DOI:
10.1039/C8QM00029H
(Research Article)
Mater. Chem. Front., 2018,
2, 1436-1440
Rational design of phosphonocarboxylate metal–organic frameworks for light hydrocarbon separations†
Received
18th January 2018
, Accepted 27th March 2018
First published on 28th March 2018
Abstract
Metal–organic frameworks (MOFs) provoke intensive research interests on separations of small gas molecules. By introducing a coordinative phosphate group into a carboxylic acid linker, a ligand 4,4′-(hydroxyphosphoryl) dibenzoic acid (H3hpda) was successfully synthesized and used to construct microporous phosphonocarboxylate framework [Cu3(hpda)2·DMF·2H2O]·3DMF·H2O (DMF = N,N-dimethylformamide) and [Mg3(μ2-OH2)2(OH2)2(hpda)2]·3DMF·2H2O. Both of them possess narrow approachable channels and exhibit excellent performance on selective separation of light hydrocarbons (C1–C2), presenting a promising ligand design strategy for the construction of porous material aiming for natural gas upgrading.
Introduction
Light hydrocarbons (C1–C2) are of great importance as a petrochemical, industrial and energy resource, but they are usually obtained as a mixture. For example, natural gas, consisting primarily of methane, is a much cleaner fuel than petroleum; ethylene is the feedstock of manufacturing polymers, which can be produced by cracking processes of ethane on an industrial scale; acetylene is the essential raw material for chemical intermediates and also, it is called welding gas, which is useful in metal cutting, drilling, and welding. The prerequisite for industrial applications of these light hydrocarbons is acquisition of individual light hydrocarbons with high quality and purity.1 The similar sizes and volatilities of these light hydrocarbons make their separations by traditional cryogenic distillation energy-consuming and consequently, separation by selective adsorption is an interesting alternative.2–4
Metal–organic frameworks (MOFs), which are a new generation of porous materials with permanent porosity and fine-tunable pore structures, have been well-studied for the applications in gas storage and separation.5–12 However, most of the MOFs are not suitable for light hydrocarbons adsorption and separation due to the relatively weak interactions with the adsorbates.13 Therefore, the construction of MOFs with strong sorbent–sorbate interactions is under intensive exploration for gas storage and separation.3,14,15 Since the open metal site and pore surface tend to have a strong interaction with gas molecules, the introduction of unsaturated metal sites and small pore apertures in the MOFs structure have been attested to be an effective method to improve the separation performance.13,16,17 For example, to separate acetylene/ethylene mixture, Cui et al. judiciously controlled the pore size of the MOFs to realize the preferential binding and orderly assembly of acetylene molecules inside the pore.18 Li et al. designed a “single-molecule trap” with the appropriate size for trapping target gas molecules.19 In light of the small size of light hydrocarbons, MOFs possessing narrow pore structure and differential sorbent–sorbate interaction towards various components would be a promising candidate for effective light hydrocarbons separation. Herein, with the introduction of a phosphate group at the middle of a carboxylate linker, we designed a novel H3hpda (H3hpda = 4,4′-(hydroxyphosphoryl) dibenzoic acid, Scheme 1) ligand as an organic building block to construct microporous MOFs. This ligand exhibits the following advantages. First, the phosphate group would coordinate with the metal/metal cluster to effectively shorten the linker, thus not only resulting in a narrow pore, but also creating additional open metal sites. Second, the phosphate group regulates the carboxylate linker to an angular conformation, which would produce a diverse structure and even new topologies.20 Third, the phosphate group can act as a node in the self-assembly process. Hence, the resultant material would have a lower density with periodically substituting lighter phosphate groups for a proportion of metal nodes.21 Finally, although MOFs based on the phosphorus ligand are usually structurally robust and offer permanent porosity for gas adsorption and separation,22–24 the single crystals of phosphorous-MOFs are usually inaccessible, which caused many difficulties on the subsequent structure characterization.
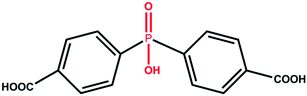 |
| Scheme 1 H3hpda ligand. | |
Introduction of phosphorous groups into carboxylate ligands provides a facile way to obtain a phosphorous-containing MOF in the single crystal form. We report herein two novel microporous phosphono-carboxylate frameworks [Cu3(hpda)2·DMF·2H2O]·3DMF·H2O and [Mg3(μ2-OH2)2(OH2)2(hpda)2]·3DMF·2H2O (hereafter referred to as FJI-C3 and FJI-C5, respectively), which were constructed based on H3hpda and copper (or magnesium) salt, which as expected, featured inherent 1D narrow channels closed to kinetic diameters of light hydrocarbons. The resultant materials exhibit excellent separation performance towards C2H2, C2H4, and C2H6 over CH4.
Experimental section
Synthesis of FJI-C3, [Cu3(hpda)2·DMF·2H2O]·3DMF·H2O
Cu(NO3)2·3H2O (12.0 mg, 0.05 mmol) and H3hpda (10 mg, 0.033 mmol) were added to the mixed solvent (2 mL, DMF/H2O = 1
:
1) in a 10 mL vial. The mixture was ultra-sonicated for 20 minutes, heated to 85 °C and kept at this temperature for 2 days, and then slowly cooled down to room temperature. Light blue single crystals were obtained by filtration. (Yield: 73% based on H3hpda). Elemental analysis for FJI-C3: calcd (%): C, 42.00; H, 4.55; N, 4.90. Found (%): C, 41.66; H, 4.44; N, 4.68. IR (KBr, cm−1): 451.54(s), 521.65(m), 571.89(w), 606.66(m), 710.82(m), 725.33(m), 740.82(s), 783.94(m), 846.87(w), 868.04(w), 1015.00(s), 1049.81(m), 1118.75(s), 1149.73(m), 1180.89(m), 1255.64(w), 1279.52(w), 1400.52(s), 1497.46(m), 1554.43(m), 1634.03(s), 1662.56(s), 2930.05(w), 3396.31(w).
Synthesis of FJI-C5, [Mg3(μ2-OH2)2(OH2)2(hpda)2]·3DMF·2H2O
Mg(NO3)2·6H2O (64.10 mg, 0.25 mmol) and H3hpda (10 mg, 0.033 mmol) were added to DMF solvent (2 mL) in a 10 mL vial. The mixed solution was slightly acidified with a small amount of neat HNO3 and then ultra-sonicated for 20 minutes, heated to 85 °C, and kept at this temperature for 2 days. After slowly cooling down to room temperature, colorless single crystals were obtained by filtration. (Yield: 78% based on H3hpda). Elemental analysis for FJI-C5: calcd (%): C, 44.18; H, 4.88; N, 4.18. Found (%): C, 44.56; H, 4.78; N, 4.53. IR (KBr, cm−1): 447.18(m), 504.23(m), 594.01(s), 667.04(m), 709.58(m), 720.89(m), 738.21(s), 786.89(s), 840.52(w), 1019.13(m), 1071.82(s), 1118.76(s), 1150.78(m), 1190.39(w), 1242.04(m),1418.63(s), 1497.25(m), 1554.89(s), 1670.97(s), 3361.07(s).
Result and discussion
Single crystal structure of FJI-C3
Single-crystal X-ray diffraction reveals that FJI-C3 crystallizes in the monoclinic space group P21/n. The asymmetric unit includes three crystallographically independent copper ions and two hpda3− ligands (Fig. 1a). The copper ions adopt two types of coordinated modes. Cu1 and Cu2 coordinate with four carboxylate groups to generate paddlewheel dinuclear secondary building units (SBUs) and the axial positions are occupied by two phosphate oxygen atoms from two different hpda3− ligands (Fig. 1c). Cu3 is five-coordinated with two phosphate oxygen atoms and three oxygen atoms from two H2O and one DMF molecules (Fig. 1d). The coordinatively unsaturated Cu3 will become an open metal site after the H2O and DMF removal, which would significantly improve the gas sorption capacities. For hpda3− ligand, each carboxylate group links with a copper paddlewheel, while one phosphate oxygen atom links with the axial position of Cu2(COO)4 paddlewheel and another phosphate oxygen atom coordinates to Cu3 atom (Fig. 1b). The dinuclear [Cu2(COO)4] paddlewheel and five-coordinated Cu3 are bridged by the hpda3− ligands to form a 3D microporous skeleton, which presents approximately square channels along the a-direction with a potential void space about 48.5% of crystal volume calculated by PLATON after removing DMF molecules and disordered solvent.25 Topologically, by considering the copper paddle-wheels as 6-connected nodes, another copper ion as a 2-connected node, and the hpda3− ligands as 4-connected nodes, FJI-C3 can be regarded as a 3-nodal 2,4,6-connected net with a point symbol of {4·65}2{42·610·83}{6}, thus revealing a new topology (Fig. 1f).
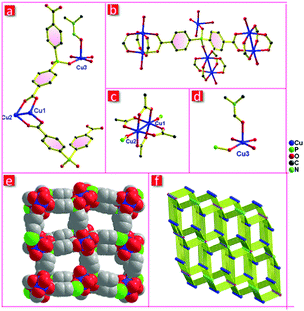 |
| Fig. 1 (a) The asymmetric unit of FJI-C3. (b) Coordination mode of the H3hpda ligand. (c and d) Two coordination modes of copper ions in FJI-C3. (e) Space-filling model showing channels along a-axis after the removal of DMF molecules and disordered solvent. (f) Topology representation of FJI-C3. | |
Single crystal structure of FJI-C5
Structure analysis discloses that FJI-C5 crystallizes in the orthorhombic space group Pnnm. The asymmetric unit of FJI-C5 (Fig. 2a) contains two crystallographically independent magnesium atoms and half hpda3− ligand and two H2O molecules. Mg1 is six-coordinated by four oxygen atoms from the carboxylate groups and two oxygens from the water molecules, while Mg2 is six-coordinated by two oxygen atoms from a carboxylate group, two from a phosphate group and the remaining from a water molecule. Mg1 and Mg2 are bridged by oxygen from water molecule to construct a trinuclear cluster [Mg3(μ2-OH2)2(OH2)2]6+ (Fig. 2b). The V-shaped hpda3− ligand links with four trinuclear clusters through the carboxylate and phosphate groups, extending into a 3D network with a square opening channel of about 5.0 Å along the a-axis (Fig. 2c). The calculated potential free void space of FJI-C5 is about 44.2% of the total crystal volume calculated by PLATON. From a topological viewpoint, the magnesium cluster can be regarded as 8-connected nodes and hpda3− ligand can be view as 4-connected nodes. The structure is a 4,8-connected flu network with the point symbol of {412·612·84}{46}2 (Fig. 2d).
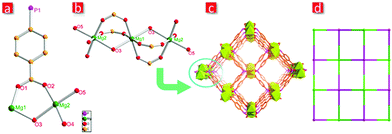 |
| Fig. 2 (a) Asymmetric unit of FJI-C5. (b) The cluster of [Mg3(μ2-OH2)2(OH2)2]6+ and its coordination environment. (c) Diagram of the 3D structure of FJI-C5 with open channels along the a-axis. (d) Simplified 4,8-connected flu topology of FJI-C5. | |
Adsorption and separation properties
Purity and homogeneity of FJI-C3 and FJI-C5 were confirmed by powder X-ray diffraction (PXRD) at room temperature and the matching analyses of PXRD patterns were carried out by LeBail route (Fig. S7, ESI†). Thermogravimetric analyses performed under N2 show that FJI-C3 and FJI-C5 maintain structural integrity till 360 °C and 500 °C, respectively, indicating their high thermal stability (Fig. S3 and S4, ESI†).
Prior to N2 adsorption experiments, the as-synthesized FJI-C3 and FJI-C5 were activated by heating at 200 °C under vacuum for 5 hours. The N2 isotherms were measured at 77 K and the as-prepared MOFs exhibit type-I isotherm, typical of the microporous materials. FJI-C3 has a Brunauer–Emmett–Teller (BET) surface area of 638 m2 g−1 and Langmuir surface area of 728 m2 g−1. FJI-C5 has a BET surface area of 584 m2 g−1 and Langmuir surface area of 638 m2 g−1 (Fig. 3a and b). Pore size distribution analyses conducted by the Horvath–Kawazoe method show that there is a narrow distribution of 5.1–5.4 Å for FJI-C3 and 4.9–6.2 Å for FJI-C5, which matches well with the crystal structure analyses.
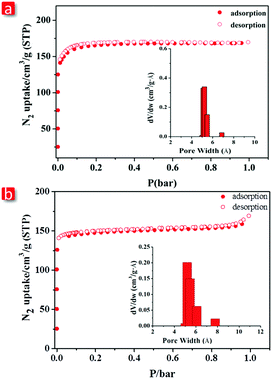 |
| Fig. 3 N2 adsorption isotherms at 77 K of (a) FJI-C3 and (b) FJI-C5. The Insets are pore distribution analysis by Horvath–Kawazoe method. | |
The existence of narrow channels in FJI-C3 and FJI-C5 inspired us to appraise their adsorption and separation properties towards light hydrocarbons. Single-component gas adsorption measurements at both 273 K and 298 K show that FJI-C3 adsorbs significantly higher amount of C2 hydrocarbons than CH4 (C2H2 55.0 cm3 g−1, C2H4 53.1 cm3 g−1, C2H6 44.5 cm3 g−1, and CH4 21.3 cm3 g−1 at 273 K and C2H2 43.6 cm3 g−1, C2H4 45.2 cm3 g−1, C2H6 41.5 cm3 g−1, and CH4 11.6 cm3 g−1 at 298 K) (Fig. 4a). Similarly, as shown in Fig. 5b, FJI-C5 uptakes more amount of C2H2 (71.8 cm3 g−1), C2H4 (60.0 cm3 g−1), and C2H6 (56.7 cm3 g−1) than CH4 (24.1 cm3 g−1) at 273 K (C2H2 51.8 cm3 g−1, C2H4 46.4 cm3 g−1, C2H6 46.8 cm3 g−1 than CH4 13.4 cm3 g−1 at 298 K). The low CH4 uptakes can be ascribed to the relatively low heats of adsorption for CH4, which are calculated to be 18.6 kJ mol−1 for FJI-C3 and 17.5 kJ mol−1 for FJI-C5, which are drastically lower than the heats of the adsorption for C2H6, C2H2, and C2H4 (Fig. 4c and 5c). The foregoing results demonstrate that the narrow channels in these two frameworks provide differential sorbent–sorbate interaction towards C2 hydrocarbons and methane, presenting a promising strategy for the construction MOFs aiming for light hydrocarbons separation.
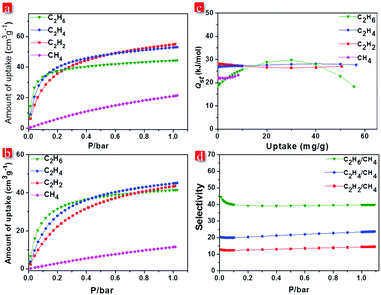 |
| Fig. 4 The light hydrocarbons (CH4, C2H2, C2H4, and C2H6) adsorption isotherms of FJI-C3 at (a) 273 K and (b) 298 K. (c) Isosteric heats of adsorption towards light hydrocarbons for FJI-C3. (d) Adsorption selectivity of C2H2, C2H4, and C2H6 with respect to CH4 are predicted by IAST at 298 K for binary equimolar mixtures. | |
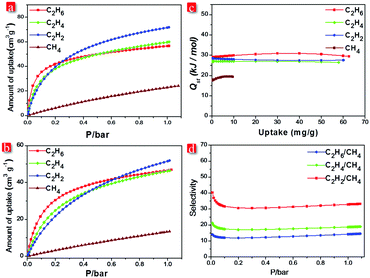 |
| Fig. 5 The light hydrocarbons (CH4, C2H2, C2H4, and C2H6) adsorption isotherms of FJI-C5 at (a) 273 K and (b) 298 K. (c) Isosteric heats of adsorption toward light hydrocarbons for FJI-C5. (d) Adsorption selectivity of C2H2, C2H4, and C2H6 with respect to CH4 are predicted by IAST at 298 K for binary equimolar mixtures. | |
To further investigate the separation efficiency, ideal adsorbed solution theory (IAST) based on the experimental single-component isotherms was performed to calculate the gas selectivity of C2 hydrocarbons over CH4 in a binary equimolar mixture. Prominently, for FJI-C3, at 1 bar and 298 K, the selectivities of C2H2, C2H4, and C2H6 with respect to CH4 are 14.6, 23.7, and 39.9, respectively. For FJI-C5, at the identical condition, the corresponding selectivities are 14.5, 19.0, and 33.3, which are slightly lower than those of FJI-C3. It is worth noting that the high C2H2/CH4, C2H4/CH4, and C2H6/CH4 selectivity reported herein rival the performance of some recently reported MOFs26–28 (Table S2, ESI†), for example, BUT-52 with the selectivity of 13.7 for C2H6/CH4 and 14.4 for C2H4/CH4.
We speculate that the high selectivity of C2 hydrocarbons with respect to CH4 may be attributed to (a) the narrow channels in FJI-C3 and FJI-C5, which are more closer to the kinetic diameters of C2 hydrocarbons except C2H2 (CH4 = 3.758, C2H6 = 4.443, C2H4 = 4.163, and C2H2 = 3.3 Å); (b) π–π interactions of C2H2, C2H4 with the benzene rings from the framework, which increase the interaction between the absorbents and the adsorbates; (c) the higher polarizabilities of C2H2 = 33–39 × 10−25, C2H4 = 42 × 10−25, and C2H6 = 44 × 10−25 cm3 than CH4 = 25 × 10−25 cm3, which would result in higher interaction energies of C2 hydrocarbons with porous surface.16,20,27,29 These synergistic effects lead to the high Qst and selectivities of C2 light hydrocarbons over CH4.
Conclusions
In summary, FJI-C3 and FJI-C5 have been successfully constructed based on a new pre-designed ligand, in which a coordinative phosphonate group is introduced to a carboxylate linker to regulate ligand with an angular conformation. The phosphonocarboxylate ligand endows these two structures with narrow channels and additional open metal sites. Therefore, FJI-C3 and FJI-C5 exhibit high selectivity of C2 light hydrocarbons over CH4. This study presents a novel method for the construction of MOFs with small pore size applied for natural gas separation and purification.
Conflicts of interest
There are no conflicts to declare.
Acknowledgements
R. C. gratefully acknowledges the National Natural Science Foundation of China (Grant 21521061, 21331006, 21520102001, 21571177) and Strategic Priority Research Program of the Chinese Academy of Sciences (XDB20000000). T. L. thanks the support of “Recruitment Program of Global Youth Experts”.
Notes and references
- H. M. Wen, H. Wang, B. Li, Y. Cui, H. Wang, G. Qian and B. Chen, Inorg. Chem., 2016, 55, 7214–7218 CrossRef CAS PubMed
.
- Y. B. He, R. Krishna and B. L. Chen, Energy Environ. Sci., 2012, 5, 9107–9120 CAS
.
- Y. B. He, W. Zhou, R. Krishna and B. L. Chen, Chem. Commun., 2012, 48, 11813–11831 RSC
.
- P. Q. Liao, W. X. Zhang, J. P. Zhang and X. M. Chen, Nat. Commun., 2015, 6, 8697 CrossRef PubMed
.
- Z. J. Lin, J. Lu, M. C. Hong and R. Cao, Chem. Soc. Rev., 2014, 43, 5867–5895 RSC
.
- D. Alezi, Y. Belmabkhout, M. Suyetin, P. M. Bhatt, L. J. Weselinski, V. Solovyeva, K. Adil, I. Spanopoulos, P. N. Trikalitis, A. H. Emwas and M. Eddaoudi, J. Am. Chem. Soc., 2015, 137, 13308–13318 CrossRef CAS PubMed
.
- K. Sumida, D. L. Rogow, J. A. Mason, T. M. McDonald, E. D. Bloch, Z. R. Herm, T. H. Bae and J. R. Long, Chem. Rev., 2012, 112, 724–781 CrossRef CAS PubMed
.
- K. C. Kim, J. Organomet. Chem., 2018, 854, 94–105 CrossRef CAS
.
- Z. Qiao, N. Wang, J. Jiang and J. Zhou, Chem. Commun., 2016, 52, 974–977 RSC
.
- L. Yan, Y. Liu, K. Zha, H. Li, L. Shi and D. Zhang, ACS Appl. Mater. Interfaces, 2017, 9, 2581–2593 CAS
.
- Z. Wang, T. Yan, L. Shi and D. Zhang, ACS Appl. Mater. Interfaces, 2017, 9, 15068–15078 CAS
.
- X. D. Zhu, K. Zhang, Y. Wang, W. W. Long, R. J. Sa, T. F. Liu and J. Lu, Inorg. Chem., 2018, 57, 1060–1065 CrossRef CAS PubMed
.
- J. R. Li, J. Sculley and H. C. Zhou, Chem. Rev., 2012, 112, 869–932 CrossRef CAS PubMed
.
- J. Li, Y. Guo, H. R. Fu, J. Zhang, R. B. Huang, L. S. Zheng and J. Tao, Chem. Commun., 2014, 50, 9161–9164 RSC
.
- K. Liu, X. Li, D. Ma, Y. Han, B. Li, Z. Shi, Z. Li and L. Wang, Mater. Chem. Front., 2017, 1, 1982–1988 RSC
.
- Y. B. Huang, Z. J. Lin, H. R. Fu, F. Wang, M. Shen, X. S. Wang and R. Cao, ChemSusChem, 2014, 7, 2647–2653 CrossRef CAS PubMed
.
- J. R. Li, R. J. Kuppler and H. C. Zhou, Chem. Soc. Rev., 2009, 38, 1477–1504 RSC
.
- J. R. Li, J. Yu, W. Lu, L. B. Sun, J. Sculley, P. B. Balbuena and H. C. Zhou, Nat. Commun., 2013, 4, 1538 CrossRef PubMed
.
- X. Cui, K. Chen, H. Xing, Q. Yang, R. Krishna, Z. Bao, H. Wu, W. Zhou, X. Dong, Y. Han, B. Li, Q. Ren, M. J. Zaworotko and B. Chen, Science, 2016, 353, 141–144 CrossRef CAS PubMed
.
- L. Li, X. Wang, J. Liang, Y. Huang, H. Li, Z. Lin and R. Cao, ACS Appl. Mater. Interfaces, 2016, 8, 9777–9781 CAS
.
- S. M. Humphrey, P. K. Allan, S. E. Oungoulian, M. S. Ironside and E. R. Wise, Dalton Trans., 2009, 2298–2305 RSC
.
- G. K. Shimizu, R. Vaidhyanathan and J. M. Taylor, Chem. Soc. Rev., 2009, 38, 1430–1449 RSC
.
- Y. Ling, M. Deng, Z. Chen, B. Xia, X. Liu, Y. Yang, Y. Zhou and L. Weng, Chem. Commun., 2013, 49, 78–80 RSC
.
- S. S. Iremonger, J. Liang, R. Vaidhyanathan and G. K. Shimizu, Chem. Commun., 2011, 47, 4430–4432 RSC
.
- A. L. Spek, J. Appl. Crystallogr., 2003, 36, 7 CrossRef CAS
.
- Y. Han, H. Zheng, K. Liu, H. Wang, H. Huang, L. H. Xie, L. Wang and J. R. Li, ACS Appl. Mater. Interfaces, 2016, 8, 23331–23337 CAS
.
- H.-R. Fu, F. Wang and J. Zhang, Dalton Trans., 2015, 44, 2893–2896 RSC
.
- Y. B. He, Z. J. Zhang, S. C. Xiang, F. R. Fronczek, R. Krishna and B. L. Chen, Chem. – Eur. J., 2012, 18, 613–619 CrossRef CAS PubMed
.
- W. Huang, J. Jiang, D. Wu, J. Xu, B. Xue and A. M. Kirillov, Inorg. Chem., 2015, 54, 10524–10526 CrossRef CAS PubMed
.
Footnote |
† Electronic supplementary information (ESI) available: Crystal structures, PXRD patterns, TGA curves, IR spectra, crystallographic data and structure refinement parameters. CCDC 1444652 and 1444653. For ESI and crystallographic data in CIF or other electronic format see DOI: 10.1039/c8qm00029h |
|
This journal is © the Partner Organisations 2018 |