 100%. The CsPbBr3:FIrpic PeLEDs show a maximum luminance of 5486 cd m−2, and an external quantum efficiency of 0.47%, which are 1.84 and 1.76 times that of neat CsPbBr3 PeLEDs, respectively. It is found that FIrpic molecules as an assistant dopant can efficiently transmit energy from the excitons of FIrpic to the excited state of the CsPbBr3 emitter via a Förster energy transfer process, leading to enhanced EL efficiency in the CsPbBr3:FIrpic PeLEDs.
1. Introduction
In recent years, all-inorganic halide perovskite light-emitting diodes (PeLEDs) have attracted a lot of attention due to their high photoluminescence (PL) quantum yield, high color purity, better thermal stability and higher charge-carrier mobility than organic–inorganic perovskites.1–4 The first perovskite light-emitting diode observed at room-temperature was reported by R. H. Friend's group in 2014,5 showing the highest luminescence of 364 cd m−2, external quantum efficiency (EQE) of 0.1% for green PeLEDs, and a tunable luminescence spectrum. And Yantara et al. presented the first CsPbBr3 LED, with enhanced maximum luminescence (407 cd m−2), but the current efficiency (CE, 0.035 cd A−1) and external quantum efficiency (EQE, 0.008%) were still low.6 To improve the performance, much work has focused on obtaining complete surface coverage, efficient charge injection, and better carrier transporting balance, such as interfacial engineering,7–11 crosslinking method,2 polymer-assisted method,12,13 small organic molecule-assisted method.14–16 And many researchers have achieved excellent PeLEDs performance by doping emitter materials (such as poly(ethylene glycol) (PEO)),12,13,17 hole transporting materials (such as 1,3-bis(9-carbazolyl) benzene (mCP)),14 electron transporting materials (such as 1,3,5-tris(1-phenyl-1H-benzimidazol-2-yl)benzene (TPBi)),15 1,3,5-tri(m-pyridin-3-yl-phenyl)benzene (TmPyPB),16 and bipolar transporting materials (such as PVK:TPBi),18 which can be attributed to the improvement of the film coverage, the charge injection and the efficient energy transfer of singlet excitons from the assistant dopants to the excited state of the perovskite emitter. However, the triplet excitons in these PeLEDs are wasted, since all the triplet excitons would decay non-radiatively due to the spin-forbidden of triplet exciton transition in fluorescent emitters.19 Usually, to break the limitation in internal quantum efficiency of fluorescent LEDs (<25%), heavy transition metal atoms are brought in the phosphorescent molecules to harvest both singlets and triplets due to the strong spin orbit coupling in organic light-emitting diodes (OLEDs).19–21 Thus, organotransition metal compounds have been extensively studied in efficient organic emitting diodes (OLEDs) to achieve 100% internal quantum efficiency. Iridium(III)bis[2-(4′,6′-difluorophenyl)pyridinato-N,C2′] picolinate (FIrpic), a phosphorescent material with very high internal quantum efficiency (IQE) approaching to 100%, is wildly used in OLEDs as a blue emitter and shows good EL performance.
In this work, FIrpic is adopted as an assistant to form CsPbBr3:FIrpic composite film. The PeLEDs based on this composite emissive layer can harvest both singlet and triplet excitons. And a maximum luminance of 5486 cd m−2, a maximum current efficiency of 1.80 cd A−1, and a maximum EQE of 0.47% are obtained.
2. Experimental section
2.1. Materials and perovskite precursor solution preparation
CsPbBr3 precursor solutions were made by dissolving PbBr2 (Alfa Aesar, 99.999%) and CsBr (Alfa Aesar, 99.999%) in dimethylsulfoxide (DMSO) with equimolar molar ratio at 10 wt% iridium(III)bis[2-(4′,6′-difluorophenyl)pyridinato-N,C2′] picolinate (FIrpic, >99.5%) was dissolved in DMSO at 1 mg ml−1. The two solutions were stirred for 12 hours at room temperature in a glove box, separately. Before spin-coating, the solutions were mixed with different proportions and stirred continually for 4 hours. The materials including poly(3,4-ethylenedioxythiophene):poly(p-styrene sulfonate) (PEDOT:PSS, Heraeus, Clevios AI 4083) are bought from Shanghai Han Feng Chemical Co. Ltd. 2,2′,2′′-(1,3,5-benzinetriyl)-tris(1-phenyl-1H-benzimidazole) (TPBi, >99%), 8-hydroxyquinolinato lithium (Liq, >99%), and aluminum (Al, >99%) were purchased from Suzhou Fangsheng Photoelectricity Shares Co, Ltd. All the materials used in this study were obtained commercially and used as received without further purification.
2.2. PeLEDs fabrication
Patterned indium-tin oxide (ITO) glass substrates were cleaned successively using deionized water, ethanol, and acetone, and dried in an oven at 100 °C for 10 min. After 5 min treatment with ultraviolet (UV)–ozone plasma, PEDOT:PSS was spin-coated onto ITO glass substrates at 4500 rpm for 40 s and annealed in air at 120 °C for 20 min. Then, the samples were placed in a nitrogen-filled glovebox for 30 min to cool down. The perovskite film was prepared by one-step spin-coating the perovskite precursor solution at 4000 rpm for 60 s and placed in a low vacuum environment of −1 bar for 20 min to remove residual DMSO solvent. Finally, TPBi (65 nm), Liq (2.5 nm), and Al (120 nm) were sequentially deposited by vacuum thermal depositing system, under a high vacuum (≤5 × 10−4 Pa). Preparation of the perovskite films, and encapsulation of PeLEDs were carried out in a nitrogen-filled glove box. The active area of the device was 6 mm2.
2.3. Measurements and characterizations
We used the UV-vis spectrophotometer (Shimadzu UV-2600) and Fluorolog-3 luminescence spectroscopy to measure the absorption and PL spectra, respectively. The XRD pattern and time-resolved PL spectra were acquired using a TD-3500 X-ray diffractometer and fluorescence spectrometer (Fluorolog-3) severally. The PR670 spectrophotometer was used to collect the EL spectra. The current density–luminance–voltage (J–L–V) characterizations and stability of all PeLEDs were carried out by a light-emitting diode measurement system, including a Keithley2400 source meter, and a calibrated Si photodiode (Photoelectric Instrument Factory of Beijing Normal University, ST-86LA). All measurements were carried out at room temperature under ambient conditions.
3. Results and discussion
3.1. PeLEDs performance
Using the CsPbBr3:FIrpic films as the emissive layers, bright and efficient PeLEDs are demonstrated with a typical layered architecture of ITO (120 nm)/PEDOT:PSS (30 nm)/CsPbBr3:FIrpic (X mg ml−1)/TPBi (65 nm)/Liq (2.5 nm)/Al (120 nm), where “X” are equal to 0, 0.5 for Devices A and B. The concentration optimization of FIrpic are shown in ESI Fig. S1.† The current density–voltage–luminance (J–V–L) and current efficiency–voltage–external quantum efficiency (CE–V–EQE) characteristics are shown in Fig. 1(a) and (b). Device B with FIrpic exhibits better EL performance than Device A with higher current density, luminance, current efficiency and EQE at each applied voltage, and shows maximum luminance of 5486 cd m−2, maximum current efficiency of 1.80 cd A−1, and maximum EQE of 0.47%, which are 1.84, 1.76 and 1.76 times to that of the Device A (2974 cd m−2, 1.02 cd A−1, and 0.26%), respectively. The corresponding characteristics data about the repeatability of the devices are summarized in Table S1.† The Device B emits green light from only CsPbBr3 despite of FIrpic (peaked at 470 nm)22 with high color purity of FWHM ∼18 nm and a peak intensity located at 522 nm, as shown in Fig. 1(c). In the right inset of Fig. 1(c), it could be found that the shapes of EL spectra are rarely affected by the different applied voltages. In addition, the EL spectra of each devices with different FIrpic concentrations are shown in ESI Fig. S2† and similar results are exhibited. These results reveal that the energy transfer from FIrpic to CsPbBr3 was efficient and complete. And the detailed mechanism to improve the EL performance in Device B are investigated by the following sections.
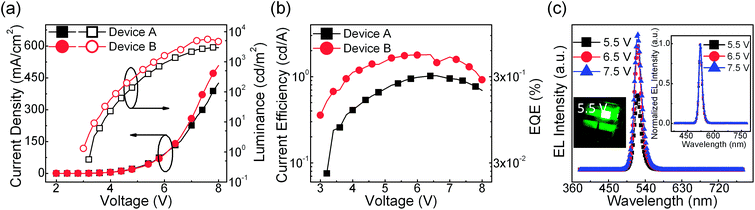 |
| Fig. 1 (a) Current density–voltage–luminance (J–V–L), (b) current efficiency–voltage–external quantum efficiency (CE–V–EQE) of Devices A (neat CsPbBr3) and B (CsPbBr3:FIrpic 0.5 mg ml−1). (c) EL intensity–wavelength curves of Device B under different driving voltages of 5.5, 6.5, and 7.5 V. The insets show an emission photo of Device B at driving voltage of 5.5 V (left inset) and the normalized EL spectra of this PeLED under different driving voltages of 5.5, 6.5, and 7.5 V (right inset). | |
3.2. Characterization of perovskite film
In order to investigate the physical mechanism of the enhanced EL performance in the PeLEDs, the XRD pattern, absorption and PL spectra, and the time-resolved PL spectra of the CsPbBr3 films FIrpic (0.5 mg ml−1) are exhibited in Fig. 2(a). In Fig. 2(a), by comparing the intensity changes of peaks at 15.7° and 31.1° assigned to the (101) and (202) planes of perovskite structure in the XRD pattern of both films, it can conclude that peaks of CsPbBr3 film with FIrpic are sharper and more intense, which demonstrate that the presence of FIrpic can induce the crystal growth along the (101) and (202) planes and may do good to the EL performance.8,10,13 And the XRD patterns match well with orthorhombic crystal structure.13 The top-view and cross-sectional SEM images of the neat CsPbBr3 film and the CsPbBr3:FIrpic film (0.5 mg ml−1) are shown in Fig. S3 and S4,† respectively. It can be found that coverage is improved in the CsPbBr3:FIrpic film. And the thickness of both the neat CsPbBr3 film and CsPbBr3:FIrpic film (0.5 mg ml−1) are estimated to ∼30 nm. UV-vis absorption and PL emission spectra of CsPbBr3 film with 0 and 0.5 mg ml−1 FIrpic are plotted in Fig. 2(b). The two kinds of films exhibit similar shapes of absorption spectra (centered at ∼518 nm) and PL spectra (peaked at ∼525 nm with a narrow FWHM of ∼18 nm). It is worthwhile mentioning that sufficient spectral overlap between the absorption spectra of the CsPbBr3 emitter and the PL spectra of the assistant dopant FIrpic, which is beneficial to energy transfer from FIrpic to CsPbBr3. And the shape of PL spectra of CsPbBr3:FIrpic film is almost same to the neat CsPbBr3 film without the sub-peak from FIrpic, which may demonstrate that the energy transfer from FIrpic to the CsPbBr3 are effective and complete. The FIrpic-doped CsPbBr3 film presents a much longer PL lifetime than that of the neat CsPbBr3 film, which is revealed by the time-resoled PL spectra (Fig. 2(c)). The average lifetime (τavg) extracted from the PL decay curve for the composite perovskite film is about 1.69 ns, while the neat CsPbBr3 has a shorter lifetime of 0.82 ns, which imply that the FIrpic additive can effectively reduce non-radiation recombination and result in enhanced the EL performance of PeLEDs. The PL curves can be fitted by the following eqn (1): |
 | (1) |
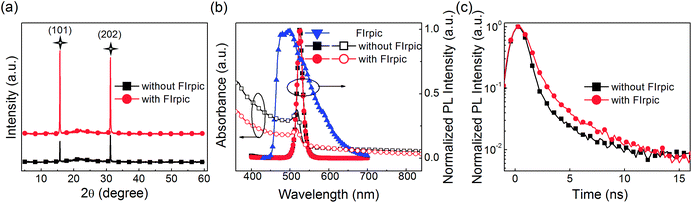 |
| Fig. 2 (a) XRD patterns, (b) UV-vis absorption and normalized PL spectra, (c) time-resolved PL spectra of CsPbBr3 film without and with FIrpic (0.5 mg ml−1). | |
The average lifetime (τavg) of the entire decay process can be calculated by the following formula (2):
|
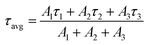 | (2) |
τ1, τ2 and τ3 are the lifetimes of the three decay components; and A1, A2, and A3 are the fractions of the three decay components, respectively. According to the study by Zheng et al.,23 the τ3 (slow) decay component is related to radiative recombination inside the grains, while the τ1 (fast) and τ2 (middle) decay components are attributed to “two kinds of trap-assisted recombination at grain boundaries.” The fitting parameters are collected in Table 1. It can be found that the proportions of τ1 and τ2 are reduced, which suggests that traps in the FIrpic assisted CsPbBr3 film are reduced, due to the effective passivation brought in by the FIrpic assistant. Moreover, the lifetime of τ3 is extended and the its proportion is improved, which suggests that the radiative recombination inside the grains are enhanced, thus leading to the better EL performance in the CsPbBr3:FIrpic based PeLEDs compared to the one without the FIrpic assistant.17
Table 1 Multi-exponential fitting parameters for PL decays both neat CsPbBr3 and CsPbBr3:FIrpic (0.5 mg ml−1) films
Films |
A1 (%) |
τ1 (ns) |
A2 (%) |
τ2 (ns) |
A3 (%) |
τ3 (ns) |
τavg (ns) |
CsPbBr3:FIrpic (0 mg ml−1) |
85.08 |
0.38 |
10.65 |
1.65 |
4.27 |
7.56 |
0.82 |
CsPbBr3:FIrpic (0.5 mg ml−1) |
78.22 |
0.64 |
16.17 |
2.76 |
5.61 |
13.23 |
1.69 |
3.3. Energy transfer process
The energy level diagram of each layer of Device B is shown in Fig. 3(a), and all energy level values are taken from the literature.14,24–26 There are two exciton generating interfaces, which are located at the PEDOT:PSS/CsPbBr3:FIrpic interface and CsPbBr3:FIrpic/TPBi interface, due to no injection barrier for electrons injected from TPBi to CsPbBr3:FIrpic film and high injection barrier for holes at PEDOT:PSS/CsPbBr3:FIrpic interface (0.6 eV) and CsPbBr3:FIrpic/TPBi interface (0.45 eV). And excitons can also be generated on FIrpic and CsPbBr3. The energy transfer diagram is described schematically in Fig. 3(b). As shown in Fig. 3(b), excitons generated in FIrpic with 25% singlets (S1) and 75% triplets (T1). And in FIrpic, the energy of S1 of FIrpic can be transfer to T1 through inter system crossing process (ISC). And the energy of both S1 and T1 of FIrpic can be transferred to the excited state of CsPbBr3 via Förster energy transfer process21 and thus leading to potentially 100% IQE in CsPbBr3:FIrpic PeLEDs.
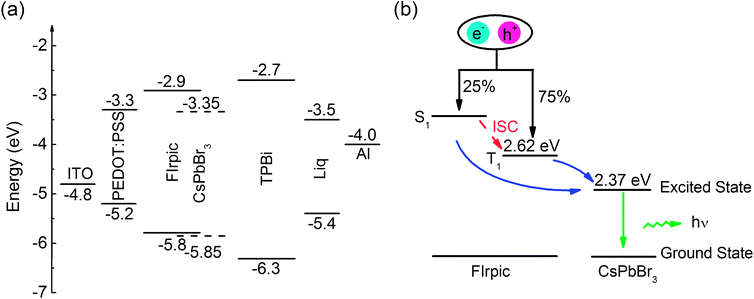 |
| Fig. 3 (a) Energy level diagram of Device B, (b) energy transfer scheme for the CsPbBr3:FIrpic emitter layer (Förster energy transfer process is indicated by blue solid arrows). | |
3.4. The stability of PeLEDs
In order to further reveal the effect of FIrpic, the half lifetimes of PeLEDs (Devices A and B) are performed and are shown in Fig. 4. The half lifetime is the time duration from the initial luminance of 100 cd m−2 decreasing to the half luminance of the initial luminance. Device B (with CsPbBr3:FIrpic film) exhibit a much longer half lifetime of 147 s, which is 1.6 times than that of Device A (87 s). The better stability of Device B may be benefit from reduced current leakage owing to the better coverage9,12,13,15 and enhanced radiative recombination inside the grain due to the reduced traps, better passivation and higher exciton harvesting efficiency in the in CsPbBr3:FIrpic film compared to that of Device A.
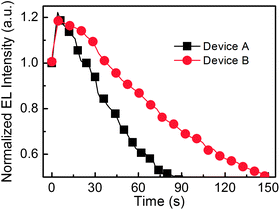 |
| Fig. 4 The stability of Device A (with a neat CsPbBr3 film) and Device B (with FIrpic-doped CsPbBr3 film). | |
4. Conclusion
In summary, the enhancements of EL performance and stability can be attributed three factors in the CsPbBr3:FIrpic PeLEDs. Firstly, the orientation of the crystallization could be remarkably enhanced by FIrpic along with the reduced traps, better passivation in the CsPbBr3:FIrpic film. Second, FIrpic improves the internal quantum efficiency in CsPbBr3:FIrpic, due to almost 100% IQE of FIrpic which can permit efficient transfer of all electrically excitons from the assistant dopant (FIrpic) to the perovskite emitter (CsPbBr3) via Förster energy transfer process. And last, the higher coverage of the CsPbBr3:FIrpic benefit to reduce current leakage. The CsPbBr3:FIrpic composite film provide a facile method to achieve highly efficient PeLEDs and a new route for advanced light emission applications.
Conflicts of interest
The authors declare no conflicts of interest.
Acknowledgements
This work is financial supported by the Natural Science Foundation of China (Grant No. 61404108, 61705150, and 11374242), and Fundamental Research Funds for the Central Universities (Grant No. XDJK2018C082), and Natural Science Foundation of Jiangsu Province (BK20160325).
References
- X. Li, Y. Wu, S. Zhang, B. Cai, Y. Gu, J. Song and H. Zeng, Adv. Funct. Mater., 2016, 26, 2435–2445 CrossRef CAS.
- G. Li, F. W. R. Rivarola, N. J. K. Davis, S. Bai, T. C. Jellicoe, F. Pena, S. Hou, C. Ducati, F. Gao, R. H. Friend, N. C. Creenham and Z. K. Tan, Adv. Mater., 2016, 28, 3528–3534 CrossRef CAS PubMed.
- Y. Wang, X. Li, J. Song, L. Xiao, H. Zeng and H. Sun, Adv. Mater., 2015, 27, 7101–7108 CrossRef CAS PubMed.
- Q. A. Akkerman, V. D'Innocenzo, S. Accornero, A. Scarpellini, A. Petrozza, M. Prato and L. Manna, J. Am. Chem. Soc., 2015, 137, 10276–10281 CrossRef CAS PubMed.
- Z. K. Tan, R. S. Moghaddam, M. L. Lai, P. Docampo, R. Higler, F. Deschler, M. Price, A. Sadhanala, L. M. Pazos, D. Credgington, F. Hanusch, T. Bein, H. J. Snaith and R. H. Friend, Nat. Nanotechnol., 2014, 9, 687–692 CrossRef CAS PubMed.
- N. Yantara, S. Bhaumik, F. Yan, D. Sabba, H. A. Dewi, N. Mathews, P. P. Boix, H. V. Demir and S. Mhaisalkar, J. Phys. Chem. Lett., 2015, 6, 4360–4364 CrossRef CAS PubMed.
- J. Wang, N. Wang, Y. Jin, J. Si, Z. K. Tan, H. Du, L. Cheng, X. Dai, S. Bai, H. He, Z. Ye, M. Ling Lai, R. H. Friend and W. Huang, Adv. Mater., 2015, 27, 2311–2316 CrossRef CAS PubMed.
- Z. Xiao, R. A. Kerner, L. Zhao, N. L. Tran, K. Min Lee, T. W. Koh, G. D. Scholes and B. P. Rand, Nat. Photonics, 2017, 11, 108–115 CrossRef CAS.
- H. Huang, H. Lin, S. V. Kershaw, A. S. Susha, W. C. Choy and A. L. Rogach, J. Phys. Chem. Lett., 2016, 7, 4398–4404 CrossRef CAS PubMed.
- X. Ji, X. Peng, Y. Lei, Z. Liu and X. Yang, Org. Electron., 2017, 43, 167–174 CrossRef CAS.
- C. F. Huang, M. L. Keshtov and F. C. Chen, ACS Appl. Mater. Interfaces, 2016, 8, 27006–27011 CAS.
- J. Li, S. G. R. Bade, X. Shan and Z. Yu, Adv. Mater., 2015, 27, 5196–5202 CrossRef CAS PubMed.
- Y. Ling, Y. Tian, X. Wang, J. C. Wang, J. M. Knox, F. Perez-Orive, Y. Du, L. Tan, K. Hanson, B. Ma and H. Gao, Adv. Mater., 2016, 28, 8983–8989 CrossRef CAS PubMed.
- Z. Y. Xiong, C. H. Gao, Z. Q. Wang, X. Y. Ma, Z. H. Xiong and P. Chen, Chin. Sci. Bull., 2017, 62, 2780–2787 Search PubMed.
- H. Cho, S. H. Jeong, M. H. Park, Y. H. Kim, C. Wolf, C. L. Lee, J. H. Heo, A. Sadhanala, N. Myoung, S. Yoo, S. Hyuk Im, R. H. Friend and T. W. Lee, Science, 2015, 350, 1222–1225 CrossRef CAS PubMed.
- F. X. Yu, Y. Zhang, Z. Y. Xiong, X. J. Ma, P. Chen, Z. H. Xiong and C. H. Gao, Org. Electron., 2017, 50, 480–484 CrossRef CAS.
- C. Wu, Y. Zou, T. Wu, M. Ban, V. Pecunia, Y. Han, Q. Liu, T. Song, S. Duhm and B. Sun, Adv. Funct. Mater., 2017, 27, 1700338 CrossRef.
- P. Chen, Z. Xiong, X. Wu, M. Shao, X. Ma, Z. H. Xiong and C. Gao, J. Phys. Chem. Lett., 2017, 8, 1810–1818 CrossRef CAS PubMed.
- M. A. Baldo, D. F. O'Brien, Y. You, A. Shoustikov, S. Sibley, M. E. Thompson and S. R. Forrest, Nature, 1998, 395, 151–154 CrossRef CAS.
- H. Yersin, Top. Curr. Chem., 2004, 241, 1–26 CrossRef CAS.
- M. A. Baldo, M. E. Thompson and S. R. Forrest, Nature, 2000, 403, 750–753 CrossRef CAS PubMed.
- H. Chen, Z. Q. Jiang, C. H. Gao, M. F. Xu, S. C. Dong, L. S. Cui, S. J. Ji and L. S. Liao, Chem.–Eur. J., 2013, 19, 11791–11797 CrossRef CAS PubMed.
- K. Zheng, K. Zidek, M. Abdellah, M. E. Messing, M. J. Al-Marri and T. Pullerits, J. Phys. Chem. C, 2016, 120, 3077–3084 CAS.
- E. Baranoff and B. F. Curchod, Dalton Trans., 2015, 44, 8318–8329 RSC.
- S. I. Yoo, J. A. Yoon, N. H. Kim, J. W. Kim, J. S. Kang, C. B. Moon and W. Y. Kim, J. Lumin., 2015, 160, 346–350 CrossRef CAS.
- V. K. Ravi, G. B. Markad and A. Nag, ACS Energy Lett., 2016, 1, 665–671 CrossRef CAS.
Footnotes |
† Electronic supplementary information (ESI) available. See DOI: 10.1039/c7ra13231j |
‡ These authors contribute equally in this work. |
|
This journal is © The Royal Society of Chemistry 2018 |
Click here to see how this site uses Cookies. View our privacy policy here.