DOI:
10.1039/C7RA12813D
(Paper)
RSC Adv., 2018,
8, 3348-3356
Co-production of microbial oil and exopolysaccharide by the oleaginous yeast Sporidiobolus pararoseus grown in fed-batch culture†
Received
27th November 2017
, Accepted 3rd January 2018
First published on 16th January 2018
1 Introduction
Nowadays, the environmental pollution and the rapid rise in the price of fossil fuels have drawn increasing interest among researchers in the study of transportation biofuels. To deal with these serious problems, alternative renewable biofuels are receiving considerable attention. One of the most prominent renewable energy resources is biodiesel that is produced from renewable biomass via transesterification of triacylglycerols to fatty acid methyl esters (FAMEs) and fatty acid ethyl esters (FAEEs). Microbial oils, as one of the types of biodiesel, can be obtained from renewable raw materials by oleaginous microorganism fermentation; also, they can be produced at a low cost with major advantages since they do not require large cultivation areas or long growing periods.1,2 Therefore, microbial oil has been considered as an important feedstock for biodiesel industry.
The economic feasibility of microorganism cultures for biodiesel production greatly depends on the high biomass productivity and appreciable oil yields. For yeast oils to be cost competitive as an oil fuel source, a potential organism should be capable of producing high oil yields (50–60% of biomass weight in the form of oils). High production cost is another limiting factor for a broader use of microbial oils. Microbial oils are more expensive than vegetable oils at present and thus, many methods are potentially valuable to improve the techno-economics of oil production processes. In previous studies, considerable efforts have been made to reduce the production costs of the oil. The cost-effective feedstock such as beet molasses, corn steep liquor and waste glycerol were used as sources of nutrients to save the production costs.3,4 Nevertheless, the production costs of the oil reduced by only 20–50% compared with that of the glucose, which is used as the carbon source. In this study, we changed these traditional principles to reduce the production costs of the oil by improving the production of the valuable co-products to share the production costs.
In our previous study, we indicated that a red yeast Sporidiobolus pararoseus JD-2 (preservation number: CCTCC M2010326), isolated from chili sauce, had a powerful oil production capacity and also accumulated a certain amount of exopolysaccharides (EPSs) and carotenoids (e.g., β-carotene, γ-carotene, torulene, torularhodin).5,6 Microbial EPSs have been widely used in numerous industries, such as textiles, adhesives, detergents and oil recovery because of their physical and chemical properties.7,8 EPSs produced by yeast comprises glucan, phosphomannan, mannan, glucomannan and galactomannan;7,9 and their effects have been validated by researchers, such as regulation of immune function, anti-mutagenic activity, anti-cytotoxic activity and anti-tumorigenic activity.8,10,11 In addition, the EPSs produced by yeast can be separated easily from the culture broth. Therefore, if we can realize the co-production of EPSs, carotenoids and oils by a yeast strain, the relative production costs of the oil will be markedly reduced because of an increase in the production of other valuable co-products.
The aim of this study was to develop a different way to share the production costs of the microbial oil by co-producing valuable EPSs and carotenoids by S. pararoseus. This study lays the foundation for the comprehensive use of S. pararoseus.
2 Materials and methods
2.1 Strain and cultivation conditions
In this study, the strain S. pararoseus JD-2 (preservation number: CCTCC M2010326) was used and isolated in our laboratory. The cultivation of S. pararoseus was described in our previous study.6
Medium composition for seed culture consisted of the following (g L−1): glucose, 30; corn steep liquor (CSL), 20; KH2PO4, 1; MgSO4·7H2O, 0.5. The batch fermentations were carried out in 500 mL Erlenmeyer flasks with 50 mL of media, which consisted of the following (g L−1): glucose, 30–360; corn steep liquor (CSL), 0–40; yeast extract (YE), 0–40; K2HPO4, 1; MgSO4·7H2O, 1. All the reagents for the medium were bought from Sinopharm, China. The initial pH was adjusted to 6.0 and then, the seed-culture medium was sterilized at 121 °C for 20 min.
The fed-batch fermentations were carried out in a 5 L-jar fermenter (BLBio-5GJ-2-H, Bailun Bio-Technology Co. Ltd., Shanghai, China) containing 1 L of media with an inoculum size of 10% (v/v). The fermentation medium contained the following (g L−1): glucose, 80; CSL, 20; YE, 10; K2HPO4, 2; MgSO4·7H2O, 1.5. In the fed-batch fermentation process, the amount of residual sugar was controlled between 20 and 30 g L−1 by regulating the feeding substrate containing 800 g L−1 glucose. The dissolved oxygen levels were controlled between 20% and 30% of saturation by the adjustment of stirring speeds. All experiments were performed in triplicate.
2.2 Determination of EPS and oil production
Fermentation broth (100 mL) was centrifuged at 10
000 × g for 25 min (Beckman Coulter J-30I, CA, USA). The sample was mixed with 95% ethanol at a 1
:
2 (v/v) ratio and then, the sediment was collected by centrifugation at 10
000 × g for 25 min. The sediment was then freeze dried. Moreover, the cell pellets were used to extract oil, which were disrupted in 50 mL 0.5 mol L−1 HCl at 60 °C for 10 min. After HCl treatment, samples were centrifuged (10
000 × g for 10 min) and then, the pellets were used to extract oil. Oil was extracted by adding 20 mL ethyl acetate. The extraction was repeated 3 times at room temperature and the extracted solvent was evaporated in a rotary evaporator at 50 °C.
2.3 Analyzing the composition of fat soluble nutrients in S. pararoseus oil
The major components were quantified by high-performance liquid chromatography (HPLC; Hitachi L-2000, Japan) equipped with a photodiode array detector and a C18 column (25 mm × 4.6 mm; 4.6 μm particle size; Agilent, USA). Isocratic elution analysis was carried out with acetonitrile
:
tetrahydrofuran = 60
:
40 as described in our group's previous study.12 The fatty acid compositions were analyzed by 7820A gas chromatography equipped with a flame ionization detector and cross-linked capillary FFAP column (30 m × 0.32 mm × 0.4 m; Agilent, USA). The steps of the operation was carried out according to the description reported by Li et al.13
2.4 Analyzing the absolute molecular weight distribution of EPS
The Viscotek system (Malvern USA) was used to determine the molecular weight distribution of the EPS. The gel permeation chromatograph column was Malvern CLM3021 A6000M (ID 7.8 mm × L 300 mm) and the detectors were low angle scattering detector, right angle light scattering detector, viscometer – DP detector and refractive detector. Moreover, EPS was dissolved in 100 mmol L−1 NaNO3, and filtered through a 0.22 μm filter before injection. The mobile phase was composed of 100 mmol L−1 NaNO3 and the flow rate was 1 mL min−1 at a constant column temperature of 35 °C.9
2.5 Monosaccharide composition analysis
For monosaccharide composition analysis, 200 mL of EPS was hydrolyzed by 2 mL of 1 mol L−1 H2SO4 in boiling water for 4 h and neutralized by BaCO3. Hydrolyzed solution was used to determine the monosaccharide compositions by high performance anion-exchange chromatography (HPAEC) equipped with pulsed amperometric detection (ICS-5000, Dionex, USA).7 The neutral sugars were eluted in 5 mmol L−1 NaOH for 20 min and the uronic acids were eluted with NaAc with a gradient of 5–20 mmol L−1 within 21–30 min.
3 Results and discussion
3.1 The scheme for co-production of EPS and oil by S. pararoseus JD-2
Microbial oils accumulate in specific organelles-like lipid bodies within the cell,14 while the EPSs are secreted into fermentation broth.5 Moreover, the strain used in this study has the ability to accumulate the oils and EPSs.5,6 Therefore, EPSs and oils can be co-produced by fermentation with S. pararoseus JD-2, and they can be separated via centrifugation. The detail scheme for co-production of EPS and oil is listed in Fig. S1.†
3.2 Effects of initial concentration of glucose on the cultivation of S. pararoseus JD-2
Glucose is widely used as a carbon source for cell growth or product biosynthesis, such as amino acids, organic acids, and lipids.15,16 To test the influences of the initial concentration of glucose on the cultivation of S. pararoseus JD-2, the cell growth and the production of oil and EPS were investigated during the fermentation on different concentrations of glucose. As shown in Fig. 1, the production of oil and EPS were positively associated with the cell growth if the initial concentration of glucose was not more than 120 g L−1. However, the cell growth and the production of oil and EPS reduced gradually in media containing more than 120 g L−1 of glucose (Fig. 1). This is because the osmotic pressure of the culture medium increases on increasing the initial concentration of glucose, thus inhibiting the cell growth.17 Compared to the growth on media with other glucose concentrations, S. pararoseus JD-2 showed the highest cell growth, oil production and EPS production during the growth on media with 120 g L−1 of glucose, which are 40.5 ± 1.7 g L−1, 24.5 ± 1.6 g L−1, and 6.8 ± 0.3 g L−1, respectively. It should be noted that S. pararoseus JD-2 exhibits a great potential in production of oil and EPS as compared with other species (Fig. 1; for review, see ref. 14 and 18). Therefore, the strain S. pararoseus JD-2 has the potential to co-produce oil and EPS with glucose as the carbon source in an industrial process, and is worthy of further work.
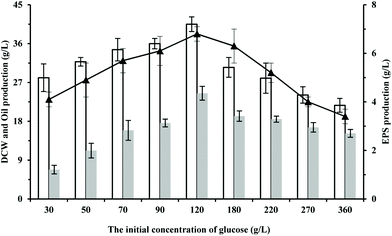 |
| Fig. 1 Effect of glucose concentrations on microbial oil and EPS production. White bar represents DCW, gray bar represents oil production, and “-▲-” represents EPS production. | |
3.3 Effect of organic nitrogen source on the cultivation of S. pararoseus JD-2
Corn steep liquor (CSL) and yeast extract (YE) are the preferred organic nitrogen sources for biomass growth and product biosynthesis.19 Numerous studies have indicated that nitrogen sources can significantly affect the total lipid content and the final biomass concentration, thus affecting the overall fermentation process.19,20 In order to study the effects of nitrogen sources on the production of oil and EPS in S. pararoseus JD-2, batch cultures were performed under different conditions of nitrogen availability. As shown in Fig. 2, the cell growth was increased during the addition of CLS or YE, and the highest cell growth was obtained with the addition of 20 g L−1 CLS and 10 g L−1 YE (45.3 ± 2.3 g L−1). In addition, the production of EPS also increased during the addition of appropriate amounts of CLS or YE, while YE was much better for EPS production than CLS. The maximum production of EPS (6.8 ± 0.6 g L−1) was obtained with the addition of 20 g L−1 CLS, while the production of EPS was up to 7.5 ± 0.5 g L−1 during addition of 20 g L−1 YE (Fig. 2). Interestingly, the production of oil was gradually reduced on increasing the concentration of YE, although the cell growth had a certain increase (Fig. 2). Similar results were also found in others studies,4,21 and they speculated that the increase of YE addition breaks the balance between carbon and nitrogen for accumulating oil.
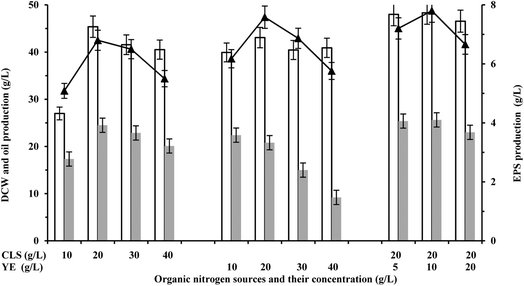 |
| Fig. 2 Effect of organic nitrogen sources on microbial oil and EPS production. White bar represents DCW, gray bar represents oil production, and “-▲-” represents EPS production. | |
Given that the CLS and YE have different effects on oil and EPS production, compound nitrogen sources containing CLS and YE were added to the fermentation medium and their effects on the fermentation progress were studied. The cell growth was significantly increased during the addition of compound nitrogen sources as compared with the addition of CLS or YE (Fig. 2). In addition, the production of oil and EPS also increased by various degrees (Fig. 2). The maximum biomass (48.3 ± 2.8 g L−1), EPS (7.8 ± 1.5 g L−1) and oil (25.6 ± 1.3 g L−1) were obtained with the addition of 20 g L−1 CLS and 10 g L−1 YE. Although the production of oil showed no remarkable change with compound nitrogen sources, the production of EPS was 14.7% higher than the case of using only 20 g L−1 CSL or 25.8% higher than the case of using only 10 g L−1 YE. Based on guidelines suggested by previous studies,22,23 the production of oil was restricted in the nitrogen-limited sugar-based media. However, 25.6 ± 1.3 g L−1 of oil was accumulated in nitrogen-rich media with 20 g L−1 CLS and 10 g L−1 YE, which was 5.3% higher than that using 20 g L−1 CSL or 14.8% higher than that using 10 g L−1 YE (Fig. 2). Li et al.13 and Ling et al.24 have realized the increase of oil production via high-density cultivation of oleaginous yeast. This perhaps is the most important reason that the oil production was increased in media with 20 g L−1 CLS and 10 g L−1 YE. Thus, addition of compound nitrogen sources containing 20 g L−1 CLS and 10 g L−1 YE is beneficial for co-production of oil and EPS.
3.4 Fed-batch fermentation
This previous research had demonstrated that the production capacity of oil and EPS by S. pararoseus JD-2 was negatively affected by osmotic pressure of fermentation broth (Fig. 1). In order to avoid the negative effects of osmotic pressure and to increase the yield and productivity of oil and EPS, numerous metabolic and process engineering techniques have been developed, such as substrate fed-batch fermentation.13,25
As shown in Fig. 3, the oil began to be produced at the initial stage and continuously increased during the entire fermentation period to obtain a final production of 41.6 ± 2.5 g L−1. This result is different from the previous study using Cryptococcus curvatus O3 as production strain, which had no oil storage at initial stage.26 The overall oil productivity was 0.58 g L−1 h−1 and the oil coefficient was 0.257 g-oil per g-glucose, which were higher than that achieved via batch fermentation (0.58 g L−1 h−1 vs. 0.36 g L−1 h−1 and 0.257 g-oil per g-glucose vs. 0.213 g-oil per g-glucose, respectively). These results indicated that the oil production could be improved by optimizing the control of the substrate feeding process. Although the total production of oil in this study was lower than those obtained in other studies, the productivity was satisfactory (Table 1). It should be noted that the titer will increase over time, but long fermentation times are unfavorable for the industrial-scale production of oil because of the decrease in the equipment capacity utilization.27 The productivity of oil by Lipomyces starkeyi AS 2.1560 was the highest ever reported,22 but the authors had used a different computing mode, in which the cultivating time of organism was unaccounted. In addition, oil coefficient (gram oil per gram substrate) is one of the most important parameters for microbial oil technology, and the theoretical oil coefficient was estimated as 0.32 g oil per g glucose.28 Our study gave an oil coefficient of 0.257 g-oil per g-glucose, which was slightly lower than that of the engineered strain Yarrowia lipolytica ADgm-hi reported by Qiao et al.29 These results suggest that the production of oil by S. pararoseus JD-2 can be achieved in industrial applications using substrate feeding strategies.
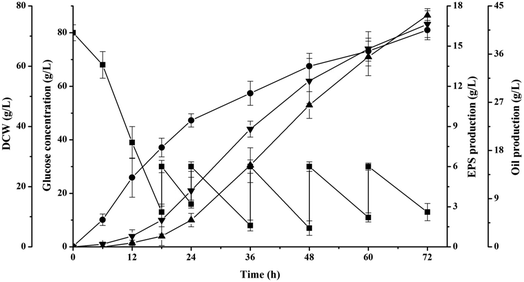 |
| Fig. 3 Fed-batch fermentation profile for S. pararoseus JD-2. Symbol: (■) glucose, (●) DCW, (▲) EPS, and (▼) oil. | |
Table 1 Comparison of oil production in various microalgae, fungi and oleaginous yeasts with glucose as the sole carbon source by fed-/batch fermentation
Strains |
Fermentation mode |
DCW (g L−1) |
Titer (g L−1) |
Oil content (%) |
Coefficient (g g−1) |
Productivity (g L−1 h−1) |
References |
— not indicated. |
Chlorella sorokiniana FC6 IITG |
Fed-batch |
15.81 |
8.69 |
55 |
—a |
0.23 |
41 |
Mucor circinelloides URM 4182 |
Batch |
4.23 |
1.88 |
44 |
0.094 |
0.20 |
42 |
Neurospora crassa 2489 |
Batch |
21.0 |
1.09 |
5.5 |
0.055 |
0.02 |
43 |
Yarrowia lipolytica AD |
Fed-batch |
87.4 |
47.7 |
54.6 |
0.184 |
0.40 |
29 |
Yarrowia lipolytica ADgm-hi |
Fed-batch |
148.0 |
98.9 |
83.8 |
0.269 |
1.20 |
29 |
Lipomyces starkeyi AS 2.1560 |
Fed-batch |
104.6 |
67.9 |
64.9 |
0.235 |
1.60 |
22 |
Cryptococcus curvatus O3 |
Fed-batch |
104.1 |
86.1 |
82.7 |
0.246 |
0.47 |
26 |
Rhodosporidium toruloides Y4 |
Fed-batch |
106.5 |
71.8 |
67.5 |
0.234 |
0.54 |
13 |
Rhodotorula glutinis BCRC 22360 |
Fed-batch |
45.4 |
19.1 |
42.1 |
0.226 |
0.28 |
44 |
Sporidiobolus pararoseus JD-2 |
Batch |
48.3 ± 2.8 |
25.6 ± 1.3 |
53.0 |
0.213 |
0.36 |
This study |
Sporidiobolus pararoseus JD-2 |
Fed-batch |
72.1 ± 3.2 |
41.6 ± 2.5 |
57.7 |
0.257 |
0.58 |
This study |
Moreover, it was clear that EPS accumulated quickly after 18 h of cultivation, and the EPS content increased with a high average EPS productivity of 0.182 g L−1 h−1, which was much higher than the reported values in previous studies (Table 2). Gientka et al.30 indicated that yeast metabolism was clearly directed toward the synthesis of EPS rather than toward the synthesis of intracellular components and biomass at specific times; also, different yeasts had different occurrence times. In addition, the production of EPS was 13.1 ± 0.6 g L−1 in fed-batch fermentation, which was 67.9% higher than bath fermentation (the titer of 7.8 ± 1.5 g L−1). This is because the high-density cultivation and the precise regulation of fermentation process can be achieved in fed-batch fermentation.13
Table 2 Comparison of exopolysaccharides (EPSs) production in various fungi and oleaginous yeasts with glucose or sucrose as the sole carbon source by fed-/batch fermentation
Strains |
Fermentation mode |
Titer (g L−1) |
Coefficient (g per g glucose) |
Productivity (g L−1 h−1) |
References |
Cultured with sucrose as the sole carbon source, and it was converted to glucose to calculate the coefficient. |
Phellinus nigricans |
Batch |
1.89 ± 0.13 |
0.036 |
0.013 |
45 |
Ganoderma lucidum |
Batch |
15 |
0.375 |
0.030 |
46 |
Sporobolomyces salmonicolor AL1 |
Batcha |
5.6 ± 0.3 |
0.106 |
0.047 |
47 |
Cryptococcus laurentii AL100 |
Batcha |
6.4 ± 0.2 |
0.152 |
0.067 |
48 |
Cryptococcus flavus A51 |
Batcha |
5.75 ± 0.04 |
0.109 |
0.040 |
9 |
Rhodotorula acheniorum MC |
Fed-batcha |
6.2 |
0.118 |
0.064 |
49 |
Rhodotorula glutinis KCTC 7989 |
Fed-batch |
4.0 |
0.133 |
0.021 |
50 |
Rhodotorula glutinins |
Batch |
2.6 |
0.087 |
0.015 |
51 |
Sporidiobolus pararoseus JD-2 |
Batch |
7.8 ± 1.5 |
0.065 |
0.108 |
This study |
Sporidiobolus pararoseus JD-2 |
Fed-batch |
13.1 ± 0.6 |
0.081 |
0.182 |
This study |
By comparing the production of oil and EPS by different yeasts (Tables 1 and 2), we can determine that S. pararoseus JD-2 can be used as a work-horse for industrial co-production of oil and EPS using substrate feeding strategies.
3.5 The fat soluble nutrients in S. pararoseus oil
In this study, we separated and identified the carotenoids in S. pararoseus oil with silica gel plates (Merck HF-254) and LC-MS. Four types of carotenoids were separated: torularhodin, torulene, γ-carotene, and β-carotene (Fig. S2–S4;† Table 3). Carotenoids, squalene and ergosterol belong to terpenoids, which are synthesized through mevalonate pathway.31 We have also determined their amounts in the oil, and their detailed characteristics are described in Table 3. Although the amount of carotenoids (approximately 500 mg per kg oil) is lower than that reported by Dias et al.,32 torulene was the primary ingredient of carotenoids (up to 294 ± 6.5 mg per kg oil), which was much higher than that observed in seed oils.33 In addition, the amount of squalene was 1.32 ± 0.4 g per kg oil. The concentration of total ergosterol was 4.92 g per kg oil, of which 17.3% of the ergosterol existed in the form of ergosterol ester (Table 3). Interestingly, the components of carotenoids could be varied by culture time (Table 4). γ-Carotene serves as a precursor for synthesizing β-carotene and torulene either by cyclizing at isoprene end of γ-carotene to synthesize β-carotene or by dehydrogenizing at 3′ and 4′ of γ-carotene to synthesize torulene.34 It is known that the unsaturation of torulene is lower than that of β-carotene.35 As observed from Table 4, the amount of oil increased over time, which was consistent with the previous experimental result (for review, see ref. 14). However, the intracellular oxidative stress will increase with oil content, thus redirecting the carbon flux to synthesize the low unsaturated torulene.
Table 3 Characteristics of the main components in S. pararoseus oil
No. |
Name |
Rf value of TLC solvent systema |
λmax (nm) |
Mol. wt. (M) |
Content (per kg oil) |
The content in brackets shows the developing solvents and their ratio. |
1 |
Squalene |
0.94 (Vn-hexane : Vacetone = 50 : 1) |
208 |
410 |
1.32 ± 0.4 g |
2 |
β-Carotene |
0.82 (Vn-hexane : Vacetone = 50 : 1) |
426, 452, 478 |
536 |
59 ± 3.7 mg |
3 |
γ-Carotene |
0.58 (Vn-hexane : Vacetone = 50 : 1) |
434, 461, 490 |
536 |
74 ± 1.3 mg |
4 |
Ergosterol esters |
0.55 (Vn-hexane : Vacetone = 50 : 1) |
283 |
— |
0.85 ± 0.3 g |
5 |
Torulene |
0.34 (Vn-hexane : Vacetone = 50 : 1) |
462, 484, 516 |
534 |
294 ± 6.5 mg |
8 |
Ergosterol |
0.47 (Vn-hexane : Vdiethyl ether : Vmethane acid = 80 : 30 : 1) |
283 |
396 |
4.07 ± 0.5 g |
9 |
Torularhodin |
0.64 (Vn-hexane : Vacetone = 3 : 2) |
471, 495, 525 |
564 |
78 ± 7.7 mg |
Table 4 The components of carotenoids in S. pararoseus oil by fed-batch fermentation
Time |
Torulene (%) |
γ-Carotene (%) |
β-Carotene (%) |
Oil content (%) |
DCW (g L−1) |
Carotenoids production |
mg per kg oil |
mg per kg DCW |
mg L−1 |
24 h |
29.5 ± 0.5 |
26.6 ± 0.5 |
21.2 ± 0.3 |
20 ± 1.3 |
42.2 ± 2.2 |
651 ± 6.5 |
78 ± 2.1 |
1.56 ± 0.2 |
36 h |
37.47 ± 1.7 |
23.0 ± 0.5 |
18.7 ± 0.6 |
25 ± 1.6 |
51.8 ± 4.0 |
645 ± 3.2 |
129 ± 2.8 |
5.16 ± 1.5 |
48 h |
52.7 ± 1.0 |
15.9 ± 0.4 |
12.1 ± 0.5 |
32 ± 0.9 |
60.4 ± 4.3 |
512 ± 4.6 |
128 ± 2.3 |
7.04 ± 1.7 |
72 h |
57.8 ± 1.4 |
14.6 ± 0.7 |
11.6 ± 0.2 |
55 ± 1.5 |
72.1 ± 3.2 |
508 ± 2.8 |
193 ± 4.5 |
13.90 ± 3.2 |
As observed from Table 3 and Fig. S5,† S. pararoseus JD-2 can also accumulate significant amounts of fatty acids (FAs). The crude oil primarily constitutes of 79.19% unsaturated FAs, most of which was oleic acid (18:1n − 9) at a level of 73.2 g per 100 g total FAs (Table 5). Moreover, Deng et al.36 indicated that the FAs profiles in ergosterol esters and triglycerides from Ganoderma lucidum were much different. In order to know the FAs patterns in ergosteryl esters and triglycerides from S. pararoseus JD-2, the FAs compositions and their relative amounts in ergosteryl esters and triglycerides were determined in this study. Unlike the previous results reported by Deng et al.,36 the percentage of unsaturated FAs in ergosteryl esters was higher than that in triglycerides from S. pararoseus JD-2 (92.15% vs. 79.15%; Table 5). Although C18:1 (Z)-9-octadecanoic acid was the predominant FA in ergosteryl esters and triglycerides, the percentages of C16:0 hexadecanoic acid and (Z,Z)-9,12-octadecanoic acid in ergosteryl esters and triglycerides showed an enormous difference. C16:0 hexadecanoic acid constituted 5.57% of the total fatty acids in ergosteryl esters and 17.4% in triglycerides. In contrast, (Z,Z)-9,12-octadecanoic acid constituted 22.3% of the total fatty acids in ergosteryl esters and 3.52% in triglycerides (Table 5). These results were in accordance with the previous results, indicating that C16:0 is the main saturated FA in triglycerides.37
Table 5 The fatty acid composition and its relative content in ergosterol esters and triglyceride from S. pararoseus oila
Composition and its relative content (%) |
Crude oil |
Ergosterol esters |
Triglycerides |
— Not detected. |
C14:0 tetradecanoic acid |
0.83 |
0.80 |
0.82 |
C16:0 hexadecanoic acid |
17.3 |
5.57 |
17.4 |
C16:1 (Z)-9-hexadecanoic acid |
0.81 |
1.39 |
0.78 |
C18:0 octadecanoic acid |
1.31 |
1.5 |
1.30 |
C18:1 trans-9-octadecenoic acid |
0.13 |
— |
0.13 |
C18:1 (Z)-9-octadecanoic acid |
73.2 |
65.8 |
74.0 |
C18:2 (Z,Z)-9,12-octadecanoic acid |
4.24 |
22.3 |
3.52 |
C20:0 eicosenoic acid |
0.36 |
— |
0.37 |
C20:1 11-eicosenoic acid |
0.52 |
1.72 |
0.52 |
C18:3 α-linolenic acid |
0.29 |
0.94 |
0.20 |
C22:0 behenic acid |
0.46 |
— |
0.48 |
C24:0 pyroligneous acid |
0.43 |
— |
0.46 |
3.6 The monosaccharide composition of S. pararoseus EPS
Zeta-potential analysis indicated that the EPS produced by S. pararoseus JD-2 is an anionic polyelectrolyte (data not shown), thus anion-exchange chromatography must be used to purify it. As shown in Fig. 4, the EPS produced by S. pararoseus JD-2 contained only one type of polysaccharide. The number-average molecular weight (Mn) of the polysaccharide was about 1.3 × 106, while the weight-average molecular weight (Mw) was approximately 1.8 × 106. Following this, the monosaccharide composition of EPS was also analyzed by HPAEC, indicating that galactose, glucose and mannose were the main components in EPS, which were in a proportion of 16
:
8
:
1 (Fig. 5; Table S1†). Moreover, uronic acids were not present in the EPS produced by S. pararoseus JD-2. Previous studies have proved that the monosaccharides contributing to their EPS production were diverse in different yeasts and even in the same yeast because the composition can be influenced by various factors, such as the culture medium and culture conditions.38,39 Mannose was generally the dominant type of monosaccharide in the EPS produced by yeast (Table S1†). However, galactose was the main monosaccharide of EPS produced by S. pararoseus JD-2 (Fig. 5). We then infer that EPS produced by S. pararoseus JD-2 was a new EPS, which might have new chemical and physical characteristics because of the different polymerization and properties of synthesized polymer.
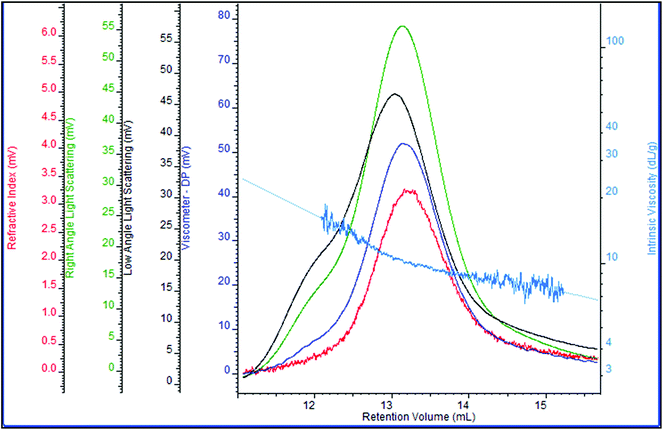 |
| Fig. 4 Distribution of molecular weight and molecular viscosity of EPS produced by S. pararoseus JD-2. | |
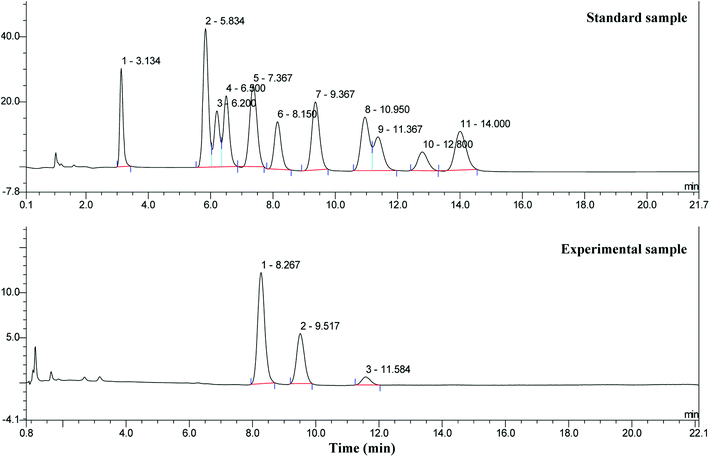 |
| Fig. 5 HPAEC results of hydrolyzed EPS produced by S. pararoseus JD-2. The chromatographic peaks of standard sample: peak 1 – fucose (3.134 min); peak 2 – glucosamine (5.834 min); peak 3 – rhamnose (6.200 min); peak 4 – arabinose (6.500 min); peak 5 – galactosamine (7.367 min); peak 6 – galactose (8.150 min); peak 7 - glucose (9.367 min); peak 8 – xylose (10.950 min); peak 9 – mannose (11.367 min); peak 10 – fructose (12.800 min); peak 11 – ribose (14.000 min). | |
4 Conclusion
Yeasts are promising producers of industrially relevant chemicals, including microbial oil and its derived chemicals.40 However, the production costs of microbial oil are currently higher than that of vegetable oil. In this study, we developed a new way to share the production costs of the oil by selecting a new yeast strain to co-produce oil and EPS. The strain S. pararoseus JD-2 produced an oil titer, oil productivity, EPS titer, and EPS productivity of up to 41.6 ± 2.5 g L−1, 0.58 g L−1 h−1, 13.1 ± 0.6 g L−1, and 0.182 g L−1 h−1, respectively, during in the fed-batch culture for 72 h. These results indicate that S. pararoseus JD-2 can be used as a work-horse for co-producing microbial oil and EPS. In addition, fatty acid composition of S. pararoseus oil and monosaccharide composition of S. pararoseus EPS were determined, which indicated that EPS produced by S. pararoseus JD-2 was a new type of EPS. Further studies could analyze the structure of the EPS and other major compositions in the oil. The physiological effects of EPS and oil should also be studied.
Conflicts of interest
There are no conflicts to declare.
Acknowledgements
This study was supported by the National Natural Science Foundation of China (Grant number 31601459), Natural Science Foundation of Jiangsu Province (Grant number BK20150149), China Postdoctoral Science Foundation Grant (Grant number 2016M590410).
References
- Q. Li, W. Du and D. Liu, Appl. Microbiol. Biotechnol., 2008, 80, 749–756 CrossRef CAS PubMed.
- X. Meng, J. Yang, X. Xu, L. Zhang, Q. Nie and M. Xian, Renewable Energy, 2009, 34, 1–5 CrossRef CAS.
- Q. Ye, X. Li, M. Yan, H. Cao, L. Xu, Y. Zhang, Y. Chen, J. Xiong, P. Ouyang and H. Ying, Appl. Microbiol. Biotechnol., 2010, 87, 517–525 CrossRef CAS PubMed.
- E. U. Kiran, A. Trzcinski and C. Webb, Bioresour. Technol., 2013, 129, 650–654 CrossRef PubMed.
- M. Han, C. Du, Z. Y. Xu, H. Qian and W. G. Zhang, Int. J. Biol. Macromol., 2016, 88, 603–613 CrossRef CAS PubMed.
- M. Han, Z. Y. Xu, C. Du, H. Qian and W. G. Zhang, Bioprocess Biosyst. Eng., 2016, 39, 1425–1433 CrossRef CAS PubMed.
- O. Ates, Front. Bioeng. Biotechnol., 2015, 3, 200 Search PubMed.
- G. Caggianiello, M. Kleerebezem and G. Spano, Appl. Microbiol. Biotechnol., 2016, 100, 3877–3886 CrossRef CAS PubMed.
- K. Pavlova, I. Panchev, M. Krachanova and M. Gocheva, Folia Microbiol., 2009, 54, 343–348 CrossRef CAS PubMed.
- G. Kogani, M. Pajtinka, M. Babincova, E. Miadokova, P. Rauko, D. Slamenova and T. Korolenko, Neoplasma, 2008, 55, 387 Search PubMed.
- M. Rondanelli, A. Opizzi and F. Monteferrario, Minerva Med., 2009, 100, 237–245 CAS.
- Q. Shi, H. Wang, C. Du, W. Zhang and H. Qian, Anal. Sci., 2013, 29, 997–1002 CrossRef CAS PubMed.
- Y. H. Li, Z. B. Zhao and F. W. Bai, Enzyme Microb. Technol., 2007, 41, 312–317 CrossRef CAS.
- L. Qin, L. Liu, A. P. Zeng and D. Wei, Bioresour. Technol., 2017, 245, 1507–1519 CrossRef CAS PubMed.
- R. Poontawee, W. Yongmanitchai and S. Limtong, Process Biochem., 2017, 53, 44–60 CrossRef CAS.
- J. Z. Xu, M. Han, J. L. Zhang, Y. F. Guo and W. G. Zhang, Amino Acids, 2014, 46, 2165–2175 CrossRef CAS PubMed.
- J. Z. Xu, X. H. Xia, J. L. Zhang, Y. F. Guo and W. G. Zhang, J. Microbiol. Biotechnol., 2014, 24, 1368–1376 CrossRef CAS PubMed.
- A. Poli, P. Di Donato, G. R. Abbamondi and B. Nicolaus, Archaea, 2011, 2011, 1–13 CrossRef PubMed.
- C. Capusoni, V. Rodighiero, D. Cucchetti, S. Galafassi, D. Bianchi, G. Franzosi and C. Compagno, Bioresour. Technol., 2017, 238, 281–289 CrossRef CAS PubMed.
- R. R. Bommareddy, W. Sabra, G. Maheshwari and A. P. Zeng, Microb. Cell Fact., 2015, 14, 36–49 CrossRef PubMed.
- E. Z. Vega, B. A. Glatz and E. G. Hammond, Appl. Environ. Microbiol., 1988, 54, 748–752 CAS.
- J. T. Lin, H. W. Shen, H. D. Tan, X. Zhao, S. G. Wu, C. M. Hu and Z. B. K. Zhao, J. Biotechnol., 2011, 152, 184–188 CrossRef CAS PubMed.
- I. Ajjawi, J. Verruto, M. Aqui, L. B. Soriaga, J. Coppersmith, K. Kwok, L. Peach, E. Orchard, R. Kalb, W. D. Xu, T. J. Carlson, K. Francis, K. Konigsfeld, J. Bartalis, A. Schultz, W. Lambert, A. S. Schwartz, R. Brown and E. R. Moellering, Nat. Biotechnol., 2017, 35, 647–655 CrossRef CAS PubMed.
- J. Y. Ling, S. Nip and H. Shim, Bioresour. Technol., 2013, 146, 301–309 CrossRef CAS PubMed.
- X.-Y. Ge, J. Yuan, H. Qin and W.-G. Zhang, Appl. Microbiol. Biotechnol., 2011, 89, 73–78 CrossRef CAS PubMed.
- J. Zhang, X. Fang, X. L. Zhu, Y. Li, H. P. Xu, B. F. Zhao, L. Chen and X. D. Zhang, Biomass Bioenergy, 2011, 35, 1906–1911 CrossRef CAS.
- J. Becker, O. Zelder, S. Hafner, H. Schroder and C. Wittmann, Metab. Eng., 2011, 13, 159–168 CrossRef CAS PubMed.
- C. Ratledge and J. P. Wynn, Adv. Appl. Microbiol., 2002, 51, 1–52 CAS.
- K. J. Qiao, T. M. Wasylenko, K. Zhou, P. Xu and G. Stephanopoulos, Nat. Biotechnol., 2017, 35, 173–177 CrossRef CAS PubMed.
- I. Gientka, A. Bzducha-Wrobel, L. Stasiak-Rozanska, A. A. Bednarska and S. Blazejak, Electron. J. Biotechnol., 2016, 22, 31–37 CrossRef CAS.
- S. C. Kampranis and A. M. Makris, Comput. Struct. Biotechnol. J., 2012, 3, e201210006 CrossRef PubMed.
- C. Dias, S. Sousa, J. Caldeira, A. Reis and T. Lopes da Silva, Bioresour. Technol., 2015, 189, 309–318 CrossRef CAS PubMed.
- J. Parry, Z. Hao, M. Luther, L. Su, K. Zhou and L. L. Yu, J. Am. Oil Chem. Soc., 2006, 83, 847–854 CrossRef CAS.
- L. Zoz, J. C. Carvalho, V. T. Soccol, T. C. Casagrande and L. Cardoso, Braz. Arch. Biol. Technol., 2015, 58, 278–288 CrossRef.
- L. C. Mata-Gomez, J. C. Montanez, A. Mendez-Zavala and C. N. Aguilar, Microb. Cell Fact., 2014, 13, 12–23 CrossRef PubMed.
- Z. L. Deng, J. P. Yuan, Y. Zhang, X. M. Xu, C. F. Wu, J. Peng and J. H. Wang, J. Am. Oil Chem. Soc., 2013, 90, 1495–1502 CrossRef CAS.
- T. Ferreira, M. Regnacq, P. Alimardani, C. Moreau-Vauzelle and T. Berges, Biochem. J., 2004, 378, 899–908 CrossRef CAS PubMed.
- R. McKellar, J. Van Geest and W. Cui, Food Hydrocolloids, 2003, 17, 429–437 CrossRef CAS.
- R. Triveni, T. Shamala and N. Rastogi, Process Biochem., 2001, 36, 787–795 CrossRef CAS.
- Y. J. Zhou, N. A. Buijs, Z. Zhu, J. Qin, V. Siewers and J. Nielsen, Nat. Commun., 2016, 7, 11709 CrossRef CAS PubMed.
- V. Kumar, M. Muthuraj, B. Palabhanvi, A. K. Ghoshal and D. Das, Bioresour. Technol., 2014, 170, 115–124 CrossRef CAS PubMed.
- A. K. Carvalho, J. D. Rivaldi, J. C. Barbosa and H. F. de Castro, Bioresour. Technol., 2015, 181, 47–53 CrossRef CAS PubMed.
- C. M. Roche, N. L. Glass, H. W. Blanch and D. S. Clark, Biotechnol. Bioeng., 2014, 111, 1097–1107 CrossRef CAS PubMed.
- H. W. Yen and Z. Zhang, J. Biosci. Bioeng., 2011, 112, 71–74 CrossRef CAS PubMed.
- Z. Y. Wang, Y. Quan and F. Zhou, Carbohydr. Polym., 2014, 105, 200–206 CrossRef CAS PubMed.
- L. Papinutti, Bioresour. Technol., 2010, 101, 1941–1946 CrossRef CAS PubMed.
- S. Dimitrova, K. Pavlova, L. Lukanov, E. Korotkova, E. Petrova, P. Zagorchev and M. Kuncheva, Appl. Biochem. Biotechnol., 2013, 169, 301–311 CrossRef CAS PubMed.
- K. Pavlova, S. Rusinova-Videva, M. Kuncheva, M. Kratchanova, M. Gocheva and S. Dimitrova, Appl. Biochem. Biotechnol., 2011, 163, 1038–1052 CrossRef CAS PubMed.
- K. Pavlova, I. Panchev and T. Hristozova, World J. Microbiol. Biotechnol., 2005, 21, 279–283 CrossRef CAS.
- D. H. Cho, H. J. Chae and E. Y. Kim, Appl. Biochem. Biotechnol., 2001, 95, 183–193 CrossRef CAS PubMed.
- S. I. Ghada, G. M. Manal, M. Mohsen and A. G. Eman, Aust. J. Basic Appl. Sci., 2012, 6, 401–408 Search PubMed.
Footnote |
† Electronic supplementary information (ESI) available. See DOI: 10.1039/c7ra12813d |
|
This journal is © The Royal Society of Chemistry 2018 |