DOI:
10.1039/C8QM00181B
(Research Article)
Mater. Chem. Front., 2018,
2, 1449-1455
Control of intramolecular excimer emission in luminophore-integrated ionic POSSs possessing flexible side-chains†
Received
23rd April 2018
, Accepted 22nd May 2018
First published on 5th June 2018
Abstract
Polycyclic aromatic hydrocarbons such as naphthalene, anthracene and pyrene were integrated onto polyhedral oligomeric silsesquioxanes (POSSs) having flexible ionic linkers and their luminescence properties were evaluated. Initially, significant excimer emissions were observed from the diluted solutions including each modified POSS. It was found that excimer formation around the POSS unit was facilitated by connecting to the rigid core via the flexible linkers. In particular, the luminescence properties were drastically changed depending on the solvent polarity. It was indicated that the spatial distances between the side-chains can be regulated by introducing ionic groups into the side-chains. Furthermore, the luminescence properties in aqueous solution of the modified POSSs were controllable by adding amphiphilic anions and polyanionic compounds. Finally, this system was applied for the detection of sodium dodecyl sulfate (SDS) which is the conventional indicator of river pollution. These data indicate that intramolecular interaction between the side-chains around the POSS was dynamically controlled by environmental factors. Consequently, stimuli-responsive luminescent materials were obtained.
Introduction
Organic–inorganic hybrids, which are a class of mixtures with organic and inorganic components at the molecular level, have been utilized as a scaffold for constructing emissive materials by introducing luminescent dyes.1 Owing to superior properties such as transparency, homogeneity, thermal and photostability, toughness, and environmental resistance, emissive hybrids have been paid attention as a key unit in modern optoelectronic devices. Therefore, many efforts have been devoted to exploring the unique photochemical characteristics of the loaded luminescent dyes and the application of their optical properties for developing advanced optoelectronic devices.2–4 Polyhedral oligomeric silsesquioxane (POSS) represents a class of molecules which have a cubic silica core and eight organic groups at each vertex and has been applied for fabricating functional materials.5–17 Simply by introducing luminescent units at each vertex, versatile optical functions applicable to light-emitting diodes, sensors and solid-state luminescent materials were obtained.18–22 Originating from the rigidity of the silica core, it was shown that various types of POSS derivatives were responsible for the enhancement of the thermal and mechanical properties of conventional polymers simply by mixing into the film samples.23–28 Thereby, POSS has been focused on for obtaining an effective molecular filler to improve the durability of polymeric materials. By employing the thermally-stabilizing effect of POSS, solid-state luminescent materials with high robustness were obtained.29 Thus, because of the structural features and physical properties, POSS can be regarded as a versatile element-block, which is a minimum functional unit composed of inorganic elements, for constructing organic–inorganic hybrids.30,31
Conventional hybrid materials show slight environment-responsive property changes due to high robustness as mentioned above. Meanwhile, control of physical properties is possible by employing POSS. Various researchers reported nanostructured assemblies of POSS.32–35 Polymer-modified POSS showed diverse assembly structures depending on environmental factors such as solvents and temperature. Naka et al. presented that the hydrophobic POSS unit crucially influences the morphology in micelle formation.36 Based on the strategy of capturing guests in the sparse space around POSS, a chemical sensor can be obtained.37 We have also reported regulation of the encapsulation ability of the POSS-based polymer–hydrophobic dye complexes.38 Depended on the pH, the amount of encapsulated molecules inside the dendrimers was altered. In these studies, intermolecular interaction among POSS derivatives played a critical role in the formation of nanostructures and physical properties. In contrast, to the best of our knowledge, few examples have been presented to control an intramolecular interaction between the side-chains inside a single POSS molecule and especially to utilize molecular interaction positively for regulating the electronic properties of the tethered units. Thus, our next interest is not only to induce intramolecular interaction between the luminophores inside a single molecule according to a preprogrammed design but also to demonstrate dynamic controls of the optical properties originating from the degree of intramolecular interaction around the POSS core.
Herein, we synthesized modified POSS with polycyclic aromatic hydrocarbons such as naphthalene, anthracene and pyrene and their optical properties were investigated. To allow the luminophores to approach neighboring molecules, the luminophores were tethered with flexible and short spacers. Additionally, the quaternary ammonium groups were introduced into the side-chains for modulating the molecular conformation by electrostatic interaction with solvents and additional ionic species. From the optical measurements, excimer emissions were initially observed from the diluted solutions including each modified POSS. Moreover, these luminescence properties were able to be altered by changing the solvent polarity and coexisting anions. Finally, the detection of sodium dodecyl sulfate (SDS) which is the conventional indicator of river pollution was demonstrated. We discuss the newly-discovered roles of the special space created around POSS.
Results and discussion
Synthesis
The introduction of functional units into POSS was often suppressed critically due to steric hindrance of the cubic core. By employing the Menshutkin reaction, various compounds can be readily connected.39–41 In this study, POSS derivatives possessing eight luminophores were synthesized via this reaction to introduce ionic groups into all side-chains.41 Several POSSs modified with polycyclic aromatic rings were already reported, whereas there are also a few cases where intramolecular excimer emission was observed.29,32,41–44 Even in the presence of eight pyrenes around POSS, excimer emission was hardly detected in the solution state from the modified POSS through the rigid linker.44 Considering these reports, a much more flexible linker was designed for realizing intramolecular interaction between side-chains. N,N-Dimethylaminopropyl groups were selected as the side-chains of POSS because of their flexibility and relatively short length. POSS having dimethylaminopropyl groups (DMA-POSS) was synthesized by hydrolysis, followed by the condensation reaction of trimethoxysilane using hydrochloric acid (Scheme 1). From the results of 29Si NMR and high-resolution mass measurements, it was confirmed that a T8 structure of POSS was successfully obtained with high selectivity.45 This means that reformation of the cubic cage hardly proceeded during the reactions. As shown in Scheme 1, POSS derivatives (NPOSS, APOSS, PPOSS) having naphthalene, anthracene or pyrene as luminophores were prepared by the Menshutkin reaction, respectively. 1H and 29Si NMR spectra of PPOSS are shown in Fig. 1, indicating that 8-substitution modification was achieved. For comparison of the optical properties, model compounds possessing a trimethylammonium group (NTMA, ATMA, and PTMA) were also synthesized by the same method as the POSS compounds. We also evaluated the thermal stability of the modified POSS with thermogravimetric analyses (Fig. S9 and Table S1, ESI†). Apparently, connection of POSS contributed to improving the thermal stability of the molecules. It is likely that molecular motions would be disturbed by POSS.17,23–31 As a consequence, pyrolysis was suppressed.
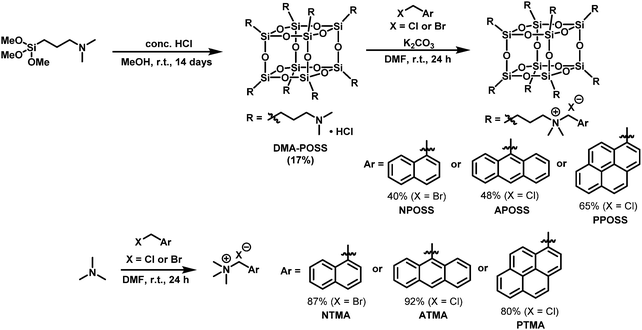 |
| Scheme 1 Synthesis of luminophore-integrated POSS and model compounds. | |
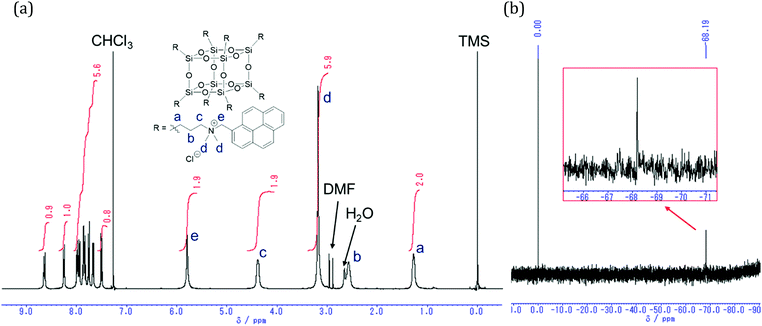 |
| Fig. 1 (a) 1H and (b) 29Si NMR spectra of PPOSS in CDCl3. | |
Optical properties
Optical properties of the synthesized POSS derivatives (NPOSS, APOSS, PPOSS) and model compounds (NTMA, ATMA, PTMA) were measured in dilute chloroform solutions. The UV-vis absorption and PL spectra are shown in Fig. 2. In the UV-vis absorption spectra, significant changes were hardly observed in the series of POSS and model compounds in each luminophore. On the other hand, in the PL spectra, the different behavior between POSS and the model compounds was observed. The model compounds showed vibrating monomer emission from a single luminophore. Meanwhile, broad emission bands in the longer wavelength region were observed from the POSS derivatives than from the monomers. From the results of fluorescence lifetime measurements, it was found that the lifetimes monitored at broad emission bands (λPL,ex) were longer than those monitored at the monomer emission bands (λPL,mo) (Table 1). These data mean that the broad emission band was attributed to excimer emission. Additionally, from the spectra under the much diluted conditions (1.0 × 10−6 M) and the comparison with model compounds in the absorption spectra, similar data were obtained, indicating that POSS derivatives should be homogeneously dispersed (Fig. S1 and S2, ESI†). Thereby, it was suggested that the excimer emission should be originated from the intramolecular excimer between the side-chains in the single POSS molecule. Naphthalene, anthracene and pyrene are known to exhibit excimer emission when the intermolecular distances are close to each other.46–48 It should be mentioned that excimer emission was observed even in naphthalene and anthracene which exhibit excimer emission under limited conditions such as assembly in the micelle.49 Moreover, the synthesis and optical properties of the anthracene-modified POSS were previously reported.29 In this molecule, the length at the spacer moiety was longer by 3 carbons than those of our linker, and significant excimer emission was hardly observed. Therefore, it can be said that the compact and three-dimensional structure of POSS with the shorter length of the spacer which was prepared through the Menshutkin reaction could play a positive role in intramolecular excimer formation even with relatively-poor assembling molecules. To evaluate intermolecular interaction on the formation of pyrene excimer, we investigated the concentration dependency of the emission with PPOSS and PTMA (Fig. S10, ESI†). In summary, PTMA hardly showed excimer emission even under concentrated conditions (10−3 M). Meanwhile, excimer emission was detected even under diluted conditions with PPOSS. These results clearly indicated that POSS can play a significant role in the facilitation of excimer formation via intramolecular interaction. In the following experiments, we mainly performed the experiments with 10−6 M concentration in which intermolecular interaction is negligible.
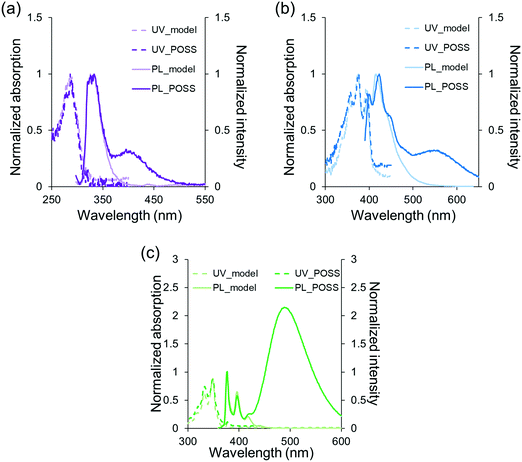 |
| Fig. 2 UV-vis absorption and PL spectra of (a) NPOSS and NTMA, (b) APOSS and ATMA, and (c) PPOSS and PTMA in CHCl3 solution (1.0 × 10−6 M for POSS, 1.0 × 10−5 M for models) excited at the wavelengths of the absorption maxima. | |
Table 1 Optical properties of luminophore-integrated POSS and the model compounds in CHCl3 (1.0 × 10−6 M for POSSs, 1.0 × 10−5 M for models)
|
λ
abs,max (nm) |
ε (× 104 M−1 cm−1) |
λ
PL,mo (nm) |
λ
PL,ex (nm) |
Φ
PL
|
τ
(ns) |
χ
2
|
Absolute PL quantum efficiency excited at λabs,max.
Emission lifetime monitored at λPL,ex for POSS, and λPL,mo for model compounds. Percentages in parentheses represent fractional contribution to the total emission intensity defined as αi/∑αi × 100.
|
NTMA
|
287 |
0.74 |
326 |
— |
0.001 |
0.7 (90%), 3.6 (10%) |
1.10 |
NPOSS
|
288 |
3.59 |
333 |
395 |
0.006 |
7.6 (100%) |
1.04 |
ATMA
|
374 |
0.66 |
415 |
— |
0.056 |
1.2 (27%), 6.2 (73%) |
1.10 |
APOSS
|
377 |
6.30 |
423 |
553 |
0.056 |
28.7 (100%) |
1.03 |
PTMA
|
349 |
3.18 |
376 |
— |
0.131 |
3.9 (6%), 10.5 (94%) |
1.19 |
PPOSS
|
349 |
16.1 |
376 |
489 |
0.185 |
21.8 (100%) |
1.09 |
Solvent effects
Next, to evaluate the influence of the solvent on excimer emission, optical measurements were carried out in various solvents using PPOSS which showed the highest luminescence intensity in the above experiments. Pyrene and its derivatives usually presented excimer emission in polar solvents such as methanol and water.50 Contrary to these conventional behaviors for the solvent effect on excimer emission, it was found that the luminescence intensity of the excimer increased in low polar solvents (Fig. 3). In chloroform, the emission intensity was maximized, while only the monomer luminescence was exhibited in the aqueous solution and even under high salt conditions (Fig. S4, ESI†). It was presumed that this reverse solvatochromicity should be caused by the effect of electrostatic repulsion between the quaternary ammonium groups in the side-chains. In less polar solvents, the counter anion should be present in the vicinity of the quaternary ammonium group. Therefore, the electrostatic repulsion could be relatively weak and the excimer formation was facilitated. On the other hand, especially in water, the counter anion should dissociate, followed by electrostatic repulsion between the cations. As a result, the formation of the intramolecular excimer should be suppressed. In other polar solvents, a similar effect should occur. Thereby, weaker excimer emission was observed.
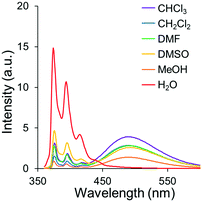 |
| Fig. 3 PL spectra of PPOSS in various solvents (1.0 × 10−6 M, including 1 v/v% of DMSO) excited at 349 nm. | |
Effect of counter anions
To evaluate the effects of counter anions, a chloride anion of PPOSS was exchanged into bis(trifluoromethanesulfonyl)imide (TFSI) as a less polar anion (Scheme 2). From the 1H NMR spectra (Fig. 4), quantitative high field shifts of alkyl and methylene peaks were observed, indicating that the eight counter anions were fully exchanged into TFSI anions. The luminescent properties were evaluated in various solvents (Fig. 5 and Fig. S3, ESI†). Accordingly, in the chloroform and aqueous solutions, the differences in PL spectra between chloride and TFSI anions were hardly obtained; meanwhile, significant changes were observed in the methanol solution (Fig. 5). The intensity of the emission band from the monomer dramatically increased, while that from the excimer decreased in the methanol solution by changing the chloride anions into the TFSI anions. It is likely that the counter anions frequently dissociate in polar solvents. Subsequently, the pyrene units should be separated from each other by electrostatic repulsion at the linker moieties. It is generally known that the negative charge in the TFSI anion is delocalized in a wider range than that in the chloride anion. Therefore, TFSI anions could be loosely captured by the ammonium cation. Thus, it is assumed that electrostatic repulsion might be recovered via anion dissociation in the TFSI salt in methanol. Consequently, a similar spectrum to that in water was obtained.
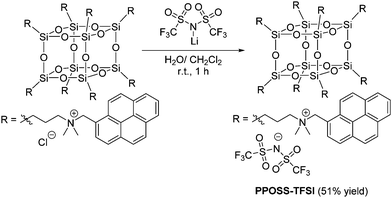 |
| Scheme 2 Synthesis of PPOSS-TFSI. | |
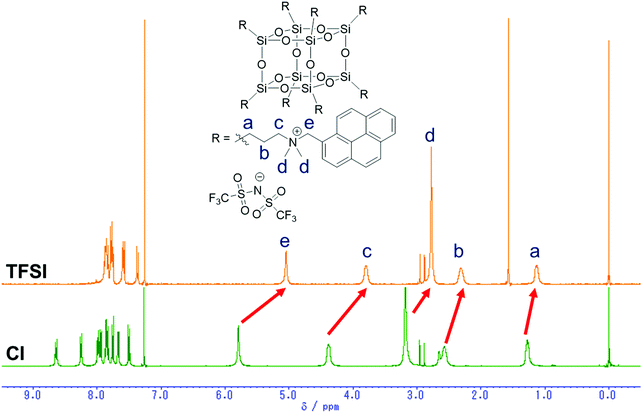 |
| Fig. 4
1H NMR spectra of PPOSS-TFSI and PPOSS in CDCl3. | |
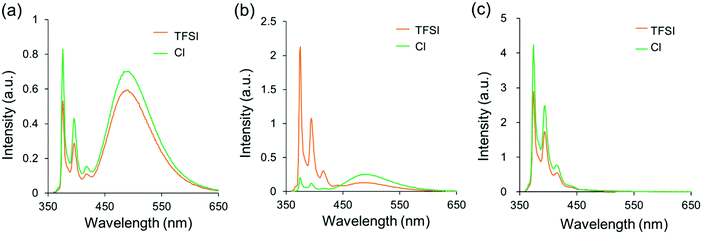 |
| Fig. 5 PL spectra of PPOSS and PPOSS-TFSI in (a) CHCl3, (b) MeOH, and (c) H2O (1.0 × 10−6 M, including 1 v/v% of DMSO) excited at 349 nm. | |
Application as a fluorescence sensor for a pollutant
Finally, we attempted to apply this system for demonstrating a fluorescence sensor based on environment-sensitive excimer emission. From the above experiments, it was suggested that the intensity of the excimer emission was significantly enhanced under relatively-low polar conditions. In the previous reports, it was revealed that less polarity of the POSS core32–35 can be responsible for capturing hydrophobic organic species by hydrophobic interaction.51–60 On the basis of these data, we presumed that hydrophobic species can be recruited in the vicinity of the POSS core and converted to ionic moieties at the linker. As a result, excimer formation could be induced even in aqueous solution. Finally, an ON-type fluorescence sensor for the hydrophobic species can be obtained. To evaluate the validity of this idea, the PL properties of PPOSS were measured in the presence of various alkyl salts in aqueous solutions. Fig. 6 shows the intensity ratios of the excimer to monomer emission (Iexcimer/Imonomer) in each sample. When an amphiphilic molecule such as SDS or LiPFOS was added to the aqueous solution of PPOSS, strong excimer emission was exhibited. Even when polyanionic compounds such as PSSNa or PANa were added, the emission band from the excimer was observed. According to the peak broadening in the UV-vis absorption spectra of the samples, aggregation should occur via the hydrophobic interactions and poly-ion complexation (Fig. 7). Thereby, excimer formation was facilitated. By changing the SDS concentration, the detection limit was estimated (Fig. S5 and S6, ESI†). Significant excimer emission was detectable above 10−4 M and up to the critical micelle concentration (8.2 × 10−3 M). Additionally, weak emission bands were detected in the presence of the SDS analogs and carboxylate (Fig. S7 and S8, ESI†). These results indicate that luminophore-integrated POSSs are useful as fluorescence sensors with high selectivity for detecting anionic surfactants, especially SDS and LiPFOS, which have been of concern regarding their environmental impact in recent years.61
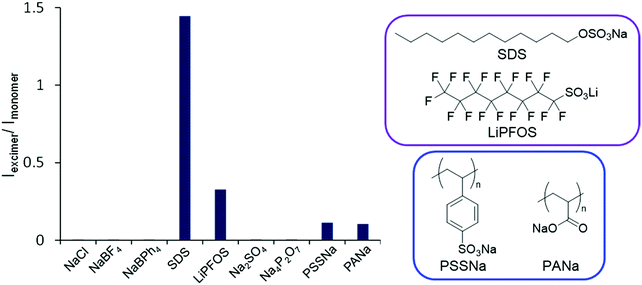 |
| Fig. 6 Intensity of the excimer to monomer emission of PPOSS aqueous solution (1.0 × 10−6 M) with various salts (1.0 × 10−4 M) excited at 349 nm. | |
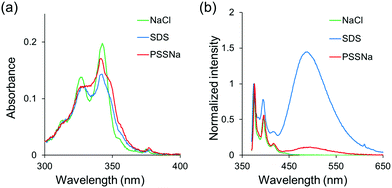 |
| Fig. 7 (a) UV-vis absorption and (b) PL spectra of PPOSS in aqueous solution (1.0 × 10−6 M) with various salts (1.0 × 10−4 M) excited at 349 nm. | |
Conclusions
In this paper, three significant issues are involved: first, the facile and versatile synthesis of the modified POSS having various types of functional units was established by employing the Menschutkin reaction. By selecting the alkyl halide, the desired modification can be readily accomplished. Second, contrary to the conventional polycyclic aromatic hydrocarbon derivatives, it was demonstrated that POSS can facilitate excimer formation in the non-polar solvents in which usually hydrocarbons show homogeneous dispersion states. It should be noted that excimer emission was able to be induced even with polycyclic aromatic hydrocarbons including naphthalene and anthracene, which are generally capable of forming excimers under limited conditions. The rigid and compact structure of POSS could play a critical role in converting the side-chains in the excited state and subsequently inducing excimer emission. Third, dynamic control of intramolecular interaction between side-chains in a single POSS molecule was achieved. By using the resulting ionic moiety at the linker as a key unit for presenting environment sensitivity, emission behaviors were modulated by changing the solvent polarity and counter anions. On the basis of the stimuli-responsiveness, the ON-type sensor for SDS, which can be an indicator for assessing environmental contamination was obtained. Further applications, such as for fabricating bioprobes and environment sensors, are promised to be feasible based on our materials.
Conflicts of interest
There are no conflicts to declare.
Acknowledgements
This work was partially supported by the Training Program of Leaders for Integrated Medical System for Fruitful Healthy-Longevity Society (LIMS), the Program for Leading Graduate Schools, MEXT, Japan (for H. N.), the Takahashi Industrial and Economic Research Foundation (for K. T.) and a Grant-in-Aid for Scientific Research on (A) and Innovative Areas “New Polymeric Materials Based on Element-Blocks (No. 2401)” (JSPS KAKENHI Grant No. 17H01220, JP24102013).
References
- M. Gon, K. Tanaka and Y. Chujo, Bull. Chem. Soc. Jpn., 2017, 90, 463–474 CrossRef.
- Y. Kajiwara, A. Nagai, K. Tanaka and Y. Chujo, J. Mater. Chem. C, 2013, 1, 4437–4444 RSC.
- H. Okada, K. Tanaka and Y. Chujo, Bioorg. Med. Chem., 2014, 22, 3141–3145 CrossRef PubMed.
- H. Okada, K. Tanaka, W. Ohashi and Y. Chujo, Bioorg. Med. Chem., 2014, 22, 3435–3440 CrossRef PubMed.
- K. Tanaka and Y. Chujo, J. Mater. Chem., 2012, 22, 1733–1746 RSC.
- W. Zhang and A. H. E. Müller, Prog. Polym. Sci., 2013, 38, 1121–1162 CrossRef.
- D. B. Cordes, P. D. Lickiss and F. Rataboul, Chem. Rev., 2010, 110, 2081–2173 CrossRef PubMed.
- J. C. Furgal, J. H. Jung, T. GoodsonIII and R. M. Laine, J. Am. Chem. Soc., 2013, 135, 12259–12269 CrossRef PubMed.
- J. C. Furgal, J. H. Jung, S. Clark, T. GoodsonIII and R. M. Laine, Macromolecules, 2013, 46, 7591–7604 CrossRef.
- T. Maegawa, O. Miyashita, Y. Irie, H. Imoto and K. Naka, RSC Adv., 2016, 6, 8346–8353 RSC.
- S. Kinoshita, S. Watase, K. Matsukawa and Y. Kaneko, J. Am. Chem. Soc., 2015, 137, 5061–5065 CrossRef PubMed.
- N. Sato, Y. Kuroda, T. Abe, H. Wada, A. Shimojima and K. Kuroda, Chem. Commun., 2015, 51, 11034–11037 RSC.
- L. Wang, Y. Ishida, R. Maeda, M. Tokita, S. Horiuchi and T. Hayakawa, Langmuir, 2014, 30, 9797–9803 CrossRef PubMed.
- C.-W. Chiou, Y.-C. Lin, L. Wang, C. Hirano, Y. Suzuki, T. Hayakawa and S.-W. Kuo, Polymers, 2014, 6, 926–948 CrossRef.
- N. Oguri, Y. Egawa, N. Takeda and M. Unno, Angew. Chem., Int. Ed., 2016, 55, 9336–9339 CrossRef PubMed.
- R. Gunawidjaja, F. Huang, M. Gumenna, N. Klimenko, G. A. Nunnery, V. Shevchenko, R. Tannenbaum and V. V. Tsukruk, Langmuir, 2009, 25, 1196–1209 CrossRef PubMed.
- P. A. Ledin, W. Xu, F. Friscourt, G.-J. Boons and V. V. Tsukruk, Langmuir, 2015, 31, 8146–8155 CrossRef PubMed.
- M. Lo, C. Zhen, M. Lauters, G. E. Jabbour and A. Sellinger, J. Am. Chem. Soc., 2007, 129, 5808–5809 CrossRef PubMed.
- K. Suenaga, K. Tanaka and Y. Chujo, Chem. – Eur. J., 2017, 23, 1409–1414 CrossRef PubMed.
- G. Zhao, Y. Zhu, S. Guang, F. Ke and H. Xu, New J. Chem., 2017, 42, 555–563 RSC.
- H. Zhou, Q. Ye, X. Wu, J. Song, C. Cho, Y. Zong, B. Tang, T. Hor, E. Yeow and J. Xu, J. Mater. Chem. C, 2015, 3, 11874–11880 RSC.
- C. He, Y. Xiao, J. Huang, T. Lin, K. Y. Mya and X. Zhang, J. Am. Chem. Soc., 2004, 126, 7792–7793 CrossRef PubMed.
- K. Tanaka, H. Kozuka, K. Ueda, J.-H. Jeon and Y. Chujo, Mater. Lett., 2017, 203, 62–67 CrossRef.
- K. Ueda, K. Tanaka and Y. Chujo, Polym. J., 2016, 48, 1133–1139 CrossRef.
- K. Tanaka, H. Yamane, K. Mitamura, S. Watase, K. Matsukawa and Y. Chujo, J. Polym. Sci., Part A: Polym. Chem., 2014, 52, 2588–2595 CrossRef.
- J.-H. Jeon, K. Tanaka and Y. Chujo, J. Mater. Chem. A, 2014, 2, 624–630 Search PubMed.
- J.-H. Jeon, K. Tanaka and Y. Chujo, J. Polym. Sci., Part A: Polym. Chem., 2013, 51, 3583–3589 CrossRef.
- J.-H. Jeon, K. Tanaka and Y. Chujo, RSC Adv., 2013, 3, 2422–2427 RSC.
- M. Gon, K. Sato, K. Tanaka and Y. Chujo, RSC Adv., 2016, 6, 78652–78660 RSC.
- Y. Chujo and K. Tanaka, Bull. Chem. Soc. Jpn., 2015, 88, 633–643 CrossRef.
- M. Gon, K. Tanaka and Y. Chujo, Polym. J., 2018, 50, 109–126 CrossRef.
- N. C. Escudé and E. Y.-X. Chen, Chem. Mater., 2009, 21, 5743–5753 CrossRef.
- W.-B. Zhang, Y. Li, X. Li, X. Dong, X. Yu, C.-L. Wang, C. Wesdemiotis, R. P. Quirk and S. Z. D. Cheng, Macromolecules, 2011, 44, 2589–2596 CrossRef.
- L. Ma, H. Geng, J. Song, J. Li, G. Chen and Q. Li, J. Phys. Chem. B, 2011, 8, 10586–10591 CrossRef PubMed.
- X. Yu, S. Zhong, X. Li, Y. Tu, S. Yang, R. M. V. Horn, C. Ni, D. J. Pochan, R. P. Quirk, C. Wesdemiotis, E.-B. Zhang and S. Z. D. Cheng, J. Am. Chem. Soc., 2010, 132, 16741–16744 CrossRef PubMed.
- S. Yusa, S. Ohno, T. Honda, H. Imoto, Y. Nakao, K. Naka, Y. Nakamura and S. Fujii, RSC Adv., 2016, 6, 73006–73012 RSC.
- K. Xiang, Y. Li, C. Xu and S. Li, J. Mater. Chem. C, 2016, 4, 5578–5583 RSC.
- K. Tanaka, K. Inafuku and Y. Chujo, Chem. Commun., 2010, 46, 4378–4380 RSC.
- P. A. Ledin, I. M. Tkachenko, W. Xu, I. Choi, V. V. Shvchenko and V. V. Tsukruk, Langmuir, 2014, 30, 8856–8865 CrossRef PubMed.
- I. M. Tkachenko, Y. L. Kbzar, V. F. Korolovych, A. V. Stryutsky, L. K. Matkovska, V. V. Shevchenko and V. V. Tsukruk, J. Mater. Chem. C, 2018, 6, 4065–4076 RSC.
- J. Han, Y. Zheng, S. Zheng, S. Li, T. Hu, A. Tang and C. Gao, Chem. Commun., 2014, 50, 8712–8714 RSC.
- Y.-L. Chu, C.-C. Cheng, Y.-P. Chen, Y.-C. Yen and F.-C. Chang, J. Mater. Chem., 2012, 22, 9285–9292 RSC.
- Y. Gao, W. Xu, D. Zhu, L. Chen, Y. Fu, Q. He, H. Cao and J. Cheng, J. Mater. Chem. A, 2014, 3, 4820–4826 Search PubMed.
- S. Chanmungkalakul, V. Ervithayasuporn, S. Hanprasit, M. Masik, N. Prigyai and S. Kiatkamjornwong, Chem. Commun., 2017, 53, 12108–12111 RSC.
- K. Ueda, K. Tanaka and Y. Chujo, Bull. Chem. Soc. Jpn., 2017, 90, 205–209 CrossRef.
- M. Albelda, E. García-España, L. Gil, J. Lima, C. Lodeiro, J. Melo, M. Melo, A. Parola, F. Pina and C. Soriano, J. Phys. Chem. B, 2003, 107, 6573–6578 CrossRef.
- L. Kaanumalle, C. Gibb, B. Gibb and V. Ramamurthy, J. Am. Chem. Soc., 2005, 127, 3674–3675 CrossRef PubMed.
- Z. Xu, N. Singh, J. Lim, J. Pan, H. Kim, S. Park, K. Kim and J. Yoon, J. Am. Chem. Soc., 2009, 131, 15528–15533 CrossRef PubMed.
- R. Akatsuka, A. Momotake, Y. Shinohara, Y. Kanna, T. Sato, M. Moriyama, K. Takahashi, Y. Nishimura and T. Arai, J. Photochem. Photobiol., A, 2011, 223, 1–5 CrossRef.
- K. Zhao, T. Liu, G. Wang, X. Chang, D. Xue, K. Belfield and Y. Fang, J. Phys. Chem. B, 2013, 117, 5659–5667 CrossRef PubMed.
- K. Tanaka, K. Inafuku, K. Naka and Y. Chujo, Org. Biomol. Chem., 2008, 6, 3899–3901 Search PubMed.
- H. Narikiyo, T. Kakuta, H. Matsuyama, M. Gon, K. Tanaka and Y. Chujo, Bioorg. Med. Chem., 2017, 25, 3431–3436 CrossRef PubMed.
- T. Kakuta, J.-H. Jeon, H. Narikiyo, K. Tanaka and Y. Chujo, Bioorg. Med. Chem., 2017, 25, 1389–1393 CrossRef PubMed.
- T. Kakuta, K. Tanaka and Y. Chujo, J. Mater. Chem. C, 2015, 3, 12539–12545 RSC.
- J.-H. Jeon, T. Kakuta, K. Tanaka and Y. Chujo, Bioorg. Med. Chem. Lett., 2015, 25, 2050–2055 CrossRef PubMed.
- J.-H. Jeon, K. Tanaka and Y. Chujo, Org. Biomol. Chem., 2014, 12, 6500–6506 Search PubMed.
- K. Tanaka, H. Okada, W. Ohashi, J.-H. Jeon, K. Inafuku and Y. Chujo, Bioorg. Med. Chem., 2013, 21, 2678–2681 CrossRef PubMed.
- K. Tanaka, J.-H. Jeon, K. Inafuku and Y. Chujo, Bioorg. Med. Chem., 2012, 20, 915–919 CrossRef PubMed.
- K. Tanaka, M. Murakami, J.-H. Jeon and Y. Chujo, Org. Biomol. Chem., 2012, 10, 90–95 Search PubMed.
- K. Tanaka and Y. Chujo, Polym. J., 2013, 45, 247–254 CrossRef.
- N. Sakač, M. Karnaš, M. Jozanović, M. Medvidović-Kosanović, S. Martinez, J. Macan and M. Sak-Bosnar, Anal. Methods, 2017, 9, 2305–2314 RSC.
Footnote |
† Electronic supplementary information (ESI) available. See DOI: 10.1039/c8qm00181b |
|
This journal is © the Partner Organisations 2018 |
Click here to see how this site uses Cookies. View our privacy policy here.