DOI:
10.1039/C8QM00309B
(Research Article)
Mater. Chem. Front., 2018,
2, 1893-1899
Luminescent supramolecular polymer nanoparticles for ratiometric hypoxia sensing, imaging and therapy†
Received
27th June 2018
, Accepted 7th August 2018
First published on 8th August 2018
Abstract
Hydrogen-bonded supramolecular polymer nanoparticles (SPNPs), serving as a ratiometric oxygen nanoprobe for hypoxia sensing and photodynamic therapy (PDT) treatment, were designed. Herein, oxygen-sensitive phosphorescent Pt(II) porphyrin moiety and oxygen-insensitive fluorescent 9,10-diphenylanthracene moiety were assembled through quadruple hydrogen bonding into SPNPs. The efficient energy transfer between the monomeric donor–acceptor pairs in the SPNPs leads to enhanced phosphorescence with long lifetimes. The resulting bright phosphorescence can realize hypoxia sensing in living cells and generate singlet oxygen efficiently in SPNPs to kill cancer cells. This is the first example of a multi-functional supramolecular polymer-based nanoprobe for ratiometric hypoxia sensing, imaging and PDT treatment.
Introduction
Molecular oxygen (O2) is a critical component to provide metabolic energy to cells in aerobic respiration.1 Hypoxia, a condition in which O2 levels are below normal, is related to many diseases including cancer, neurological disorders and retinal diseases.2 Thus, the determination of O2 levels is of significance in diagnosing cancers and understanding the cellular function and etiology of diseases at their early stage. Optical O2 sensors provide a powerful tool for sensing and mapping the presence of O2 in cells and tissues.3 Ratiometric O2 sensing, in which the O2 level is calculated using the ratio of O2-insensitive fluorescence to O2-sensitive phosphorescence, further allows more accurate measurement and avoids background interference.3 Currently, there is great interest in the development of ratiometric optical oxygen nanoprobes by incorporating metal porphyrin complexes with conjugated polymer nanoparticles (CPNs), silica gels and quantum dots.4–7 Phosphorescence quenching of the indicator by the ground triplet state of O2, in principle, can result in the generation of singlet oxygen (1O2). 1O2 is highly toxic to cells and is widely used in photodynamic therapy (PDT) for cancer treatment. Additionally, photosensitizers such as metal porphyrins, BODIPY and bacteriochlorin derivatives, which are able to react with O2 to produce 1O2, have been demonstrated to possess therapeutic abilities, some of which have obtained approval for clinical use.8,9 Thus, it is quite possible to develop a system capable of both hypoxia sensing and PDT treatment. Although such integrative system for diagnosis and therapy is highly desirable in the biomedical field, it is rather challenging owing to the difficulty in combining all the essential features under hypoxia conditions. To date, only a few oxygen sensors based on CPNs have been reported to realize multiple functions for both hypoxia sensing, imaging and treatment.7 However, these sensors contain polymers that require tedious synthesis procedures and are probably not biodegradable.
Supramolecular polymers are a class of polymers that are assembled via low-molecular-weight monomers through reversible non-covalent interactions.10–13 Previously, we developed ureidopyrimidinone (UPy)-based quadruple hydrogen bonded supramolecular polymer nanoparticles (SPNPs).14 SPNPs exhibit the same merits of CPNs, such as excellent photostability and good biocompatibility. Moreover, SPNPs possess several unique characteristics. (1) The functionalities of SPNPs are mainly determined by their small monomeric units, and they can be finely tuned through synthetic manipulations.11a,b (2) The noncovalent interactions between their small molecular monomers endow the SPNPs potential biodegradability after a reasonable period. (3) SPNPs with a uniform small particle size can be readily dispersed in aqueous solution and can penetrate cells.14c (4) The occurrence of fluorescence resonance energy transfer (FRET) between the appropriate donors and acceptors built into SPNP has been established.14a,b
In the present study, we designed a supramolecular polymer nanoparticle (SPNP)-based O2 nanoprobe for ratiometric hypoxia sensing and cancer therapy. An oxygen-insensitive fluorescence reference moiety bearing bis-ureidopyrimidinone (bis-UPy) acts as the energy donor (D), and an oxygen-sensitive phosphorescence indicator moiety bearing bis-UPy acts as the energy acceptor (A). We assembled these fluorescence and phosphorescence monomeric units into SPNPs through quadruple hydrogen bonding between UPy. The resulting SPNPs can be readily dispersed in aqueous solution and can penetrate cells. Efficient FRET among the D–A pairs in the SPNPs is greatly facilitated, resulting in drastic phosphorescence enhancement. Importantly, the triplet–triplet energy transfer (TTET) from the phosphorescent SPNPs to O2 enables sensitive ratiometric hypoxia sensing both in aqueous solution and in living cells as well as effective apoptosis of cancer cells by the generated 1O2 (Fig. 1). The results presented demonstrate that SPNPs are excellent luminescent nanoprobes for practical application in determining O2 concentration in biological systems and effective PDT agents in the treatment of cancer cells.
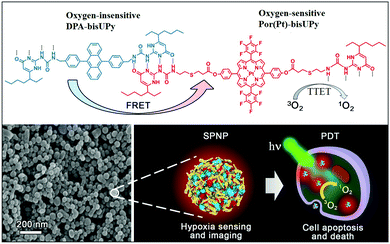 |
| Fig. 1 Chemical structures of Por(Pt)-bisUPy and DPA-bisUPy. SEM image of SPNPs and schematic of the dual-emissive SPNPs for hypoxia sensing, imaging and PDT treatment. | |
Results and discussion
Assembly and characterization of SPNPs
The SPNPs we developed for ratiometric O2 sensing with FRET mediated phosphorescence contain two components: a UPy-containing 9,10-diphenyl anthracene unit (DPA-bisUPy) and a UPy-containing fluorinated phenyl of Pt(II) porphyrin [Por(Pt)-bisUPy]. The former acts as the fluorescence reference dye and energy donor and the latter as a phosphorescence indicator and energy acceptor. Por(Pt)-bisUPy was synthesized in ∼60% yield via the facile Michael addition of UPy-SH to alkene substituted Pt(II) porphyrin complexes at room temperature. Por(Pt)-bisUPy was characterized thoroughly via1H/13C NMR and HR-MS (see Experimental section and ESI† for details). DPA-bisUPy was synthesized in high yield from 9,10-dibromoanthracene in a few steps. The water-dispersible nanoparticles containing two UPy monomers were prepared following a previously reported method.14a The important role of the strong quadruple hydrogen bonding (Ka = 107 M−1 in CHCl3) between UPy in construction of SPNPs has been established.14a SPNPs with different molar ratios of Por(Pt)-bisUPy and DPA-bisUPy (1
:
30, 1
:
40, and 1
:
60) were prepared. The morphological studies conducted via transmission electron microscopy (TEM) and scanning electron microscopy (SEM) show that stable nanospheres with an average diameter of 70 nm were formed using different molar ratios of DPA-bisUPy and Por(Pt)-bisUPy. The dynamic light scattering measurements (DLS) indicate that the hydrodynamic diameter of the SPNPs is ca. 96 nm. The SPNPs stabilized by cationic cetyltrimethyl ammonium bromide (CTAB) surfactant have a zeta potential of +16.1 mV. Furthermore, no significant changes in the size and charge of the nanoparticles with different ratios of DPA-bisUPy and Por(Pt)-bisUPy were observed (Fig. 2a, b and Fig. S1, ESI†).
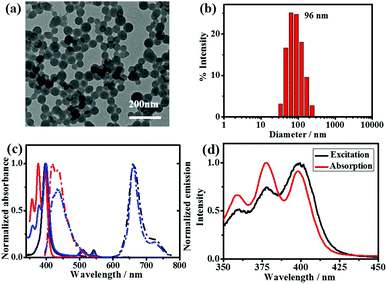 |
| Fig. 2 TEM image (a) and DLS analysis (b) of SPNPs with a molar ratio of 40 : 1 DPA-bisUPy and Por(Pt)-bisUPy. (c) Normalized absorption and emission spectra of monomers DPA-bisUPy (c = 1.6 × 10−5 M) and Por(Pt)-bisUPy (c = 3.0 × 10−6 M) in chloroform and SPNPs (D/A = 40/1) in aqueous solution (c = 1.7 × 10−6 M). Black line: absorption of Por(Pt)-bisUPy, red line: absorption of DPA-bisUPy, blue line: absorption of SPNPs, black dotted line: phosphorescence of Por(Pt)-bisUPy (λex = 400 nm), red dotted line: fluorescence of DPA-bisUPy (λex = 375 nm) and blue dotted line: emission of SPNPs (λex = 375 nm). (d) Normalized excitation spectrum of SPNPs-dispersed aqueous solution ([D]/[A] = 60 : 1) and normalized absorption spectrum of DPA-bisUPy in CHCl3. | |
Photophysical studies of SPNPs
Fig. 2c shows the absorption spectra of the SPNPs composed of Por(Pt)-bisUPy and DPA-bisUPy in aqueous solution. The absorption bands at 350–430 nm are attributed to DPA-bisUPy and the Soret band of the Por(Pt)-bisUPy moiety. The bands at 510 nm and 542 nm are attributed to the Q (1,0) and Q (0,0) bands of the Por(Pt)-bisUPy moiety, respectively. Excitation of the SPNPs at 400 nm resulted in fluorescence at 434 nm from DPA-bisUPy and phosphorescence at 660 nm from Por(Pt)-bisUPy. Owing to the substantial overlap between the absorption bands of Por(Pt)-bisUPy (375–430 nm) and the emission bands of DPA-bisUPy (400–550 nm) as well as the short D–A distance resulting from the hydrogen bonding interactions in SPNPs, the FRET process from DPA-bisUPy to Por(Pt)-bisUPy was confirmed by recording the emission spectra of SPNPs with different ratios of donor and acceptor while maintaining the same absorption intensity at 375 nm (Fig. S2a, ESI†). As shown in Fig. S2b (ESI†), upon selective excitation at 375 nm, an increase in the A/D molar ratio from 1/60 to 1/30 lowered the fluorescence intensity at 434 nm, but enhanced the phosphorescence intensity at 660 nm. The excitation spectrum of the SPNPs at 660 nm matches the absorption spectrum of DPA-bisUPy, indicating that the emission was contributed mainly by DPA-bisUPy (Fig. 2d). The energy-transfer efficiency (ΦET) of the three SPNPs was 96.8%, 94.4% and 89.3%, respectively. The Förster radius (r0) was estimated to be 3.4 nm for the D–A pair (see ESI† for details). The phosphorescence quantum yield of the Por(Pt)-bisUPy monomer in chloroform is 0.4%, which was enhanced by more than 14-times to 5.7% in an aqueous solution of the SPNPs (D/A = 40
:
1) due to the light harvesting effect from the FRET process.15 The photophysical properties of the monomers and SPNPs were studied and listed in the ESI† in Table S1. The phosphorescence lifetime of the SPNPs-dispersed aqueous solution was found to be 70.3 μs at 660 nm under nitrogen atmosphere, which is a significant enhancement from that of the platinum(II)porphyrin moiety in chloroform (τ = 36.4 μs) and conjugated polymers (34.7 μs).7a This might be attributed to the suppression of the aggregation and free motion of Por(Pt)-bisUPy by the rigid supramolecular backbone. Higher phosphorescence quantum yield and longer phosphorescence lifetime are beneficial for sensitive O2 detection and efficient 1O2 production.
Hypoxia sensing and imaging
The luminescence spectra of SPNPs in water under different O2 partial pressures are presented in Fig. 3a. The D to A ratio of 40
:
1 in SPNPs exhibited appropriate fluorescence to phosphorescence signal ratios. The fluorescence at 434 nm associated with the DPA-bisUPy moiety was almost unchanged, while the phosphorescence at 660 nm from the Por(Pt)-bisUPy moiety decreased markedly with an increase in partial pressure of O2. As a result, the ratio of phosphorescence to fluorescence intensity in the SPNPs is dependent on the O2 concentration. The red phosphorescence at 660 nm became dominant under 0% O2 atmosphere in the presence of efficient FRET from the singlet excited state of DPA-bisUPy to the Por(Pt)-bisUPy moieties. Upon increasing the O2 partial pressure, the emission colors of the aqueous solution changed from pink to purple, which can be visualized under a 365 nm UV lamp by the naked eyes (inset of Fig. 3c). The ratiometric O2 sensing was analyzed quantitatively according to the Stern–Volmer equation based on the data shown in Fig. 3b. | 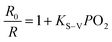 | (1) |
where R and R0 are the ratios of the emission intensities of phosphorescence at 660 nm and fluorescence at 434 nm in the presence and absence of O2, respectively, PO2 is the O2 partial pressure, and KS–V represents the Stern–Volmer constant of the plot involving R0/R and PO2. As shown in Fig. 3b, there is a linear relationship between R0/R and PO2, from which KS–V was estimated to be 7.26 bar−1. The O2 partial pressure in aqueous solution was determined using this KS–V value. The high sensitivity of the SPNPs could be expressed as the Q factor from the total response to O2, which was measured to be 82.6% (see ESI† for details). The phosphorescence quenching by O2 is rapid and reversible by N2 bubbling, revealing the reusability of the system. The phosphorescence quenching process by collision with O2 was further confirmed by measuring the phosphorescence lifetimes at 660 nm, which were 15.7 μs, 32.3 μs and 70.3 μs for the SPNPs-dispersed aqueous solutions under O2, air and N2 atmosphere, respectively (Fig. 3c).
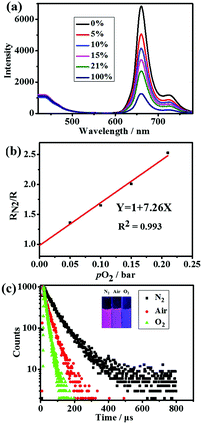 |
| Fig. 3 (a) Ratiometric emission responses of the aqueous solution of SPNPs (D/A = 40 : 1) under different oxygen partial pressures (0%, 5%, 10%, 15%, 21%, and 100% bar) (λex = 400 nm). (b) Stern–Volmer plot of RN2/R vs. different O2 partial pressures. (c) Luminescence decays at 660 nm of the SPNPs in water under oxygen, air and nitrogen atmospheres (λex = 400 nm). The concentration of the SPNPs expressed by DPA-bisUPy is 2.7 × 10−5 M. Inset: Emission colors of the SPNPs-dispersed aqueous solution with different gas atmospheres under a 365 nm UV lamp. | |
Imaging of intracellular O2 levels by SPNPs in HeLa cells was investigated via confocal laser scanning microscopy (CLSM). HeLa cells were cultured with the SPNPs, and then incubated under 1% and 21% O2 atmosphere at 37 °C. Upon excitation at 405 nm, the signals for fluorescence (430–470 nm, Iblue) and phosphorescence (660–740 nm, Ired) were collected in two separate channels. The outstanding ratiometric intracellular O2 detection properties of the SPNPs are evident from the changes in the Iblue/Ired ratio values in HeLa cells cultured under 21% and 1% oxygen atmosphere (Fig. 4).
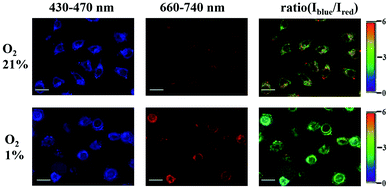 |
| Fig. 4 Ratiometric confocal luminescence images of HeLa cells cultured with SPNPs (1.8 × 10−6 M in water) under 1% and 21% O2 partial pressure. The ratio between the emission intensities at 430–470 nm to that at 660–740 nm (Iblue/Ired) was set as the detection signal (λex = 405 nm), scale bar: 20 μm. | |
Photoinduced cytotoxicity by the PDT process
Next, the electron spin resonance spectra (ESR) were recorded to verify the generation of 1O2. For this purpose, 1O2 was trapped with the well-known scavenger 2,2,6,6-tetramethylpiperidine (TEMP).16 As shown in Fig. 5a, upon irradiation of a mixture of TEMP and SPNPs-dispersed aqueous solution for 5 min, ESR signals of the 1O2 adduct was detected. The quantum yield of 1O2 generation was measured via chemical trapping with 1,3-diphenylbenzofuran (DPBF)17 and Rose Bengal (RB) as the standard photosensitizer (ΦRB = 0.80 in MeOH) under 532 nm laser irradiation.18 As illustrated in Fig. 5b and Fig. S3 (ESI†), the absorption due to the DPBF solution at a wavelength of 418 nm decreased linearly over 60 s of irradiation. This suggests that 1O2 was generated efficiently upon direct excitation of the Q band of the Por(Pt)-bisUPy moiety in the SPNPs. The 1O2 quantum yield was calculated to be 0.70 (Fig. 5c).
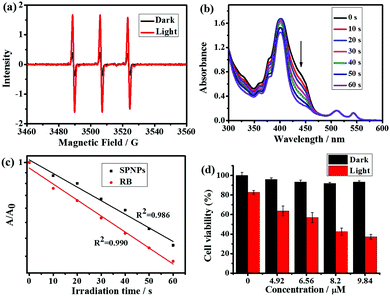 |
| Fig. 5 (a) ESR spectra of an aqueous solution of SPNPs (4.1 × 10−4 M) and TEMP (3 μL) upon photoirradiation by an Xe lamp at >400 nm for 0 min and 5 min under air. (b) UV-vis absorption spectra of SPNPs (1.4 × 10−4 M) and DPBF (40 μL) upon irradiation by a 532 nm laser. (c) Plots of the optical density changes of DPBF at 418 nm in the presence of SPNPs and RB versus irradiation time. (d) CCK-8 cell viabilities of HeLa cells incubated with SPNPs at various concentrations and then irradiated with an Xe lamp (>400 nm, 30 mW) for 30 min. The concentrations of the SPNPs are expressed using DPA-bisUPy, in which [D]/[A] = 40 : 1. | |
To evaluate the PDT performance of the SPNPs quantitatively, the photoinduced cytotoxicity of the SPNPs in HeLa cells was measured using the standard Cell Counting Kit-8 assay (CCK-8) at various concentrations (0–9.84 μM). As shown in Fig. 5d, under the PDT process, prominent cytotoxicity was revealed. Upon increasing the sample concentration to 9.84 μM, the viability of the cells decreased to 37%. However, the SPNPs had a negligible effect on the viability of the cells in the dark, confirming the need for light in the process. The PDT effects of the SPNPs against cancer cells were further studied by monitoring the morphological variations in the HeLa cells with SPNPs under 514 nm laser irradiation using CLSM. As illustrated in Fig. S4 (ESI†), the morphology of the cells changed significantly upon laser irradiation, with the formation of numerous blebs and shrinkage of the HeLa cells, indicating apoptosis and death of the tumour cells, respectively. In sharp contrast, no significant morphological changes in the cancer cells were observed without SPNPs under the same conditions, suggesting that the SPNPs efficiently generated 1O2 upon laser irradiation. Furthermore, the SPNPs exhibited superior storage stability and photostability, which are critical in biomedical applications (Fig. S5 and see ESI† for details).
Conclusions
In summary, we presented our design of a multifunctional SPNPs-based O2 nanoprobe for ratiometric hypoxia sensing, imaging and therapy. The nanoprobe was assembled by a phosphorescent Pt(II) porphyrin unit and fluorescent 9,10-diphenylanthracene moiety through quadruple hydrogen bonding. Bright phosphorescence with long lifetime was facilitated by the efficient energy transfer in the nanoprobe, resulting in sensitive ratiometric hypoxia sensing both in solution and in living cells. More importantly, the singlet oxygen generated during the quenching process also causes damage to cancer cells. The SPNPs possess the advantages of easy preparation and structural modulation, good water-dispersibility, excellent stability, and low dark cytotoxicity toward living cells. The preliminary experiments demonstrate the great potential of SPNP-based integrated systems for diagnosis therapy in the biomedical field. The investigation of SPNPs in PDT for near-infrared multi-photon excitation is currently underway.
Experimental section
Synthesis
DPA-bisUPy was prepared in high yield according to the reported procedure.14a Por(Pt)-bisUPy was synthesized following the steps outlined in Scheme S1 (ESI†).
Synthesis of Por(Pt)-bisUPy: a mixture of P4 (100 mg, 0.089 mmol), UPy-SH (75 mg, 0.235 mmol) and triethylamine (0.1 mL) in CH2Cl2 (50 mL) was stirred under N2 at room temperature for 24 h. After evaporation of the solvent, the product was purified via gel chromatography using CH2Cl2/CH3OH (100
:
1, v/v) as the eluent to afford 0.06 g of the product. Yield: 60%. 1H NMR (400 MHz, CDCl3): δ (ppm) = 0.87–0.91(m, 12H), 1.27(s, 8H), 1.58–1.67(m, 8H), 2.34(s, 2H), 2.94–3.10(m, 12H), 3.60–3.61(m, 4H), 5.90(s, 2H), 7.50–7.52(d, 4 H, J = 6.8 Hz), 8.15–8.17(d, 4H, J = 7.2 Hz), 8.72–8.73(d, 4H, J = 3.6 Hz), 8.88–8.90(d, 4H, J = 4.0 Hz), 10.60(s, 2H), 12.09(s, 2H), 13.21(s, 2H). 13C NMR (100 MHz, CDCl3): δ 11.76, 13.91, 14.16, 22.57, 22.77, 25.66, 26.75, 27.24, 27.34, 29.43, 29.71, 29.80, 29.89, 31.66, 32.02, 32.99, 35.41, 40.07, 45.51, 106.45, 120.31, 122.55, 129.43, 132.62, 134.80, 138.23, 140.59, 141.60, 151.10, 154.99, 155.82, 157.07, 170.56, 173.29. HR-ESI-MS: m/z calculated for [M + H]+ C78H71F10N12O8PtS2: 1752.4424, found 1752.4442.
Preparation of SPNPs
SPNPs with different molar ratios of Por(Pt)-bisUPy and DPA-bisUPy (1
:
30, 1
:
40, 1
:
60) were prepared by following the general method outlined below for a solution of 1
:
40 molar ratio of Por(Pt)-bisUPy and DPA-bisUPy: a mixture of Por(Pt)-bisUPy (0.24 mg, 0.14 mmol) and DPA-bisUPy (4.76 mg, 5.54 mmol) in 200 μL of CHCl3 was quickly injected into 10 mL of CTAB aqueous solution (1.0 × 10−3 M), sonicated for 25 min, centrifuged and washed with water three times to afford uniform nanoparticles.
The concentration of the SPNPs in aqueous solution is expressed by the DPA-bisUPy concentration. Different concentrations of SPNPs in water were prepared by diluting the concentrated stock aqueous solution of SPNPs. Lifetime and quantum yield measurements were performed by carefully degassing all solutions.
Phosphorescence quantum yield measurements
The phosphorescence quantum yield was measured using [Ru(bpy)3]Cl2 (Φ = 0.094 in deoxygenated acetonitrile) as the standard according to the literature procedure.19,20 | 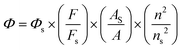 | (2) |
In eqn (2), Φ represents the quantum yield, F denotes the integrated phosphorescence intensity, n represents the refractive index, and A is the optical density. The subscript S stands for the standard.
Measurement of quantum yield for 1O2 generation
RB and DPBF were used as the singlet oxygen photosensitizer and 1O2-trapping agent, respectively. In the experiments, 40 μL DPBF solution (0.75 mg mL−1 in MeOH) was added to 2 mL SPNP-dispersed aqueous solution (1.4 × 10−4 M) and photoirradiated under a 532 nm laser at a power of 40 mW. The reaction decay rate was obtained by recording the absorption of DPBF at 418 nm at different irradiation times. The 1O2 quantum yield was calculated using eqn (3): | 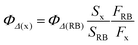 | (3) |
where subscripts x and RB denote the nanoprobe and RB, respectively, and S represents the linear slope of the plot of DPBF absorbance at 418 nm versus irradiation time. F, which is given by F = 1–10−OD, represents the absorption correction factor (OD stands for the optical density of the SPNPs and RB at 532 nm). ΦΔ(RB) is the singlet oxygen quantum yield of RB, and ΦΔ(RB) = 0.80 in MeOH. Photoirradiation of the solutions under identical conditions but in the absence of either photosensitizer or light induced negligible absorption changes.
Cytotoxicity of SPNPs
The dark cytotoxicity and photocytoxicity of the SPNPs on HeLa cells were evaluated using the standard CCK-8 assay. The SPNPs-dispersed aqueous solution was diluted to various concentrations (0, 4.92, 6.56, 8.20 and 9.84 μM) with DMEM medium containing 5% FBS. The HeLa cells were then incubated with the above solutions under 5% CO2 at 37 °C for 4 h. After removing the medium, the HeLa cells were washed with fresh medium twice to remove free SPNPs, and irradiated by a Xe lamp at an intensity of 30 mW for 0 or 30 min. Following this, fresh growth medium (100 μL) containing CCK-8 (10 μL) was added to each well. Upon 20 h incubation, the absorbance intensity at 450 nm was recorded using a microplate reader. The control wells did not include SPNPs. The reference point of 100% cell viability was established by the absorbance of an untreated cell population under identical experimental conditions. The ratio between the average absorption intensities of the sample wells and control wells, [A]sample/[A]control, was calculated to obtain the cell viability relative to the control.
Conflicts of interest
There are no conflicts to declare.
Acknowledgements
Financial support from the Ministry of Science and Technology of China (2014CB239402 and 2017YFA0206903), the National Natural Science Foundation of China (21474124, 21390404 and 91427303), the Strategic Priority Research Program of the Chinese Academy of Sciences (XDB17000000), the Key Research Program of Frontier Sciences of the Chinese Academy of Sciences (QYZDY-SSW-JSC029) are gratefully acknowledged. V. Ramamurthy acknowledges K. C. Wong Education Foundation for the research support.
Notes and references
-
(a) G. L. Semenza, Life with Oxygen, Science, 2007, 318, 62–64 CrossRef PubMed;
(b) J. Aragones, P. Fraisl, M. Baes and P. Carmeliet, Oxygen Sensors at the Crossroad of Metabolism, Cell Metab., 2009, 9, 11–22 CrossRef PubMed.
-
(a) P. Carmeliet, Y. Dor, J. M. Herbert, D. Fukumura, K. Brusselmans, M. Dewerchin, M. Neeman, F. Bono, R. Abramovitch, P. Maxwell, C. J. Koch, P. Ratcliffe, L. Moons, R. K. Jain, D. Collen and E. Keshet, Role of HIF-1α in Hypoxia-Mediated Apoptosis, Cell Proliferation and Tumour Angiogenesis, Nature, 1998, 394, 485–490 CrossRef PubMed;
(b) A. H. V. Schapira, Lancet, 2012, 379, 1825–1834 CrossRef PubMed;
(c) J. E. Selfridge, L. E. J. H. Lu and R. H. Swerdlow, Role of Mitochondrial Homeostasis and Dynamics in Alzheimer's Disease, Neurobiol. Dis., 2013, 51, 3–12 CrossRef PubMed.
-
(a) X.-D. Wang and O. S. Wolfbeis, Optical Methods for Sensing and Imaging Oxygen: Materials, Spectroscopies and Applications, Chem. Soc. Rev., 2014, 43, 3666–3761 RSC;
(b) D. B. Papkovsky and R. I. Dmitriev, Biological Detection by Optical Oxygen Sensing, Chem. Soc. Rev., 2013, 42, 8700–8732 RSC;
(c) Y. Feng, J. Cheng, L. Zhou, X. Zhou and H. Xiang, Ratiometric Optical Oxygen Sensing: a Review in Respect of Material Design, Analyst, 2012, 137, 4885–4901 RSC.
-
(a) Y.-E. L. Koo, Y. Cao, R. Kopelman, S. M. Koo, M. Brasuel and M. A. Philbert, Real-Time Measurements of Dissolved Oxygen Inside Live Cells by Organically Modified Silicate Fluorescent Nanosensors, Anal. Chem., 2004, 76, 2498–2505 CrossRef PubMed;
(b) X.-d. Wang, J. A. Stolwijk, T. Lang, M. Sperber, J. Wegener and O. S. Wolfbeis, Ultra-Small, Highly Stable, and Sensitive Dual Nanosensors for Imaging Intracellular Oxygen and pH in Cytosol, J. Am. Chem. Soc., 2012, 134, 17011–17014 CrossRef PubMed;
(c) J. N. Liu, Y. Liu, W. B. Bu, J. W. Bu, Y. Sun, J. L. Du and J. L. Shi, Ultrasensitive Nanosensors based on Upconversion Nanoparticles for Selective Hypoxia Imaging in Vivo upon Near-Infrared Excitation, J. Am. Chem. Soc., 2014, 136, 9701–9709 CrossRef PubMed.
- C. M. Lemon, P. N. Curtin, R. C. Somers, A. B. Greytak, R. M. Lanning, R. K. Jain, M. G. Bawendi and D. G. Nocera, Metabolic Tumor Profiling with pH, Oxygen, and Glucose Chemosensors on a Quantum Dot Scaffold, Inorg. Chem., 2014, 53, 1900–1915 CrossRef PubMed.
-
(a) R. Xu, Y. Wang, X. Duan, K. Lu, D. Micheroni, A. Hu and W. Lin, Nanoscale Metal-Organic Frameworks for Ratiometric Oxygen Sensing in Live Cells, J. Am. Chem. Soc., 2016, 138, 2158–2161 CrossRef PubMed;
(b) C. F. Wu, B. Bull, K. Christensen and J. McNeill, Ratiometric Single-Nanoparticle Oxygen Sensors for Biological Imaging, Angew. Chem., Int. Ed., 2009, 48, 2741–2745 CrossRef PubMed.
-
(a) X. B. Zhou, H. Liang, P. F. Jiang, K. Y. Zhang, S. J. Liu, T. S. Yang, Q. Zhao, L. J. Yang, W. Lv, Q. Yu and W. Huang, Multifunctional Phosphorescent Conjugated Polymer Dots for Hypoxia Imaging and Photodynamic Therapy of Cancer Cells, Adv. Sci., 2016, 3, 1500155 CrossRef PubMed;
(b) H. Shi, X. Ma, Q. Zhao, B. Liu, Q. Qu, Z. An, Y. Zhao and W. Huang, Ultrasmall Phosphorescent Polymer Dots for Ratiometric
Oxygen Sensing and Photodynamic Cancer Therapy, Adv. Funct. Mater., 2014, 24, 4823–4830 CrossRef;
(c) Z. Lv, L. Zou, H. Wei, S. Liu, W. Huang and Q. Zhao, Phosphorescent Starburst Pt(ii) Porphyrins as Bifunctional Therapeutic Agents for Tumor Hypoxia Imaging and Photodynamic Therapy, ACS Appl. Mater. Interfaces, 2018, 10, 19523–19533 CrossRef PubMed;
(d) Z. Feng, P. Tao, L. Zou, P. Gao, Y. Liu, X. Liu, H. Wang, S. Liu, Q. Dong, J. Li, B. Xu, W. Huang, W.-Y. Wong and Q. Zhao, Hyperbranched Phosphorescent Conjugated Polymer Dots with Iridium(III) Complex as the Core for Hypoxia Imaging and Photodynamic Therapy, ACS Appl. Mater. Interfaces, 2017, 9, 28319–28330 CrossRef PubMed.
-
(a) S. S. Lucky, K. C. Soo and Y. Zhang, Nanoparticles in Photodynamic Therapy, Chem. Rev., 2015, 115, 1990–2042 CrossRef PubMed;
(b) Y. Shen, A. J. Shuhendler, D. Ye, J.-J. Xu and H.-Y. Chen, Two-Photon Excitation Nanoparticles for Photodynamic Therapy, Chem. Soc. Rev., 2016, 45, 6725–6741 RSC;
(c) C. Zhu, L. Liu, Q. Yang, F. Lv and S. Wang, Water-Soluble Conjugated Polymers for Imaging, Diagnosis, and Therapy, Chem. Rev., 2012, 112, 4687–4735 CrossRef PubMed;
(d) L. Chen, H. Bai, Q. Song, J.-F. Xu, S. Wang and X. Zhang, Supramolecular Porphyrin Photosensitizers: Controllable Disguise and Photoinduced Activation of Antibacterial Behavior, ACS Appl. Mater. Interfaces, 2017, 9, 13950–13957 CrossRef PubMed.
-
(a) M. Ethirajan, Y. Chen, P. Joshi and R. K. Pandey, The Role of Porphyrin Chemistry in Tumor Imaging and Photodynamic Therapy, Chem. Soc. Rev., 2011, 40, 340–362 RSC;
(b) S. Singh, A. Aggarwal, N. V. S. D. K. Bhupathiraju, G. Arianna, K. Tiwari and C. M. Drain, Glycosylated Porphyrins, Phthalocyanines, and Other Porphyrinoids for Diagnostics and Therapeutics, Chem. Rev., 2015, 115, 10261–10306 CrossRef PubMed;
(c) J. P. Celli, B. Q. Spring, I. Rizvi, C. L. Evans, K. S. Samkoe, S. Verma, B. W. Pogue and T. Hasan, Imaging and Photodynamic Therapy: Mechanisms, Monitoring, and Optimization, Chem. Rev., 2010, 110, 2795–2838 CrossRef PubMed;
(d) J. Zhao, K. Xu, W. Yang, Z. Wang and F. Zhong, The Triplet Excited State of Bodipy: Formation, Modulation and Application, Chem. Soc. Rev., 2015, 44, 8904–8939 RSC;
(e) A. Kamkaew, S. H. Lim, H. B. Lee, L. V. Kiew, L. Y. Chung and K. Burgess, BODIPY Dyes in Photodynamic Therapy, Chem. Soc. Rev., 2013, 42, 77–88 RSC.
-
(a) R. P. Sijbesma, F. H. Beijer, L. Brunsveld, B. J. B. Folmer, J. H. K. Ky Hirschberg, R. F. M. Lange, J. K. L. Lowe and E. W. Meijer, Reversible Polymers Formed from Self-Complementary Monomers Using Quadruple Hydrogen Bonding, Science, 1997, 278, 1601–1604 CrossRef PubMed;
(b) S.-G. Chen, Y. Yu, X. Zhao, Y. Ma, X.-K. Jiang and Z.-T. Li, Highly Stable Chiral (A)6–B Supramolecular Copolymers: A Multivalency-Based Self-Assembly Process, J. Am. Chem. Soc., 2011, 133, 11124–11127 CrossRef PubMed.
-
(a) L. Yang, X. Tan, Z. Wang and X. Zhang, Supramolecular Polymers: Historical Development, Preparation, Characterization, and Functions, Chem. Rev., 2015, 115, 7196–7239 CrossRef PubMed;
(b) T. Aida, E. W. Meijer and S. I. Stupp, Functional Supramolecular Polymers, Science, 2012, 335, 813–817 CrossRef PubMed;
(c) C. Fouquey, J. M. Lehn and A. M. Levelut, Molecular Recognition Directed Self-assembly of Supramolecular Liquid Crystalline Polymers from Complementary Chiral Components, Adv. Mater., 1990, 2, 254–257 CrossRef.
-
(a) J. D. Barrio, P. N. Horton, D. Lairez, G. O. Lloyd, C. Toprakcioglu and O. A. Scherman, Photocontrol over Cucurbit [8] uril Complexes: Stoichiometry and Supramolecular Polymers, J. Am. Chem. Soc., 2013, 135, 11760–11763 CrossRef PubMed;
(b) Y. Wang, C.-L. Sun, L.-Y. Niu, L.-Z. Wu, C.-H. Tung, Y.-Z. Chen and Q.-Z. Yang, Photoresponsive AA/BB Supramolecular polymers Comprising Stiff-stilbene Based Guests and Bispillar [5] arenes, Polym. Chem., 2017, 8, 3596–3602 RSC.
-
(a) G. Yu, X. Zhao, J. Zhou, Z. Mao, X. Huang, Z. Wang, B. Hua, Y. Liu, F. Zhang, Z. He, O. Jacobson, C. Gao, W. Wang, C. Yu, X. Zhu, F. Huang and X. Chen, Supramolecular Polymer-Based Nanomedicine: High Therapeutic Performance and Negligible Long-Term Immunotoxicity, J. Am. Chem. Soc., 2018, 140, 8005–8019 CrossRef PubMed;
(b) M. Ni, N. Zhang, W. Xia, X. Wu, C. Yao, X. Liu, X.-Y. Hu, C. Lin and L. Wang, Dramatically Promoted Swelling of a Hydrogel by Pillar[6]arene–Ferrocene Complexation with Multistimuli Responsiveness, J. Am. Chem. Soc., 2016, 138, 6643–6649 CrossRef PubMed.
-
(a) H.-Q. Peng, J.-F. Xu, Y.-Z. Chen, L.-Z. Wu, C.-H. Tung and Q.-Z. Yang, Water-Dispersible Nanospheres of Hydrogen-Bonded Supramolecular Polymers and their application for mimicking light-harvesting systems, Chem. Commun., 2014, 50, 1334–1337 RSC;
(b) H.-Q. Peng, C.-L. Sun, L.-Y. Niu, Y.-Z. Chen, L.-Z. Wu, C.-H. Tung and Q.-Z. Yang, Supramolecular Polymeric Fluorescent Nanoparticles based on Quadruple Hydrogen Bonds, Adv. Funct. Mater., 2016, 26, 5483–5489 CrossRef;
(c) R.-F. Wang, H.-Q. Peng, P.-Z. Chen, L.-Y. Niu, J.-F. Gao, L.-Z. Wu, C.-H. Tung, Y.-Z. Chen and Q.-Z. Yang, A Hydrogen-Bonded-Supramolecular-Polymer-Based Nanoprobe for Ratiometric Oxygen Sensing in Living Cells, Adv. Funct. Mater., 2016, 26, 5419–5425 CrossRef;
(d) F.-W. Liu, L.-Y. Niu, Y. Chen, V. Ramamurthy, L.-Z. Wu, C.-H. Tung, Y.-Z. Chen and Q.-Z. Yang, A Phosphorescent Platinum(II) Bipyridyl Supramolecular Polymer Based on Quadruple Hydrogen Bonds, Chem. – Eur. J., 2016, 22, 18132–18139 CrossRef PubMed.
- H.-Q. Peng, Y.-Z. Chen, Y. Zhao, Q.-Z. Yang, L.-Z. Wu, C.-H. Tung, L.-P. Zhang and Q.-X. Tong, Artificial Light-Harvesting System Based on Multifunctional Surface-Cross-Linked Micelles, Angew. Chem., Int. Ed., 2012, 51, 2088–2092 CrossRef PubMed.
- J. Ge, M. Lan, B. Zhou, W. Liu, L. Guo, H. Wang, Q. Jia, G. Niu, X. Huang, H. Zhou, X. Meng, P. Wang, C.-S. Lee, W. Zhang, X. Han and A. Graphene, Quantum Dot Photodynamic Therapy Agent with High Singlet Oxygen Generation, Nat. Commun., 2014, 5, 4596 CrossRef PubMed.
- N. Adarsh, R. R. Avirah and D. Ramaiah, Tuning Photosensitized Singlet Oxygen Generation Efficiency of Novel Aza-BODIPY Dyes, Org. Lett., 2010, 12, 5720–5723 CrossRef PubMed.
- L. Xiao, L. Gu, S. B. Howell and M. J. Sailor, Porous Silicon Nanoparticle Photosensitizers for Singlet Oxygen and Their Phototoxicity against Cancer Cells, ACS Nano, 2011, 5, 3651–3659 CrossRef PubMed.
- A. M. Brouwer, Standards for Photoluminescence Quantum Yield Measurements in Solution (IUPAC Technical Report), Pure Appl. Chem., 2011, 83, 2213–2228 Search PubMed.
- K. Suzuki, A. Kobayashi, S. Kaneko, K. Takehira, T. Yoshihara, H. Ishida, Y. Shiina, S. Oishic and S. Tobita, Reevaluation of Absolute Luminescence Quantum Yields of Standard Solutions Using a Spectrometer with an Integrating Sphere and a Back-Thinned CCD Detector, Phys. Chem. Chem. Phys., 2009, 11, 9850–9860 RSC.
Footnote |
† Electronic supplementary information (ESI) available: Synthesis and characterization of compounds, photophysical parameters, energy transfer calculations and other materials. See DOI: 10.1039/c8qm00309b |
|
This journal is © the Partner Organisations 2018 |