DOI:
10.1039/C7RA13139A
(Paper)
RSC Adv., 2018,
8, 6125-6135
Effect and mechanism of oyster hydrolytic peptides on spatial learning and memory in mice
Received
8th December 2017
, Accepted 25th January 2018
First published on 7th February 2018
Abstract
Oysters (Crassostrea talienwhanensis) contain large amounts of protein and exhibit many biological activities. This study was aimed at preparing oyster protein hydrolysates (OPH) and evaluating the OPH based on a spatial learning and memory capacity. A response surface methodology was employed to optimize hydrolysis conditions to determine the OPH with the highest AChE inhibitory activity, and the optimum extraction conditions were as follows: enzyme concentration of 1444.88 U g−1, pH of 7.38, extraction temperature of 45 °C, extraction time of 5.56 h and a water/material ratio of 2.45
:
1, and the minimum acetylcholinesterase (AChE) activity was 0.069 mM min−1. The spatial memory and learning abilities and passive avoidance in mice were determined by using the Morris water maze test and a dark/light avoidance test. Furthermore, the OPH group could relieve oxidative stress, reduce AChE levels, increase choline acetyltransferase (ChAT) levels and alleviate inflammatory reaction through reduction of interleukin-1β (IL-1β), interleukin-6 (IL-6), and tumor necrosis factor-α (TNF-α) levels. Additionally, up-regulated expressions of brain-derived neurotrophic factor (BDNF) and neural cell adhesion molecules (NCAM) were observed in mice treated with OPH. These findings suggested that OPH could be a functional food candidate to improve the learning and memory ability associated with oxidative stress and inflammatory reactions.
1. Introduction
With the development of competitive pressure and working stress, more and more people experience memory loss. Additionally, the development of memory loss in adults, in conjunction with an ageing population has resulted in many people developing Alzheimer's disease (AD).1 AD is one of the most common neurodegenerative disorders affecting the elderly population worldwide, and is characterized by memory deterioration and behavior disorder.2,3 As of 2010, there were more than 36 million people worldwide suffering from AD.4 By 2050, the prevalence will quadruple, by which time 1 in 85 persons worldwide will be living with the disease.5 It is likely that behavioral, dietary and other environmental factors may affect the possibility of developing AD. However, most research has not yet matured to a point where clear recommendations can be made,6 and in recent years, the prevalence of AD has exponentially increased.7 Although five drugs are approved in the United States for the treatment of AD include tacrine, rivastigmine, donepezil, galantamine and memantine, their use in humans may be compromised by side effects, such as hepatotoxicity and excitotoxicity.8 Currently, the demand for finding alternative therapeutic agents to synthetic chemicals, such as natural products, is increasing because they are generally regarded as safer than their synthetic counterparts.9
Some researchers have found that the higher memory capacity a person has, the slower the decline in his or her cognitive ability during the early stage of AD.10 In recent years, researchers have focused on the impairment of learning and memory. Despite the research, the most effective approach and the precise underlying mechanism for memory-enhancing effects remained unknown.11 Growing evidences shows that oxidative stress resulting from reactive oxygen species (ROS) seriously affects AD animals and patients.12,13 Other hypothesis showed that inflammation clearly occurs in the AD-affected brain,14 and neuroinflammation is now recognized as a prominent feature in the pathology of Alzheimer's, making it a potential target for disease therapy and prevention.15 Furthermore, the current strategy of AD intervention is mainly to ameliorate the cognitive symptoms related to acetylcholine (ACh) depletion, thereby enhancing the central cholinergic neurotransmission by reversing acetylcholinesterase (AChE) inhibition.16 Experimental studies suggest ACh plays a vital role in learning and memory.17 In addition, some researchers have studied interrelated physiological indicators in mice to evaluate the effect of products in improving the memory. Behavioral tests, such as the Morris water maze, dark/light avoidance and other passive avoidance tests are used to evaluate the effects of treatment on learning and memory ability in animal models known to be sensitive to hippocampal-dependent learning and memory deficits.9,18
Oysters are excellent sources of high-quality nutrition in Northeast China and in other parts of the world.19 The oyster species, Crassostrea talienwhanensis, is rich in protein, low in fat and is a good source of several vitamins and minerals. C. talienwhanensis is used to study a variety of biological activities, including antioxidant activity,19 antihypertensive activity,20 anti-bacterial activity,21 antitumor activity22 and anti-inflammatory activity.23 Additionally, Lin et al.24 had researched the enzymatic hydrolysates from oyster protein on the ability of learning memory in mice, and found the hydrolysates had remarkable antioxidant activity in vivo, however, the mechanisms of the oyster protein hydrolysates (OPH) on the learning and memory capacity needed fully explored. With this idea in mind, we prepared OPH by using AChE activity in the astrocyte as a test model in vitro. The Morris water maze and dark/light avoidance tests, along with a series of biochemical indicators determinations were performed on mice in vivo to explore the relationship of spatial learning and memory mechanism with antioxidative stress and anti-inflammatory activity.
2. Materials and methods
2.1 Materials
Oyster (Crassostrea talienwhanensis) was purchased from a seafood market in Qingdao, China. Upon arrival, the oyster was washed, and the edible meat was separated from the shells, homogenized and stored at −20 °C until use.
Five proteases (compound proteinase, trypsin, papain, neutrase and flavourzyme) were purchased from Beijing Solarbio Science & Technology Co., Ltd (Beijing, China); 1,1-diphenyl-2-picryhydrazyl (DPPH) and phenazine methosulfate (PMS) were purchased from Sigma Chemical Co. (St Louis, MO, USA); Nicotinamide-adenine dinucleotide (NADH) and nitroblue tetrazolium (NBT) were purchased from Ruitaibio Co (Beijing, China). The Medical College of Qingdao University (Qingdao, China) provided astrocytes. The growth medium and antibiotics for the cell culture experiments were purchased from Hyclone (USA). Fetal bovine serum was purchased from Gibco (Australia). All other chemicals and solvents were of analytical grade.
2.2 Methods
2.2.1 Preparation of OPH. Hydrolysis was performed by first mixing oyster homogenate with deionized water at a ratio of 1
:
5 (w/v). The mixtures were adjusted to the required pH with 0.01 mol L−1 NaOH or HCl, and heated in a water bath to the required temperature before the five proteases were added in proper proportion based on activity. Next, the hydrolysis reactions were carried out in a shaking incubator. At the end of the hydrolysis period, the mixtures were heated in boiling water for 10 minutes to inactivate the protease. Following inactivation, the hydrolysates were centrifuged at 10
000 × g (4 °C) for 15 minutes, and the supernatants were spray-dried to obtain OPH, which was saved for further study. Among the five hydrolysates, the one with the highest antioxidant activity and AChE inhibition rate was chosen for further optimization according to single factor experiment and response surface methodology (RSM).
2.2.2 AChE activity assays. As the most widely distributed type of cell in the mammalian brain, astrocytes are important players in neurological disorders and participate and modulate neuronal communication. AChE has critical catalytic function in cholinergic synapses and is also present in astrocytes. In this study, astrocytes were cultured in DMEM/F12 medium containing 10% fetal bovine serum and 1% antibiotic and antimycotic solution, and incubated. The medium was changed every two days.Astrocytes were seeded in 24-well plates at a final concentration of 1 × 105 to 106 cells per mL, and 0.5 mL OPH (10 mg mL−1) was added for 24 hours. After the removal of culture medium, 0.5 mL phosphate buffer (0.01 M, pH 7.2) was added in 24-well plates and the astrocytes were ground with a grinding rod.
Finally, the phosphate buffer was centrifuged at 5000 × g (4 °C) for 10 minutes, and the supernatant was used for testing AChE activity using an AChE kit.
2.2.3 Determination of soluble peptide content. The content of soluble peptides was determined by the biuret method according to our previous report.25 To complete this method, 4.0 mL biuret reagent was added to a 1.0 mL sample. The mixture was shaken and left to stand for 30 minutes, and then the absorbance was measured at 540 nm. The biuret reagent was used as the control and BSA was used as the standards. All experiments were performed in triplicate.
2.2.4 Scavenging activity on hydroxyl radical. Scavenging activity of OPH on hydroxyl radicals was performed, using method described by Smeriglio et al.26 with a few modifications. Briefly, the reaction mixture contained 1.0 mL of phosphate buffer (PBS, 0.15 mol L−1, pH 7.4), 1.0 mL of safranine T (1.0 mM), 0.5 mL of EDTA–FeSO4 (2.0 mmol L−1) and 1.0 mL of OPH with the concentration of 5 mg mL−1, the same concentration of vitamin C (Vc) was used as standard. After sufficient mixing, 1.0 mL of H2O2 (3%) was added to the mixture. Following incubation at 37 °C for 30 min, the absorbance of the mixture was measured at 520 nm. The hydroxyl radical scavenging activity was calculated as: scavenging rate (%) = [(A1 − A0)/(A2 − A0)] × 100, where A1 was the absorbance of OPH, A2 was the absorbance without H2O2, A0 was the absorbance of the control. Both A0 and A2 were the mixtures with sample solution replaced by deionized water. All experiments were performed in triplicate.
2.2.5 Scavenging activity on DPPH radical. The DPPH radical scavenging activity of the OPH was determined as described by Blois27 with slight modifications. Briefly, 1.0 mL of DPPH (0.1 mmol L−1) diluted in ethanol was added to 3.0 mL of OPH with the concentration of 5 mg mL−1, the same concentration of Vc was used as standard. After vigorous shaking, the mixture was left to stand for 30 min and the absorbance was measured at 517 nm. The DPPH radical scavenging activity was calculated as follows: scavenging rate (%) = [1 − (A1 − A0)/(A2 − A0)] × 100, where A0 was the absorbance without DPPH, A1 was the absorbance in the presence of the OPH, and A2 was the absorbance of the control (without sample). All experiments were performed in triplicate.
2.2.6 Scavenging activity on superoxide radical. The superoxide radical scavenging activity of OPH was assessed based on the method of Nishikimi et al.28 with a slight modification. The reaction mixture, containing OPH with the final concentration of 5 mg mL−1, 0.5 mL PMS (20 mol L−1), 0.5 mL NADH (240 mol L−1) and 0.5 mL NBT (150 mol L−1) in 3.0 mL Tris–HCl buffer (0.1 mol L−1, pH 7.4), was incubated at room temperature for 5 min and the absorbance was read at 560 nm against a blank. The same concentration of Vc was used as standard. The capability of scavenging to superoxide radical was calculated using the following equation: scavenging effect (%) = [1 − (A1/A0)] × 100, where A1 was the absorbance in the presence of the sample, and A0 was the absorbance of the control. All experiments were carried out in triplicate.
2.2.7 Animal test. One hundred and twenty Kunming male mice (18–22 g, approval no. SCXK 2012-003) were provided by the Experimental Animal Center of Xi'an Jiaotong University Health Science Center. Four-week old mice were allowed to adapt to their surroundings for one week before starting the experiments, and were housed in stainless steel cages (ten mice per cage) at room temperature (20–26 °C), with humidity ranging from 40 to 60%, and a 12/12 hour light–dark cycle. The mice had free access to water and a standard rodent diet. After adaptation, the mice were divided into two parts, each part contained five groups, and there were twelve mice in each group: (1) normal control (NC) group, (2) positive control (PC) group, (3) low-dose OPH (OPH-L) group, (4) middle-dose OPH (OPH-M) group, (5) high-dose OPH (OPH-H) group. The NC group was gavaged 0.4 mL normal saline. The PC group was gavaged 0.4 mL huperzine water solution (Shanghai Fudan Fuhua Pharmaceutical Co., Ltd, Shanghai, China) at a dose of 0.05 mg kg−1. The OPH groups were gavaged 0.4 mL OPH water solution with the dose of 150 mg kg−1, 500 mg kg−1 and 1500 mg kg−1, respectively. One part mice were subject to the Morris water maze test and the other part mice were subject to the dark/light avoidance test at the end of the treatment period.
2.2.8 Morris water maze test. Spatial learning and memory ability of the mice was evaluated by the Morris water maze test, which has become one of the most frequently used laboratory tools in behavioral neuroscience.29,30 The experimental apparatus, consisting of a blank circular tank (diameter 120 cm, height 50 cm), filled with water (24 ± 1 °C) to a height of 30 cm, and was located in a test room. The tank was divided into four equal quadrants, and a movable circular platform (9 cm diameter) was placed in the fourth quadrant, submerged 1 cm below the water surface, and invisible. After 30 days of oral administration, the mice were placed in water to swim freely for two minutes to become familiar with the environment on the first day. A trial began by releasing the mice into the water pool from one of the four different starting positions facing towards the wall of the maze. The mice were trained to swim to the platform in the circular tank. If a mouse was unable to find the platform within 90 seconds, it was guided to the platform and placed on the platform for 10 seconds. The four quadrants training are completed as one trial. The mice received three training trials per day for five consecutive days, and escape latency was measured as a learning score by an auto-tracking system.A spatial probe trial was carried out after five days. In this test, the platform was removed from the water tank. The mice were placed into the water pool from one of the four different starting positions facing towards the wall of the maze, and total swimming distance, swimming time and frequency of going into the quadrants of the mice in the trail were recorded. Based on the above experiment data, the percentage of swimming distance/time in the quadrant to the total distance/time would reflect the spatial memory ability of the mice on the location of the platform.
2.2.9 Dark/light avoidance test. After 45 days of oral administration, the dark/light avoidance test was carried out from day 46 to day 50, according to Yamaguchi et al.31 with some modification. The apparatus consisted of light (12 cm × 4.5 cm) and dark (17 cm × 4.5 cm) chambers; a door with a diameter of 3 cm was set for separation of the two chambers. Electric wires, arranged with a parallel interval of 1 cm, were set in the two chambers, and delivered an electric shock (0.3–0.4 mA) in the dark chamber. In each trial, a mouse was placed into the light chamber first with the shuttle door open. After the mouse entered the dark chamber, an escape failure was recorded and an inescapable electric shock was delivered through the electric wires. The mouse was removed from the dark chamber and the trial ended. The trial also ended if the mouse remained in the light chamber for 300 seconds. The avoidance test was repeated after 24 hours, and the memory reappearance experiment was carried out after five days, which could estimate the ability of memory in mice.
2.2.10 Biochemical parameters of mice. After completing the behavioral studies, the mice were anesthetized and decapitated, and the blood was collected. The brain, spleen and thymus were taken out and weighed. The hippocampus was separated from the whole brain on ice, blotted gently with filter paper to remove blood and extraneous tissue fragments, then flash frozen with liquid nitrogen and stored at −80 °C until use. The hippocampus was suspended in ice-cold, normal saline at a ratio of 1
:
9. The homogenate was centrifuged at 10
000 × g for 30 minutes, and the supernatant was used for a biochemical assay.The contents of ROS, malondialdehyde (MDA), superoxide dismutase (SOD), glutathione peroxidase (GSH-Px), tumor necrosis factor-α (TNF-α), interleukin-6 (IL-6), interleukin-1β (IL-1β), AChE and choline acetyltransferase (ChAT) were determined using assay kits (Institute of Biological Engineering of Nanjing Jiancheng, Nanjing, China). Brain-derived neurotrophic factor (BDNF) and neural cell adhesion molecules (NCAM) were determined using ELISA testing (Institute of Biological Engineering of Nanjing Jiancheng, Nanjing, China). In addition, the levels of BDNF and NCAM protein expressions were determined by western blot. This section was conducted by Xi'an United Nations Quality Detection Technology CO., Ltd (Xi'an, China).
2.3 Statistical analysis
Data were presented as means ± SD. The statistical significance of the data was determined by variance analysis (ANOVA) using the SPSS software (version 18.0 for Windows, SPSS Inc., Chicago, IL, USA) and means were compared by Duncan's multiple comparison post-test. Statistical differences were considered to be significant at p < 0.05.
3. Results and discussions
3.1 Selections of proteolytic enzymes
The types of enzymes that affect antioxidant activity and other biological activities of enzymatic hydrolysis32 is critical to select an appropriate protease because this leads to different peptide bond cleavage patterns in protein hydrolysates according to the enzyme used.33 In this study, five proteases were used for hydrolytic production of OPH: protamex, trypsin, papain, neutrase and flavourzyme. Selection of these proteases was based on the ability of each enzyme to reach high radical scavenging activities and low AChE activity. As shown in Table 1, hydrolysate treated with protamex demonstrated the highest soluble peptide content of 5.86 g/100 mL, hydroxyl radical scavenging activity of 70.78%, DPPH radical scavenging activity of 83.41% and superoxide radical scavenging activity of 62.53%, which were significantly different from other four proteases hydrolysates (P < 0.05), and protamex hydrolysate also showed considerable radical scavenging activities compared to the standard antioxidant (Vc). These results were consistent with those reported by Jia et al.,34 where Alaska pollack skin was treated with six kinds of proteases, showing protamex hydrolysate exhibiting the highest yield of peptide and antioxidant activities. Furthermore, the catalytic activity of AChE was confirmed through complete inhibition with AChE inhibitor.35 The protamex hydrolysate exhibited the lowest AChE activity of 0.080 mM min−1, meaning that OPH prepared with protamex could better inhibit AChE activity. Therefore, protamex was chosen as the best candidate for further study.
Table 1 The soluble peptide content, radical scavenging activities and AChE activity of OPH prepared by different proteasesa
Protease |
Soluble peptide content (g/100 mL) |
Hydroxyl radical scavenge activity (%) |
DPPH radical scavenging activity (%) |
Superoxide-radical scavenging (%) |
AChE activity (U mL−1) |
Data were presented as means ± SD. Values followed by different superscript in the same columns were significantly different (P < 0.05) according to variance analysis (ANOVA) using the SPSS software and means were compared by Duncan's multiple comparison post-test. Statistical differences were considered to be significant at p < 0.05. |
Protamex |
5.86 ± 0.04a |
70.78 ± 0.30a |
83.41 ± 0.92a |
62.53 ± 0.48a |
0.080 ± 0.01c |
Trypsin |
4.26 ± 0.01b |
64.93 ± 0.70b |
77.42 ± 0.46c |
46.83 ± 0.28c |
0.086 ± 0.01b |
Papain |
4.04 ± 0.12c |
60.89 ± 1.08e |
77.11 ± 0.70c |
31.04 ± 1.88e |
0.085 ± 0.01b |
Flavourzyme |
2.42 ± 0.06d |
62.87 ± 0.93 cd |
80.03 ± 0.96b |
40.13 ± 0.69d |
0.085 ± 0.01b |
Neutrase |
4.25 ± 0.09b |
64.34 ± 0.80bc |
68.97 ± 0.96e |
25.34 ± 1.65f |
0.088 ± 0.01a |
Vc |
|
62.46 ± 1.05d |
71.88 ± 0.67d |
55.09 ± 0.61b |
|
3.2 Single factor experiments
In this work, the effects of five single factors on the AChE activity were investigated. Results showed that under the range of these five single factors, the AChE activity decreased at first followed by an increase. As such, optimal conditions were determined as follows: enzyme concentration of 1200 U g−1, extraction time of 5.0 hours, pH of 8.0, a water/material ratio of 2
:
1 and extraction temperature of 55 °C.
3.3 Optimization of extraction conditions by BBD
The single factor experiments involved five independent variables at five levels employed in a Box–Behnken Design (BBD). The five independent variables, enzyme concentration, extraction time, pH, water/material ratio and extraction temperature, were coded as X1, X2, X3, X4 and X5, respectively. The ranges and levels of the variables were given by the Design Expert 8.0 (Stat-Ease. Inc., China), which was used to analyze and calculate the predicted responses and experimental design for the AChE activity. The design matrix and corresponding results obtained from BBD for determining the effects of the five independent variables, X1, X2, X3, X4 and X5, were listed in Table 2.
Table 2 Experimental design and result of response surface
Run numbers |
X1 |
X2 |
X3 |
X4 |
X5 |
AChE activity (mM min−1) |
1 |
800 |
3 |
8 |
2 |
55 |
0.074 |
2 |
1600 |
3 |
8 |
2 |
55 |
0.072 |
3 |
800 |
7 |
8 |
2 |
55 |
0.072 |
4 |
1600 |
7 |
8 |
2 |
55 |
0.070 |
5 |
1200 |
5 |
7 |
1 |
55 |
0.071 |
6 |
1200 |
5 |
9 |
1 |
55 |
0.072 |
7 |
1200 |
5 |
7 |
3 |
55 |
0.070 |
8 |
1200 |
5 |
9 |
3 |
55 |
0.070 |
9 |
1200 |
3 |
8 |
2 |
45 |
0.072 |
10 |
1200 |
7 |
8 |
2 |
45 |
0.070 |
11 |
1200 |
3 |
8 |
2 |
65 |
0.073 |
12 |
1200 |
7 |
8 |
2 |
65 |
0.071 |
13 |
800 |
5 |
7 |
2 |
55 |
0.072 |
14 |
1600 |
5 |
7 |
2 |
55 |
0.070 |
15 |
800 |
5 |
9 |
2 |
55 |
0.072 |
16 |
1600 |
5 |
9 |
2 |
55 |
0.070 |
17 |
1200 |
5 |
8 |
1 |
45 |
0.070 |
18 |
1200 |
5 |
8 |
3 |
45 |
0.069 |
19 |
1200 |
5 |
8 |
1 |
65 |
0.072 |
20 |
1200 |
5 |
8 |
3 |
65 |
0.070 |
21 |
1200 |
3 |
7 |
2 |
55 |
0.072 |
22 |
1200 |
7 |
7 |
2 |
55 |
0.070 |
23 |
1200 |
3 |
9 |
2 |
55 |
0.072 |
24 |
1200 |
7 |
9 |
2 |
55 |
0.071 |
25 |
800 |
5 |
8 |
1 |
55 |
0.072 |
26 |
1600 |
5 |
8 |
1 |
55 |
0.071 |
27 |
800 |
5 |
8 |
3 |
55 |
0.071 |
28 |
1600 |
5 |
8 |
3 |
55 |
0.070 |
29 |
1200 |
5 |
7 |
2 |
45 |
0.070 |
30 |
1200 |
5 |
9 |
2 |
45 |
0.070 |
31 |
1200 |
5 |
7 |
2 |
65 |
0.070 |
32 |
1200 |
5 |
9 |
2 |
65 |
0.070 |
33 |
800 |
5 |
8 |
2 |
45 |
0.071 |
34 |
1600 |
5 |
8 |
2 |
45 |
0.069 |
35 |
800 |
5 |
8 |
2 |
65 |
0.072 |
36 |
1600 |
5 |
8 |
2 |
65 |
0.071 |
37 |
1200 |
3 |
8 |
1 |
55 |
0.072 |
38 |
1200 |
7 |
8 |
1 |
55 |
0.071 |
39 |
1200 |
3 |
8 |
3 |
55 |
0.072 |
40 |
1200 |
7 |
8 |
3 |
55 |
0.070 |
41 |
1200 |
5 |
8 |
2 |
55 |
0.070 |
42 |
1200 |
5 |
8 |
2 |
55 |
0.070 |
43 |
1200 |
5 |
8 |
2 |
55 |
0.069 |
44 |
1200 |
5 |
8 |
2 |
55 |
0.069 |
45 |
1200 |
5 |
8 |
2 |
55 |
0.070 |
46 |
1200 |
5 |
8 |
2 |
55 |
0.070 |
These results showed that the AChE activity ranged from 0.069 to 0.074 mM min−1. The analysis of variance (ANOVA) results for the model were given in Table 3. The corresponding variables were more significant as the F-value became greater and the P-value became smaller.36 The model F-value of 11.99 implies the model was significant. The value of “Prob > F” of less than 0.0500 indicated that the model terms were significant. Additionally, it could be stated that the variables with significant effects on the AChE activity of OPH were linear terms (X1, X2, X4 and X5), quadratic terms (X12, X22, X32 and X42). Furthermore, the model showed good fit with the experimental data, with high values of R2 (90.56%) and adj. R2 (83.00%). The low coefficient value of the variation (CV = 0.66%) clearly suggested a high degree of precision and reliability of the experimental values. This result implied that the hydrolysis process of OPH could be analyzed and predicted by the model.
Table 3 ANOVA for response surface quadratic model
Variables |
Sum of squares |
df |
Mean square |
F value |
P value prob > F |
Model |
5.19 × 10−5 |
20 |
2.59 × 10−6 |
11.99 |
<0.0001 |
Residual |
5.41 × 10−6 |
25 |
2.16 × 10−7 |
|
|
Lack of fit |
4.53 × 10−6 |
20 |
2.27 × 10−7 |
1.30 |
0.4180 |
Pure error |
8.73 × 10−7 |
5 |
1.75 × 10−7 |
|
|
Cor. total |
5.73 × 10−5 |
45 |
|
|
|
R2 |
0.9056 |
|
|
|
|
Adj. R2 |
0.8300 |
|
|
|
|
Pred. R2 |
0.6613 |
|
|
|
|
Adeq. precision |
13.421 |
|
|
|
|
CV% |
0.66 |
|
|
|
|
Using Design-Expert 8.0, the optimal hydrolysate conditions were as follows: enzyme concentration of 1444.88 U g−1, extraction time of 5.56 hours, pH of 7.38, and a water/material ratio of 2.45
:
1 and extraction temperature of 45 °C. The minimum AChE activity was 0.069 mM min−1, which was in agreement with the experimental value (0.071 mM min−1), suggesting a good fit between the model and experimental data.
3.4 Effect of OPH on the body weight and viscera indexes of mice
After 45 days of oral administration, the mice showed no mortality or behavioral abnormalities. In addition, body weight and viscera indexes of the mice were shown in Table 4. No differences in body weight were shown between the five treatment groups at the end of 45 days of normal saline, huperzine, OPH-L, OPH-M or OPH-H oral administration. In addition, there were no significant changes in brain and spleen indexes among the five groups of mice (P < 0.05). Only the thymus index was significant higher in the OPH-L group compared to the NC group. The result showed that the PC and OPH groups had no significant effect on the body weight and viscera indexes of mice.
Table 4 Effect of OPH on the body weight and viscera indexes of micea
Groups |
Body weight (g) |
Viscera indexesb (mg g−1) |
Beginning |
Ending |
Brain |
Spleen |
Thymus |
Data were presented as means ± SD. Mean values within the same column with different superscript were significantly different according to variance analysis (ANOVA) using the SPSS software and means were compared by Duncan's multiple comparison post-test. Statistical differences were considered to be significant at p < 0.05. Viscera indexes = weight of brain, spleen or thymus (mg)/weight of mice (g) × 10. |
NC |
21.45 ± 1.97 |
32.53 ± 2.34 |
12.85 ± 1.29 |
2.26 ± 0.40 |
2.56 ± 0.51b |
PC |
19.83 ± 2.02 |
32.46 ± 2.40 |
13.28 ± 1.23 |
2.23 ± 0.48 |
2.98 ± 0.69ab |
MPH-L |
20.33 ± 1.56 |
32.89 ± 2.65 |
12.65 ± 1.27 |
2.51 ± 0.51 |
2.71 ± 0.49b |
MPH-M |
20.33 ± 1.83 |
31.33 ± 3.01 |
13.62 ± 1.82 |
2.36 ± 0.61 |
2.65 ± 0.68b |
MPH-H |
20.04 ± 1.96 |
33.76 ± 2.98 |
12.72 ± 0.91 |
2.33 ± 0.56 |
3.44 ± 1.01a |
3.5 Latency of mice in Morris water maze
We conducted a Morris water maze test to assess memory and learning ability of the mice treated with OPH. Reference memory outcomes from Morris water maze test were shown in Fig. 1. After five days of training, escape latency in the five groups reduced, which proving the mice exhibited spatial learning and memory. On the first day, the escape latency of the NC group and PC group were about 44.40 seconds and 41.03 seconds, and the mice in the OPH groups showed better performance with the escape latency were 39.75 seconds, 41.10 seconds and 33.39 seconds respectively. During the five days of training, OPH administrations also resulted in shorter escape latency compared with the NC and PC groups. In addition, changes in path length produced by training trials in each group showed a pattern similar to that of escape latency.
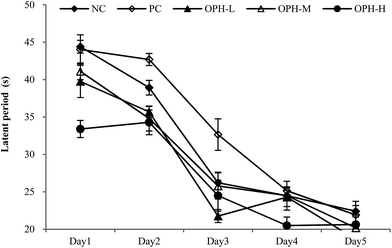 |
| Fig. 1 Effect of OPH on performance in the training trials of reference memory task. After daily oral administration with normal saline (NC group), huperzine (PC group) or OPH (OPH-L, OPH-M and OPH-H groups) for 30 days, animals were test in Morris water maze task. Values were presented as means ± SD. | |
Following the reference memory task, a spatial probe trial was carried out after five days. As shown in Fig. 2A, the OPH groups significantly shorten the total swimming distance, compared to the NC group (P < 0.05). Besides, OPH-M group showed the shortest total swimming distance by 11
416.52 ± 2491 mm, which was also significantly shorter than that in the PC group (P < 0.05). Fig. 2B indicated that there was a significant group effect on the time spent in the quadrant, in which the platform was located at the same place during training (target quadrant). The NC group spent less time in the target quadrant than the OPH-M group (p < 0.05), moreover, compared to the PC group, OPH groups could also prolong the time that the mice spent in the target quadrant (p < 0.05), which showed the mice were consciously looking for the platform. We suggested that the performance of mice in OPH group was attributable to the effect of OPH. Additionally, swimming pathway is helpful in understanding the truth of the memory and learning ability of mice in spatial probe trial. Fig. 2C clearly indicated the swimming pathway in the five groups, the mice of NC group searched the target quadrant with directionless and disorder path; while the OPH and PC groups showed the simple and clear path, which indicated the memory and learning ability of mice was improved.
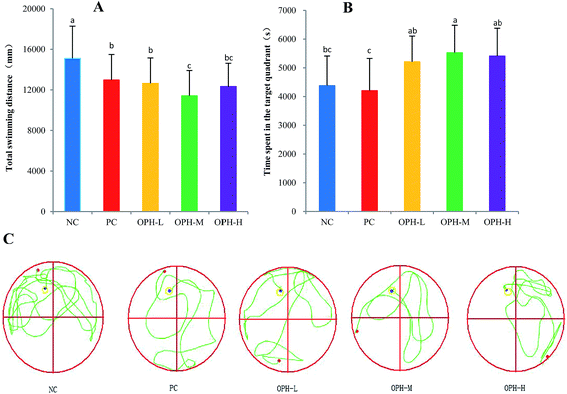 |
| Fig. 2 Effect of OPH on performance in the probe trial of the water maze task. (A) Comparison of total swimming distance of each group. (B) The time of each group in the target quadrant in the probe trial. (C) The swimming pathway of each group in the probe trial. Data were presented as means ± SD. Bar graphs followed by different letters were significantly different according to variance analysis (ANOVA) using the SPSS software and means were compared by Duncan's multiple comparison post-test. Statistical differences were considered to be significant at p < 0.05. | |
3.6 Dark/light avoidance test
A dark/light avoidance test was used to evaluate the memory and learning ability in an animal model.11 Step-through latency from a light chamber to a dark chamber was used as a marker to evaluate memory and learning ability.29 As shown in Fig. 3, all the mice went into the dark chamber in a relatively short time because of skototaxis, with no significant difference in the five groups in the first trials. The aim of this test was to prove the mice went into the dark chamber and received an electric shock, whether they could remember the electric shock and avoided entering the dark chamber the next time.
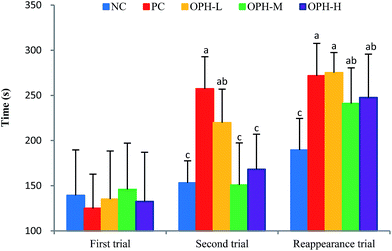 |
| Fig. 3 Effect of OPH on step-through latency of dark/light avoidance test. The latency of five groups in the first trial showed no significant difference, and bar graphs followed no letters. In the second trial and reappearance trial, the same letters between the two groups meant no significant difference. Data were presented as means ± SD. Bar graphs followed by different letters were significantly different (P < 0.05) according to variance analysis (ANOVA) using the SPSS software and means were compared by Duncan's multiple comparison post-test. Statistical differences were considered to be significant at p < 0.05. | |
Interestingly, when we placed the mice in the light chamber after 24 hours, the step-through latency of the five groups showed different behaviors in the second trial. The PC group showed the longest step-through latency by 257.3 seconds, which was significantly different from the NC, OPH-M and OPH-H groups (P < 0.05). The result indicated that the mice in the PC group remembered the electric shock and stayed in the light chamber for the longest time. In addition, the OPH-L group stayed in the light chamber for a longer time compared to the NC group. In general dose–response model, an effect increases with dose increases.37 However, an interesting phenomenon to note was that the OPH groups exhibited no dose-dependence in the dark/light avoidance test.
The memory reappearance trial was carried out five days later, with the purpose of estimating the mice ability of memory. As shown in Fig. 3, the mice in NC group still spent the shortest time staying in the light chamber and the treatment with OPH significantly prolonged the latency of entering the dark chamber comparing with the NC group (P < 0.05). Interestingly, there was no significant difference between the OPH treated groups, which was similar to the result from Chai et al.9 In addition, the latency to enter the dark chamber was also longer in the PC group than those of the NC group.
3.7 Effect of OPH relieved the oxidative stress in vivo
In recent years, some studies revealed that antioxidative peptides could improve learning and memory ability via antioxidative stress.11 It is possible that antioxidative peptides with strong free radical-scavenging activity might exhibit some beneficial effect on impairment-related memory degeneration.11,38 It is certain now that oxidative stress can lead to cell and tissue damage, thus resulting in age-related cognitive decline.39 As such, it is reasonable to study the relationship between OPH and oxidative stress.
Oxidative stress refers to an imbalance between cellular productions of ROS and antioxidant enzymes, such as SOD and GSH-Px.40 ROS is a collective term for oxygen-centered radicals. In humans, uncontrolled generation of ROS can result in oxidative damage of cellular DNA, protein and lipids; even the development of Alzheimer's and inflammatory diseases.41,42 As shown in Fig. 4, the increase of ROS in the NC group reflected free radical accumulation after the dark/light avoidance test, this was consistent with those reported by Vollaard et al.,43 who considered that exercise was accompanied by an increased generation of free radicals. While the ROS levels in OPH groups were significantly lower than that of the NC group (P < 0.05), and the results indicate that the OPH groups could eliminate free radicals and reduce oxidative stress. Moreover, the ROS level of the PC group was also significantly lower than that of the NC group.
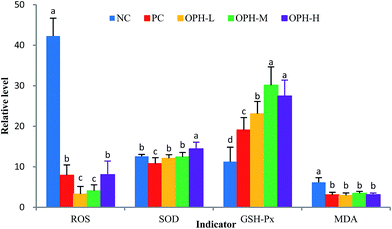 |
| Fig. 4 Effect of OPH on indicators related to oxidative stress of mice. The vertical units of groups were %, U mg−1, U mg−1 and mmol mg−1, respectively. Data were presented as means ± SD. Bar graphs followed by different letters were significantly different (P < 0.05) according to variance analysis (ANOVA) using the SPSS software and means were compared by Duncan's multiple comparison post-test. Statistical differences were considered to be significant at p < 0.05. | |
The whole brain is vulnerable to free radical-induced damage because of its high oxygen consumption, abundant lipid content and relative paucity of antioxidant enzymes.44 SOD and GSH-Px were actual indicators of the antioxidant capacity of the body and were involved in protection against damage caused by oxidative stress. Fig. 4 showed that the level of SOD was significantly increased in the OPH-H group compared with the NC group (P < 0.05), while there was no significant difference between other OPH groups and the NC group. On the other hand, the level of GSH-Px was significantly increased in the OPH groups compared to the NC group (P < 0.05), which indicated that all the OPH groups could increase enzymatic antioxidant activity and reduce oxidative stress in the body. Additionally, MDA was one of the end products in the lipid metabolism, and increasing MDA levels reflected the damage to tissue and cell caused by accumulation of free radicals.45 As shown in Fig. 4, the MDA levels of all OPH groups were significantly lower than that of the NC group; moreover, the MDA level of the PC group was also significantly lower than that of the NC group (P < 0.05). Therefore, the OPH groups could significantly relieve oxidative stress in vivo.
In addition, there are some studies illustrating that learning and memory ability is associated with oxidative stress. For example, Gao et al.46 found that fucoidan from brown algae could ameliorate Aβ-induced memory impairments in rats by increasing the homogenate levels of SOD and GSH-Px, and decreasing the level of MDA. Zhou et al.47 exhibited that mussel oligopeptides could improve cognitive learning, memory ability and protect the hippocampal neurons. In addition, GSH, SOD and GSH-Px activities were increased and MDA level was significantly decreased in mice fed with mussel oligopeptides. It was found that antioxidant therapy prevented learning and memory deficits induced by Aβ in rats. Therefore, OPH had in vivo antioxidant activity, indicating that it might have the potential to improve learning and memory ability.
3.8 Effect of OPH on indicators related to improving memory and inflammatory reaction
3.8.1 AChE and ChAT. Biological studies have found that the central cholinergic system is closely linked to learning and memory, and that normal functioning of central cholinergic nerves is essential for learning and memory.48 AChE present in cholinergic axons and in some cholinoceptive pyramidal cortical neurons. A previous study reported that AChE activity is considered to be a potential therapeutic approach that can slow or mitigate the progression of AD.49 Furthermore, ChAT is the major player of the cholinergic system, and is involved in acetylcholine synthesis and breakdown.50 A decrease in ChAT activity, as well as inflammatory response, was found in the hippocampus and cerebral cortex of AD patients.29 Additionally, AChE and ChAT may play a substantial part in the improvement of learning and memory ability. However, synthetic drugs have long-term safety problems and a negative consumer perception.8 For these reasons, the demand for natural AChE inhibitors has recently increased. For example, Hirbod et al.51 have found the leaf and stem extractions of the Aquilaria were safe for use as natural AChE inhibitors, an alternative to berberine for the treatment of AD. In addition, Zhao et al.52 studied rhizome extractions from Anemarrhena asphodeloides Bge., which is widely used in traditional Chinese medicine, and the results indicated that rhizome extraction offered protection against scopolamine-induced deficits in learning and memory, possibly by inhibiting AChE and preventing oxidative stress damage.In this section, we have measured the AChE activity and ChAT activity in the brain. Table 5 showed that the OPH-H group significantly inhibited AChE activity by 13.88 mM min−1 compared to that of the NC group of 28.89 mM min−1 (P < 0.05). Interestingly, the OPH-L, OPH-M and PC groups did not decrease the AChE activity compared to that of the NC group.
Table 5 Effect of OPH on AChE, ChAT, IL-1β, IL-6 and TNF-α of micea
Group |
AChE (mM min−1) |
ChAT (mM min−1) |
IL-1β (pg mL−1) |
IL-6 (pg mL−1) |
TNF-α (pg mL−1) |
Data were presented as means ± SD. Values followed by different superscript in the same columns were significantly different according to variance analysis (ANOVA) using the SPSS software and means were compared by Duncan's multiple comparison post-test. Statistical differences were considered to be significant at p < 0.05. |
NC |
28.89 ± 3.88a |
37.97 ± 2.14d |
66.19 ± 11.17c |
81.33 ± 4.02b |
388.72 ± 29.32b |
PC |
28.51 ± 3.71a |
44.94 ± 4.13c |
84.64 ± 2.89b |
127.74 ± 6.21a |
475.90 ± 20.76a |
OPH-L |
30.13 ± 4.04a |
53.77 ± 2.89b |
97.31 ± 6.81a |
89.15 ± 4.15b |
399.54 ± 32.26b |
OPH-M |
26.19 ± 3.07a |
65.54 ± 2.16a |
86.97 ± 7.30b |
77.39 ± 5.46c |
359.29 ± 73.56c |
OPH-H |
13.88 ± 3.82b |
38.68 ± 2.18d |
52.31 ± 7.44d |
77.01 ± 5.94c |
338.05 ± 13.71d |
Soodi et al.53 demonstrated that learning and memory deficit was associated with reduced ChAT in the hippocampus. As shown in Table 5, the expression of ChAT by 53.77 and 65.54 mM min−1 in the OPH-L and OPH-M were significantly increased in contrast to the NC group (P < 0.05). In addition, the PC group also increased the expression of ChAT compared to that of the NC group (P < 0.05). However, the OPH group showed no dose-dependence, and there was no statistical significance between the OPH-H and NC groups. As such, we assumed that there were great individual differences in animal experiments.
3.8.2 Inflammatory factors: TNF-α, IL-6 and IL-1β. As one of the most generic response in innate immunity system, inflammation is believed to play crucial roles in the pathogenesis of various diseases.54,55 During the dark/light avoidance test, the mouse carried out a lot of exercise and was frightened. There were some studies have shown that strenuous exercise or intense muscular work could induce oxidative stress and inflammation.43,56 Furthermore, when the body had an inflammatory response, the immune defense system could respond actively to inflammation by releasing pro-inflammatory cytokines, such as TNF-α, IL-6 and IL-1β. However, high levels of these cytokines could cause systemic complications.57Based on the above theories, we have investigated the effects of OPH on pro-inflammatory cytokines production, including TNF-α, IL-6 and IL-1β. The TNF-α was elevated in several neuropathological states that are associated with learning and memory deficits58 and could stimulate the production or expression of IL-6 and IL-1β.59 As shown in Table 5, after treatment with OPH, the TNF-α level decreased significantly in OPH-M and OPH-H groups compared to that of the NC group (P < 0.05). The result was consistent with those reported by Lee et al.,60 who found oyster shell extract significantly inhibited the production of IL-1β, IL-6, and TNF-α in a dose-dependent manner. Recent studies suggest IL-6 may sit in a pivotal position and serve as an important catalyst because administration of IL-6 improved spatial alternation behavior61 and positively correlated with deficits in cognition in humans.62 Therefore, we had also studied the level of IL-6 after treatment with OPH and the result showed that the IL-6 level decreased significantly in OPH-M and OPH-H groups compared to that of the NC group (P < 0.05). Furthermore, it has been widely described that immune activation, such as IL-1β caused deficits in learning and memory.63 As shown in Table 5, IL-1β level decreased dramatically with OPH-H treatment compared to that of the NC group (P < 0.05). This result indicated that OPH could attenuate the production of pro-inflammatory cytokines, including TNF-α, IL-1β, and IL-6. Whereas IL-1β, IL-6 and TNF-α levels in the PC group was increased dramatically compared to that of the NC group. Several types of drugs are used to treat inflammatory disorders, but they cause adverse side effects. We inferred that the PC group would demonstrate some inflammatory activity when compared to the normal mice, and that natural products are alternatives to these drugs for treatment.
3.8.3 BDNF and NCAM. BDNF is a critical molecule for learning and memory.64,65 In AD, there is a significant down-regulation of BDNF messenger RNA and protein, which is strongly implicated in loss of memory and cognitive function.66 As shown in Fig. 5A, quantitative analysis showed that hippocampal level of BDNF increased to 2302 pg mL−1 in the OPH group was obviously higher than that by 2167 pg mL−1 in the NC group (P < 0.05). However, the PC group was down-regulated of BDNF levels by 1929 pg mL−1.
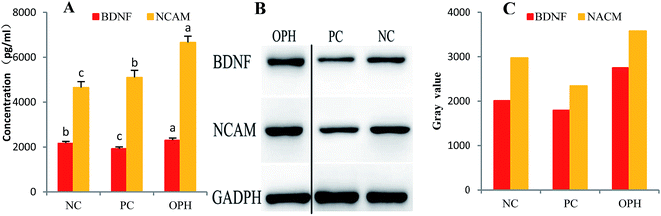 |
| Fig. 5 Immunoblot analysis of BDNF and NCAM in the hippocampus of the OPH, PC and NC treated mice. (A) Quantitative analysis showed that the hippocampal levels of BDNF and NCAM of the OPH group were obviously higher than that of the NC group. (B) Western blot from the NC and PC groups showed weak hippocampal BDNF and NCAM compared with the OPH group. The OPH group data and NC and PC group's data were spliced together because there were other data between them in the initial gel. (C) Gray value of BDNF and NCAM in the hippocampus of mice treated with the OPH, PC and NC. Data were presented as means ± SD. Bar graphs followed by different letters were significantly different (P < 0.05) according to variance analysis (ANOVA) using the SPSS software and means were compared by Duncan's multiple comparison post-test. Statistical differences were considered to be significant at p < 0.05. | |
NCAM are complexes of transmembrane proteins critical for cell–cell interactions. Crucially, subsequent research demonstrated that NCAMs retain these functions in the mature adult brain with regards to cognitive and mimetics abilities.67 In this section, we have also investigated the NCAM levels and found that these levels in OPH group were significantly up-regulated compared to that of the NC group (Fig. 5A).
Furthermore, as shown in Fig. 5B, western blot from the PC group showed weak hippocampal BDNF and NCAM expressions compared with the NC group. These results reinforced that BDNF and NCAM expressions were observed in the OPH-treated groups. Additionally, the gray values of BDNF and NCAM in the hippocampus of the OPH group were higher than that of NC group in Fig. 5C. The result also showed that the OPH could improve learning and memory ability by up-regulating the BDNF and NCAM levels.
4. Conclusions
In this work, we confirmed the preparation conditions of OPH by RSM and proved that OPH had a spatial learning and memory capacity. In the Morris water maze test, the mice in OPH group showed shorter escape latency and total swimming distance; in the dark/light avoidance test, the mice in OPH group also showed longer step-through latency. Refer to other research works, we inferred that learning and memory ability was associated with oxidative stress and inflammatory reaction, and the related indexes confirmed our conjecture. However, the individual differences between mice were too great, and the OPH group did not show dose-dependent behavior in some experimental results, and, if possible, we would adjust the OPH dose in a future animal experiment. Furthermore, we also measured related indexes such as AChE and ChAT to proof the OPH could be a candidate functional food for the improvement of learning and memory. On the basis of the results obtained in this work, more work is worthy to be done to reveal the mechanisms on learning and memory ability of OPH, and further purification and identification of OPH was needed.
Conflicts of interest
The authors declare that there is no conflict of interests regarding the publication of this paper.
Acknowledgements
The study was supported by the Key Research and Development Program of Shandong Province (2017YYSP018), National Natural Science Foundation of China (No. 41506175), Qingdao Science and Technology Project (No. 17-3-3-21-nsh), Science and Technology Development Plan Project of Shandong Province (2016YYSP010) and the Key Research Program of the Chinese Academy of Sciences (No. KFZD-SW-106).
References
- L. Ma, L. Chang, X. Y. Chen and R. L. Zhou, PLoS One, 2017, 12, 1–19 Search PubMed.
- D. A. B. D. Boyd-Kimball, Brain Pathol., 2004, 14, 426–432 Search PubMed.
- C. Supnet and I. Bezprozvanny, Cell Calcium, 2011, 50, 303–309 CrossRef CAS PubMed.
- M. S. Rafii and P. S. Aisen, BMC Med., 2015, 13, 1–7 CrossRef CAS PubMed.
- R. Brookmeyer, E. Johnson, K. Ziegler-Graham and H. M. Arrighi, Alzheimer's Dementia, 2007, 3, 186–191 CrossRef PubMed.
- M. P. Mattson, Nature, 2004, 430, 631–639 CrossRef CAS PubMed.
- H. R. Amanatkar, B. Papagiannopoulos and G. T. Grossberg, Expert Rev. Neurother., 2017, 17, 7–16 CrossRef CAS PubMed.
- E. D. Roberson and L. Mucke, Science, 2006, 314, 781–784 CrossRef PubMed.
- H.-J. Chai, C.-J. Wu, S.-H. Yang, T.-L. Li and B. Sun Pan, J. Funct. Foods, 2016, 24, 438–449 CrossRef CAS.
- J. A. Pillai, A. Bonner-Jackson, E. Walker, L. Mourany and J. L. Cummings, Dementia Geriatr. Cognit. Disord., 2014, 38, 224–233 CrossRef PubMed.
- H. Chen, M. Zhao, L. Lin, J. Wang, D. Sun-Waterhouse, Y. Dong, M. Zhuang and G. Su, Food Res. Int., 2015, 78, 216–223 CrossRef CAS PubMed.
- H. J. Heo, S. J. Choi, S. G. Choi, D. H. Shin, J. M. Lee and C. Y. Lee, J. Food Sci., 2008, 73, H28–H32 CrossRef CAS PubMed.
- Z. Li, X. Chen, W. Lu, S. Zhang, X. Guan, Z. Li and D. Wang, Int. J. Mol. Sci., 2017, 18, 1–14 Search PubMed.
- C. S. Raine, Neurobiol. Aging, 2000, 21, 437–440 CrossRef CAS PubMed.
- S. Xiao, D. Zhou, P. Luan, B. Gu, L. Feng, S. Fan, W. Liao, W. Fang, L. Yang, E. Tao, R. Guo and J. Liu, Biomaterials, 2016, 106, 98–110 CrossRef CAS PubMed.
- S. Duan, X. Guan, R. Lin, X. Liu, Y. Yan, R. Lin, T. Zhang, X. Chen, J. Huang, X. Sun, Q. Li, S. Fang, J. Xu, Z. Yao and H. Gu, Neurobiol. Aging, 2015, 36, 1792–1807 CrossRef CAS PubMed.
- B. Bloem, R. B. Poorthuis and H. D. Mansvelder, Front. Neural Circuits, 2014, 8, 1–16 Search PubMed.
- M. H. Kim, S.-H. Kim and W. M. Yang, Planta Med., 2014, 80, 1249–1258 CrossRef CAS PubMed.
- Q. Wang, W. Li, Y. He, D. Ren, F. Kow, L. Song and X. Yu, Food Chem., 2014, 145, 991–996 CrossRef CAS PubMed.
- J. Wang, J. Hu, J. Cui, X. Bai, Y. Du, Y. Miyaguchi and B. Lin, Food Chem., 2008, 111, 302–308 CrossRef CAS PubMed.
- X. C. He, Y. Zhang, F. Yu and Z. N. Yu, Fish Shellfish Immunol., 2011, 31, 1247–1250 CrossRef CAS PubMed.
- Y. K. Wang, H. L. He, G. F. Wang, H. Wu, B. C. Zhou, X. L. Chen and Y. Z. Zhang, Mar. Drugs, 2010, 8, 255–268 CrossRef CAS PubMed.
- T. Xu, J. Xie, B. Zhu, X. Liu and X. Wu, PLoS One, 2014, 9, e95859 Search PubMed.
- H. S. Lin, W. H. Cao, H. Y. Lu, C. H. Zhang, X. M. Qin and M. Xie, Food Sci. Technol., 2013, 38, 37–41 CAS.
- X. Q. Wang, R. E. Xing, S. Liu, H. H. Yu, K. C. Li, Z. Y. Chen and P. C. Li, Chin. J. Oceanol. Limnol., 2014, 33, 159–168 CrossRef.
- A. Smeriglio, M. Denaro, D. Barreca, A. Calderaro, C. Bisignano, G. Ginestra, E. Bellocco and D. Trombetta, Int. J. Mol. Sci., 2017, 18, 1–13 CrossRef PubMed.
- M. S. Blois, Nature, 1958, 181, 1199–1200 CrossRef CAS.
- M. Nishikimi, N. Appaji Rao and K. Yagi, Biochem. Biophys. Res. Commun., 1972, 46, 849–854 CrossRef CAS PubMed.
- C. L. Lee, T. F. Kuo, J. J. Wang and T. M. Pan, J. Neurosci. Res., 2007, 85, 3171–3182 CrossRef CAS PubMed.
- Y. Liu, Y. F. Xu, L. Zhang, L. Huang, P. Yu, H. Zhu, W. Deng and C. Qin, Int. J. Mol. Sci., 2017, 18, 1–17 Search PubMed.
- Y. Yamaguchi, H. Miyashita, H. Tsunekawa, A. Mouri, H.-C. Kim, K. Saito, T. Matsuno, S. Kawashima and T. Nabeshima, J. Pharmacol. Exp. Ther., 2006, 317, 1079–1087 CrossRef CAS PubMed.
- X. Fang, N. Xie, X. Chen, H. Yu and J. Chen, Food Bioprod. Process., 2012, 90, 676–682 CrossRef CAS.
- J.-Y. Ko, J.-H. Lee, K. Samarakoon, J.-S. Kim and Y.-J. Jeon, Food Chem. Toxicol., 2013, 52, 113–120 CrossRef CAS PubMed.
- J. P. Jia, Y. G. Zhou, J. Z. Lu, A. Y. Chen, Y. Z. Li and G. L. Zheng, J. Sci. Food Agric., 2010, 90, 635–640 CAS.
- G. Zha, V. P. Chen, W. K. Luk, X. Zou, R. C. Choi and K. W. Tsim, Chem.-Biol. Interact., 2013, 203, 277–281 CrossRef CAS PubMed.
- Z. Y. Zhao, Q. Zhang, Y. F. Li, L. L. Dong and S. L. Liu, Carbohydr. Polym., 2015, 119, 101–109 CrossRef CAS PubMed.
- X. Q. Wang, R. Xing, Z. Y. Chen, H. H. Yu, R. F. Li and P. C. Li, Food Funct., 2014, 5, 2113–2119 CAS.
- I. O. Ishola, F. M. Adamson and O. O. Adeyemi, Metab. Brain Dis., 2017, 32, 235–245 CrossRef CAS PubMed.
- L. Liu, J. Cao, J. Chen, X. Zhang, Z. Wu and H. Xiang, Neurosci. Lett., 2016, 631, 30–35 CrossRef CAS PubMed.
- Z. Mao, Y. L. Zheng and Y. Q. Zhang, Int. J. Mol. Sci., 2011, 12, 114–127 CrossRef CAS PubMed.
- H. E. Seifried, D. E. Anderson, E. I. Fisher and J. A. Milner, J. Nutr. Biochem., 2007, 18, 567–579 CrossRef CAS PubMed.
- G.-W. Oh, S.-C. Ko, S.-Y. Heo, N. Van-Tinh, G. Kim, C. H. Jang, W. S. Park, I.-W. Choi, Z.-J. Qian and W.-K. Jung, Process Biochem., 2015, 50, 1318–1326 CrossRef CAS.
- N. B. J. Vollaard, J. P. Shearman and C. E. Cooper, Sports Med., 2005, 35, 1045–1062 CrossRef PubMed.
- C. Noschang, R. Krolow, D. M. Arcego, A. P. Toniazzo, A. P. Huffell and C. Dalmaz, Int. J. Dev. Neurosci., 2012, 30, 285–291 CrossRef CAS PubMed.
- M. E. Inal, G. Kanbak and E. Sunal, Clin. Chim. Acta, 2001, 305, 75–80 CrossRef CAS.
- Y. Gao, C. Li, J. Yin, J. Shen, H. Wang, Y. Wu and H. Jin, Environ. Toxicol. Pharmacol., 2012, 33, 304–311 CrossRef CAS PubMed.
- Y. Zhou, Y. Dong, Q. Xu, Y. He, S. Tian, S. Zhu, Y. Zhu and X. Dong, Food Chem. Toxicol., 2013, 59, 412–420 CrossRef CAS PubMed.
- Y.-s. Li, Y.-f. Hong, J. He, J.-x. Lin, Y.-l. Shan, D.-y. Fu, Z.-p. Chen, X.-r. Ren, Z.-h. Song and L. Tao, Biol. Pharm. Bull., 2013, 36, 764–771 Search PubMed.
- Y. Zhen and F. Jian, Curr. Alzheimer Res., 2004, 1, 241–248 CrossRef.
- X. Hu, J. Wang, Q. Zhang, X. Duan, Z. Chen and Y. Zhang, J. Surg. Res., 2016, 206, 307–315 CrossRef CAS PubMed.
- B. Hirbod, M. Jamaludin, P. Mohammadjavad and A. R. Hussin, Curr. Alzheimer Res., 2014, 11, 206–214 CrossRef.
- X. Zhao, C. Liu, Y. Qi, L. Fang, J. Luo, K. Bi and Y. Jia, Metab. Brain Dis., 2016, 31, 1455–1461 CrossRef CAS PubMed.
- M. Soodi, S. Saeidnia, M. Sharifzadeh, H. Hajimehdipoor, A. Dashti, M. R. Sepand and S. Moradi, Metab. Brain Dis., 2016, 31, 395–404 CrossRef CAS PubMed.
- L. Ferrero-Miliani, O. H. Nielsen, P. S. Andersen and S. E. Girardin, Clin. Exp. Immunol., 2007, 147, 227–235 CAS.
- R. C. Cheung, T. B. Ng, J. H. Wong, Y. Chen and W. Y. Chan, Appl. Microbiol. Biotechnol., 2016, 100, 1645–1666 CrossRef CAS PubMed.
- B. K. Pedersen, K. Ostrowski, T. Rohde and H. Bruunsgaard, Can. J. Physiol. Pharmacol., 1998, 76, 505–511 CrossRef CAS PubMed.
- L. Bosca, M. Zeini, P. G. Traves and S. Hortelano, Toxicology, 2005, 208, 249–258 CrossRef CAS PubMed.
- M. P. Butler, J. J. O'Connor and P. N. Moynagh, Neuroscience, 2004, 124, 319–326 CrossRef CAS PubMed.
- B. B. Aggarwal and K. Natarajan, Eur. Cytokine Network, 1996, 7, 93–124 CAS.
- S.-Y. Lee, H.-J. Kim and J.-S. Han, Prev. Nutr. Food Sci., 2013, 18, 23–29 CrossRef PubMed.
- N. L. Sparkman, J. B. Buchanan, J. R. R. Heyen, J. Chen, J. L. Beverly and R. W. Johnson, J. Neurosci., 2006, 26, 10709–10716 CrossRef CAS PubMed.
- J. D. Weaver, M. H. Huang, M. Albert, T. Harris, J. W. Rowe and T. E. Seeman, Neurology, 2002, 59, 371–378 CrossRef CAS PubMed.
- C. Cunningham and D. J. Sanderson, Brain, Behav., Immun., 2008, 22, 1117–1127 CrossRef CAS PubMed.
- M. Fahnestock, Future Neurol., 2011, 6, 627–639 CrossRef CAS.
- H. Hori, R. Yoshimura, A. Katsuki, K. Atake, R. Igata, Y. Konishi, H. Beppu and H. Tominaga, Int. J. Mol. Sci., 2017, 18, 1–8 Search PubMed.
- M. Baydyuk and B. J. Xu, Front. Neural Circuits, 2014, 8, 1–10 Search PubMed.
- G. Dallérac, C. Rampon and V. Doyère, Neurochem. Res., 2013, 38, 1163–1173 CrossRef PubMed.
|
This journal is © The Royal Society of Chemistry 2018 |