DOI:
10.1039/C7QM00560A
(Research Article)
Mater. Chem. Front., 2018,
2, 554-558
Amplification effect of circularly polarized luminescence induced from binaphthyl-based zinc(II) chiral coordination polymers†
Received
7th December 2017
, Accepted 10th January 2018
First published on 10th January 2018
Abstract
Two novel binaphthyl-based dipyrrin zinc(II) chiral coordination polymers (R/S-P1) can be synthesized by coordination reaction. R/S-P1 can exhibit stronger Cotton effects and circularly polarized luminescence (CPL) emission signals compared with the chiral complex monomers (R/S-M1). The luminescence dissymmetry factors (glum) can reach as high as 9 × 10−3 for R/S-P1.
Introduction
Chiral coordination polymers (CCPs) have been attracting attention because of their potential applications in enantioselective recognition and separation,1 asymmetric catalysis,2 nonlinear optics3 and sensors.4 Generally, utilizing enantiopure chiral building blocks can be regarded as an effective strategy to directly construct CCPs,5 but homochiral coordination polymers from achiral organic ligands can also be achieved through chirality induction and spontaneous resolution.6 The architectures and optical properties of CCPs can be modified by exploiting different building blocks and environments (chiral agents and circularly polarized light). Zhuang's group reported the absolute asymmetric synthesis of a chiral coordination polymer from achiral ligands by irradiation with circularly polarized light (CPL) during the reaction and crystallization.7
Circularly polarized luminescence (CPL), which is receiving increasing interest, has been developed in systems like chiral lanthanide complexes,8 chiral small organic molecules,9 chiral polymers,10 chiral assemblies,11 chiral inorganic materials12 and so on. As a class of excellent CPL materials, chiral lanthanide complexes have been widely studied and proved to exhibit high values of luminescence dissymmetry factors (glum).13 However, other transition metal complexes like Ir(III), Ru(II), Pt(II) and Zn(II) complexes have attracted attention for CPL emission materials owing to their unique optoelectronic properties.14 Zhou's group synthesized two pairs of Ir(III) complex enantiomers with a chiral metal center and chiral carbons, whose CPL signals were mainly determined by the conformations (Λ/Δ) of the chiral metal center.14a Clever's group reported a pair of square-planar Pt(II) complexes with the metal serving as the stereo center from the achiral chelate ligand, and the chiral complexes exhibited phosphorescence properties and CPL signals.14b However, research on CPL active chiral complexes used to focus on small molecules, and there are few reports on CPL active CCPs.15 Therefore, developing CCPs which can exhibit CPL response signals is of great significance.
Among emissive transition metal complexes, Zn(II) complexes have excellent electroluminescence and photoluminescence properties and have been particularly applied in fluorescent probes,16 organic light emitting diodes (OLEDs),17 solar cells18 and CPL materials.19 Zn(II) complexes with tunable emission wavelength and intensity can be easily fabricated by introducing different ligands and counter-anions.20 Our group reported that an in situ generated 1
:
1 Zn(II) complex of a chiral (S)-BINAM-based polymer can serve as a fluorescence sensor for highly enantioselective recognition of N-Boc-protected alanine, and the value of the enantiomeric fluorescence difference ratio (ef) can reach as high as 6.90.21 Recently, Nishihara's group reported a new class of heteroleptic (dipyrrinato)zinc(II) complexes that have high fluorescence quantum yields (ΦF) and CPL emission signals with glum values of ±1 × 10−3.19d Herein, we designed two pairs of chiral binaphthyl-based dipyrrin Zn(II) coordination polymers (R/S-P1) and the complex monomers (R/S-M1). R/S-P1 can exhibit stronger CD and CPL signals compared with R/S-M1. The CPL |glum| values can be up to 9 × 10−3 for the chiral coordination polymers.
Results and discussion
The synthesis procedures of R/S-M1 and R/S-P1 are outlined in Scheme 1. We first synthesized chiral binaphthyl-based boron dipyrromethenes (BODIPYs) from binaphthalene-3,3′-dicarbaldehyde or binaphthalene-3-carbaldehyde. Then the chiral dipyrrin ligands were obtained from the deboration reactions of the BODIPYs according to the literature.22 The chiral coordination polymers and monomers were synthesized by mixing the chiral dipyrrin ligands with zinc(II) acetate in a mixture of dichloromethane and methanol at room temperature. Then the complexes can be isolated by precipitating them in methanol. The detailed procedures and characterization of the ligands and complexes are described in the ESI.† The 1H NMR spectra and GPC data of R/S-P1 can demonstrate the formation of the coordination polymers. The thermogravimetric analysis (TGA) curves reveal that these chiral complexes have high degradation temperature (Td) of 5% weight loss above 340 °C (Fig. S1, ESI†), indicating that these chiral complexes are of good stability and can be applied for CPL emission or optoelectronic materials. R/S-P1 show good solubility in common organic solvents, which can be attributed to the nonplanar polymer backbone structure and the flexible n-octyl group substituents.
 |
| Scheme 1 The synthesis procedures of R/S-M1 and R/S-P1. | |
We carried out X-ray photoelectron spectroscopy (XPS) to identify the chiral coordination polymers. Fig. 1 shows the XPS results of M1 and P1 in the N 1s and Zn 2p3 regions. M1 showed a single peak at 394.9 eV in the N 1s region and a single peak at 1018.1 eV in the Zn 2p3 region. However, the XPS peaks of P1 showed a little splitting, which can be ascribed to the different polymerization degrees of P1. The N
:
Zn abundance ratios for M1 and P2 which were calculated from the peak areas corrected by photoionization cross-section were 4.48
:
1.13 and 4.97
:
1.22 (Fig. S2, ESI†), respectively, which are consistent with the theoretical ratio of 4
:
1.
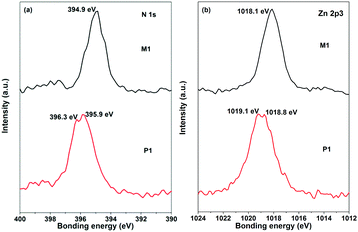 |
| Fig. 1 XPS spectra of M1 and P1. | |
The UV-vis absorption spectra of L1, L2, P1 and M1 were measured in THF solution (1.0 × 10−5 mol L−1). As shown in Fig. 2, L1 and L2, and P1 and M1 have similar absorption spectra due to the analogous structures. The peaks at 280 nm and 330 nm can be assigned to the π–π* transition of the binaphthyl, and the absorption bands around 450 nm can be ascribed to the π–π* transition of the dipyrrin ligand in L1 and L2. However, P1 and M1 exhibit absorption bands around 490 nm, which are red-shifted by 40 nm compared with L1 and L2 and can be regarded as the typical absorption bands of Zn(II) complexes. The chiral coordination polymers P1 have a broader peak at 490 nm compared with M1 because of the extended conjugated structure of the polymer backbones.
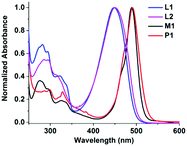 |
| Fig. 2 The UV-vis absorption spectra of L1, L2, P1 and M1 (1.0 × 10−5 mol L−1 in THF). | |
The fluorescence spectra of R/S-M1 and R/S-P1 are presented in Fig. 3 (1.0 × 10−4 mol L−1 for zinc in toluene). The chiral complex monomers (R/S-M1) exhibit an emission peak at 538 nm, while the chiral coordination polymers (R/S-P1) emit at 547 nm. The emission peak of R/S-P1 is red-shifted by 9 nm compared with R/S-M1, which can also be ascribed to the extended π-conjugated of ligand L1. We also measured the fluorescence quantum yields (ΦF) and lifetimes (τ) of the complexes. As shown in Table 1, the fluorescence quantum yields of R/S-M1 and R/S-P1 are 0.30% and 0.39% in toluene. The low ΦF of the complexes can be attributed to the thermal transition from the emissive lowest singlet excited state (1π–π*) localized on either dipyrrin ligand to non-emissive charge-separated states. The nonradiative rate constants (knr) can further explain this phenomenon. The larger knr values of R/S-M1 represent the generation of more non-emissive charge-separated states, which results in the lower ΦF of R/S-M1.
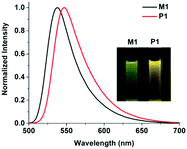 |
| Fig. 3 The fluorescence spectra of R/S-M1 and R/S-P1 (1.0 × 10−4 mol L−1 in toluene, λex = 450 nm); inset: photographs taken under UV illumination (365 nm). | |
Table 1 The fluorescence properties and decay parameters of R/S-P1 and R/S-M1
|
Fluorescence decayb |
Sample |
λ
em (nm) |
Φ
F
(%) |
A
1/A2 (%) |
τ
1 (ns) |
τ
2 (ns) |
〈τ〉b (ns) |
k
f (s−1) |
k
nr (s−1) |
Φ
F measured using a calibrated integrating sphere, excitation wavelength, λex = 450 nm.
Determined from I = A1 exp(−t/τ1) + A2 exp(−t/τ2); herein, τ1 and τ2 are the lifetimes of the shorter-lived and longer-lived species, and A1 and A2 are their respective fractions, respectively. The weighted mean lifetime 〈τ〉 was obtained from the equation: 〈τ〉 = (A1τ1 + A2τ2)/(A1 + A2). The radiative rate constant kf and non-radiative rate constant knr were obtained from the equations: kf = ΦF/〈τ〉 and knr = (1 − ΦF)/〈τ〉, respectively.
|
P1 |
547 |
0.39 |
91/9 |
0.28 |
1.03 |
0.30 |
1.3 × 107 |
3.3 × 109 |
M1 |
538 |
0.30 |
97/3 |
0.12 |
0.81 |
0.13 |
2.3 × 107 |
7.7 × 109 |
In order to investigate the chiroptical properties of the chiral complexes, we performed CD absorption determination of R/S-L1, R/S-L2, R/S-M1 and R/S-P1. As is evident from Fig. 4, we can observe that R/S-M1 and R/S-P1 can exhibit clear mirror-image CD bands. The Cotton effects around 280 nm and 340 nm in the short wavelength region can be assigned to the characteristic absorption of chiral binaphthyl moieties. R/S-M1 and R/S-P1 can show strong positive–negative Cotton effects in the long wavelength region at 490 nm, which correspond to the absorption bands of the dipyrrin Zn(II) complexes. The strong positive–negative Cotton effects, which can be explained as strong exciton coupling,23 demonstrate that the binaphthyl chirality has been transferred successfully to the Zn(II) complexes. R/S-L2 can also display obvious positive–negative Cotton effects at 450 nm. However, there are almost no Cotton effects in the long wavelength region for R/S-L1. This phenomenon can be ascribed to the C2-symmetric molecule structure of R/S-L2, which is essential for exciton coupling. Interestingly, the Cotton effects of R/S-P1 are much stronger than those of R/S-M1 in the long wavelength region. The absorption dissymmetry factors (gabs) at the wavelengths of the maximal CD signals are ±6 × 10−4 (at 499 nm) and ±4 × 10−3 (at 507 nm) for R/S-M1 and R/S-P1, respectively.
 |
| Fig. 4 The CD spectra of (a) R/S-L1 and R/S-L2 and (b) R/S-M1 and R/S-P1 (1.0 × 10−5 mol L−1 in THF, cell length: 10 mm, bandwidth: 1 nm, scanning speed: 200 nm min−1, data pitch: 1 nm, accumulations: 3). | |
The CPL spectra of R/S-M1 and R/S-P1 were measured in JASCO CPL-300. As can be seen in Fig. 5, CPL response signals of R/S-P1 can exhibit obvious mirror-image monosignals in toluene solutions at the Zn(II) complex emission peaks. The CPL glum values of R/S-P1 at the wavelengths of the maximal CPL signals are 8 × 10−3 (at 553 nm for the R configuration) and −9 × 10−3 (at 560 nm for the S configuration). Similar to CD spectra, the CPL signals of R/S-M1 are much weaker than R/S-P1 and the glum values are just ±2 × 10−3 at 570 nm. The CD and CPL signals of R/S-M1 and R/S-P1 can clearly indicate the chiral amplification of the chiral coordination polymers. The chiral amplification may come from the more regular arrangement of chiral monomers and chiral accumulation in the polymer backbone structure. R/S-P1 can also show apparently larger glum values compared with reported non-lanthanide transition metal complexes (mostly smaller than 5 × 10−3).14,19 We also measured the specific rotations of R/S-M1 and R/S-P1. The [α]25D values of R/S-M1 and R/S-P1 (c = 1.0, CH2Cl2) are +682/−663 and +2313/−2488, respectively, which also support the chiral amplification of R/S-P1.
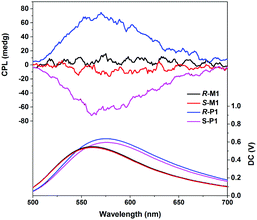 |
| Fig. 5 The CPL spectra of R/S-M1 and R/S-P1 (1.0 × 10−4 mol L−1 in toluene, λex = 340 nm, cell length: 10 mm, Ex & Em slit width: 3000 μm, scanning speed: 200 nm min−1, data pitch: 1 nm, accumulations: 3). | |
Conclusions
In summary, chiral binaphthyl-based Zn(II) complex monomers (R/S-M1) and coordination polymers (R/S-P1) can be obtained through direct coordination reactions. The CD and CPL signals can exhibit several times enhancement from monomers to polymers, which can be attributed to the chiral amplification of the chiral coordination polymer backbone structure. The |glum| values of R/S-P1 can be up to 9 × 10−3, which is larger than most non-lanthanide transition metal complexes. The results of this work can provide a new strategy to design chiral complex CPL materials.
Conflicts of interest
There are no conflicts to declare.
Acknowledgements
This work was supported by the National Natural Science Foundation of China (21674046, 21474048 and 51673093).
Notes and references
-
(a) R. Vaidhyanathan, D. Bradshaw, J. N. Rebilly, J. P. Barrio, J. A. Gould, N. G. Berry and M. J. Rosseinsky, Angew. Chem., Int. Ed., 2006, 45, 6495 CrossRef CAS PubMed
;
(b) K. Suh, M. P. Yutkin, D. N. Dybtsev, V. P. Fedinc and K. Kim, Chem. Commun., 2012, 48, 513 RSC
;
(c) J. Liu, F. Wang, Q. R. Ding and J. Zhang, Inorg. Chem., 2016, 55, 12520 CrossRef CAS PubMed
;
(d) J. D. Martell, L. B. Porter-Zasada, A. C. Forse, R. L. Siegelman, M. I. Gonzalez, J. Oktawiec, T. Runcevski, J. Xu, M. Srebro-Hooper, P. J. Milner, K. A. Colwell, J. Autschbach, J. A. Reimer and J. R. Long, J. Am. Chem. Soc., 2017, 139, 16000 CrossRef CAS PubMed
.
-
(a) J. S. Seo, D. Whang, H. Lee, S. I. Jun, J. Oh, Y. J. Jeon and K. Kim, Nature, 2000, 404, 982 CrossRef CAS PubMed
;
(b) M. Banerjee, S. Das, M. Yoon, H. J. Choi, M. H. Hyun, S. M. Park, G. Seo and K. Kim, J. Am. Chem. Soc., 2009, 131, 7524 CrossRef PubMed
;
(c) S. Takizawa, H. Somei, D. Jayaprakash and H. Sasai, Angew. Chem., Int. Ed., 2003, 42, 5711 CrossRef CAS PubMed
;
(d) J. Zhang, X. Han, X. W. Wu, Y. Liu and Y. Cui, J. Am. Chem. Soc., 2017, 139, 8277 CrossRef CAS PubMed
;
(e) X. Chen, H. Jiang, B. Hou, W. Gong, Y. Liu and Y. Cui, J. Am. Chem. Soc., 2017, 139, 13476 CrossRef CAS PubMed
.
- C. Wang, T. Zhang and W. B. Lin, Chem. Rev., 2012, 112, 1084 CrossRef CAS PubMed
.
-
(a) Y. H. Yu, H. T. Ye, G. F. Hou, C. Y. Ren, J. S. Gao and P. F. Yan, Cryst. Growth Des., 2016, 16, 5669 CrossRef CAS
;
(b) Q. Zhang, Y. Hong, N. Chen, D. D. Tao, Z. Li and Y. B. Jiang, Chem. Commun., 2015, 51, 8017 RSC
.
-
(a) S. Bernhard, K. Takada, D. J. Díaz, H. D. Abruña and H. Mürner, J. Am. Chem. Soc., 2001, 123, 10265 CrossRef CAS PubMed
;
(b) R. R. Pal, M. Higuchi and D. G. Kurth, Org. Lett., 2009, 11, 3562 CrossRef CAS PubMed
;
(c) Y. Tang, M. Chen, D. J. Qian, L. Zhang and M. H. Liu, Langmuir, 2013, 29, 6308 CrossRef CAS PubMed
;
(d) W. W. Lestari, H. C. Streit, P. Lönnecke, C. Wickleder and E. Hey-Hawkins, Dalton Trans., 2014, 43, 8188 RSC
.
-
(a) E. Q. Gao, Y. F. Yue, S. Q. Bai, Z. He and C. H. Yan, J. Am. Chem. Soc., 2004, 126, 1419 CrossRef CAS PubMed
;
(b) K. K. Bisht and E. Suresh, J. Am. Chem. Soc., 2013, 135, 15690 CrossRef CAS PubMed
;
(c) P. C. Rao, S. P. Chaudhary, D. Kuznetsov and S. Mandal, Inorg. Chem., 2016, 55, 12669 CrossRef CAS PubMed
;
(d) X. L. Luo, Y. Cao, T. Wang, G. H. Li, J. G. Li, Y. G. Yang, Z. X. Xu, J. Zhang, Q. S. Huo, Y. L. Liu and M. Eddaoudi, J. Am. Chem. Soc., 2016, 138, 786 CrossRef CAS PubMed
.
- S. T. Wu, Z. W. Cai, Q. Y. Ye, C. H. Weng, X. H. Huang, X. L. Hu, C. C. Huang and N. F. Zhuang, Angew. Chem., Int. Ed., 2014, 53, 12860 CrossRef CAS PubMed
.
-
(a) R. Carr, N. H. Evans and D. Parker, Chem. Soc. Rev., 2012, 41, 7673 RSC
;
(b) A. T. Frawley, R. Pal and D. Parker, Chem. Commun., 2016, 52, 13349 RSC
;
(c) M. Leonzio, A. Melchior, G. Faura, M. Tolazzi, F. Zinna, L. Di Bari and F. Piccinelli, Inorg. Chem., 2017, 56, 4413 CrossRef CAS PubMed
;
(d) Y. Kono, N. Hara, M. Shizuma, M. Fujiki and Y. Imai, Dalton Trans., 2017, 46, 5170 RSC
;
(e) B. Zercher and T. A. Hopkins, Inorg. Chem., 2016, 55, 10899 CrossRef CAS PubMed
.
-
(a) S. Feuillastre, M. Pauton, L. Gao, A. Desmarchelier, A. J. Riives, D. Prim, D. Tondelier, B. Geffroy, G. Muller, G. Clavier and G. Pieters, J. Am. Chem. Soc., 2016, 138, 3990 CrossRef CAS PubMed
;
(b) E. M. Sánchez-Carnerero, F. Moreno, B. L. Maroto, A. R. Agarrabeitia, M. J. Ortiz, B. G. Vo, G. Muller and S. de la Moya, J. Am. Chem. Soc., 2014, 136, 3346 CrossRef PubMed
;
(c) W. L. Yang, G. Longhi, S. Abbate, A. Lucotti, M. Tommasini, C. Villani, V. J. Catalano, A. O. Lykhin, S. A. Varganov and W. A. Chalifoux, J. Am. Chem. Soc., 2017, 139, 13102 CrossRef CAS PubMed
.
-
(a) D. Di Nuzzo, C. Kulkarni, B. D. Zhao, E. Smolinsky, F. Tassinari, S. C. J. Meskers, R. Naaman, E. W. Meijer and R. H. Friend, ACS Nano, 2017, 11, 12713 CrossRef CAS PubMed
;
(b) T. Nishikawa, Y. Nagata and M. Suginome, ACS Macro Lett., 2017, 6, 431 CrossRef CAS
;
(c) J. Yan, F. Ota, B. A. San Jose and K. Akagi, Adv. Funct. Mater., 2017, 27, 1604529 CrossRef
.
-
(a) J. Z. Liu, H. Su, L. Meng, Y. Zhao, C. Deng, J. C. Y. Ng, P. Lu, M. Faisal, J. W. Y. Lam, X. Huang, H. Wu, K. S. Wong and B. Z. Tang, Chem. Sci., 2012, 3, 2737 RSC
;
(b) D. Yang, P. Duan, L. Zhang and M. Liu, Nat. Commun., 2017, 8, 15727 CrossRef CAS PubMed
;
(c) R. Sethy, J. Kumar, R. Métivier, M. Louis, K. Nakatani, N. M. T. Mecheri, A. Subhakumari, K. G. Thomas, T. Kawai and T. Nakashima, Angew. Chem., Int. Ed., 2017, 56, 15053 CrossRef CAS PubMed
;
(d) B. S. Li, R. Wen, S. Xue, L. Shi, Z. Tang, Z. Wang and B. Z. Tang, Mater. Chem. Front., 2017, 1, 646 RSC
.
-
(a) L. Shi, L. Zhu, J. Guo, L. Zhang, Y. Shi, Y. Zhang, K. Hou, Y. Zheng, Y. Zhu, J. Lv, S. Liu and Z. Tang, Angew. Chem., Int. Ed., 2017, 56, 15397 CrossRef CAS PubMed
;
(b) Y. Duan, L. Han, J. Zhang, S. Asahina, Z. Huang, L. Shi, B. Wang, Y. Cao, Y. Yao, L. Ma, C. Wang, R. K. Dukor, L. Sun, C. Jiang, Z. Tang, L. A. Nafie and S. Che, Angew. Chem., Int. Ed., 2015, 54, 15170 CrossRef CAS PubMed
.
-
(a) J. L. Lunkley, D. Shirotani, K. Yamanari, S. Kaizaki and G. Muller, Inorg. Chem., 2011, 50, 12724 CrossRef CAS PubMed
;
(b) N. Koiso, Y. Kitagawa, T. Nakanishi, K. Fushimi and Y. Hasegawa, Inorg. Chem., 2017, 56, 5741 CrossRef CAS PubMed
.
-
(a) T. Y. Li, Y. X. Zheng and Y. H. Zhou, Dalton Trans., 2016, 45, 19234 RSC
;
(b) T. R. Schulte, J. J. Holstein, L. Krause, R. Michel, D. Stalke, E. Sakuda, K. Umakoshi, G. Longhi, S. Abbate and G. H. Clever, J. Am. Chem. Soc., 2017, 139, 6863 CrossRef CAS PubMed
;
(c) M. Gon, Y. Morisaki and Y. Chujo, Chem. Commun., 2017, 53, 8304 RSC
;
(d) T. Biet, T. Cauchy, Q. Sun, J. Ding, A. Hauser, P. Oulevey, T. Bürgi, D. Jacquemin, N. Vanthuyne, J. Crassous and N. Avarvari, Chem. Commun., 2017, 53, 9210 RSC
.
- R. Aoki, R. Toyoda, J. F. Kögel, R. Sakamoto, J. Kumar, Y. Kitagawa, K. Harano, T. Kawai and H. Nishihara, J. Am. Chem. Soc., 2017, 139, 16024 CrossRef CAS PubMed
.
-
(a) L. E. Kreno, K. Leong, O. K. Farha, M. Allendorf, R. P. Van Duyne and J. T. Hupp, Chem. Rev., 2012, 112, 1105 CrossRef CAS PubMed
;
(b) Z. C. Zhu, L. Xu, H. Li, X. Zhou, J. G. Qin and C. L. Yang, Chem. Commun., 2014, 50, 7060 RSC
;
(c) A. Terenzi, A. Lauria, A. M. Almericoa and G. Barone, Dalton Trans., 2015, 44, 3527 RSC
;
(d) T. Jiang, N. N. Lu, Y. D. Hang, J. Yang, J. Mei, J. Wang, J. L. Hua and H. Tian, J. Mater. Chem. C, 2016, 4, 10040 RSC
.
-
(a) R. J. Wang, L. J. Deng, M. Fu, J. L. Cheng and J. Y. Li, J. Mater. Chem., 2012, 22, 23454 RSC
;
(b) K. Wang, F. C. Zhao, C. G. Wang, S. Y. Chen, D. Chen, H. Y. Zhang, Y. Liu, D. G. Ma and Y. Wang, Adv. Funct. Mater., 2013, 23, 2672 CrossRef CAS
;
(c) Y. Sakai, Y. Sagara, H. Nomura, N. Nakamura, Y. Suzuki, H. Miyazaki and C. Adachi, Chem. Commun., 2015, 51, 3181 RSC
;
(d) G. Cheng, G. K. M. So, W. P. To, Y. Chen, C. C. Kwok, C. Ma, X. Guan, X. Chang, W. M. Kwok and C. M. Che, Chem. Sci., 2015, 6, 4623 RSC
.
- V. M. Manninen, W. A. E. Omar, J. P. Heiskanen, H. J. Lemmetyinen and O. E. O. Hormi, J. Mater. Chem., 2012, 22, 22971 RSC
.
-
(a) K. D. Oyler, F. J. Coughlin and S. Bernhard, J. Am. Chem. Soc., 2007, 129, 210 CrossRef CAS PubMed
;
(b) M. Morisue, H. Fukui, M. Shimizu, K. Inoshita, Y. Morisaki and Y. Chujo, Tetrahedron Lett., 2014, 55, 271 CrossRef CAS
;
(c) M. Morisue, T. Yumura, R. Sawada, M. Naito, Y. Kuroda and Y. Chujo, Chem. Commun., 2016, 52, 2481 RSC
;
(d) J. F. Kögel, S. Kusaka, R. Sakamoto, T. Iwashima, M. Tsuchiya, R. Toyoda, R. Matsuoka, T. Tsukamoto, J. Yuasa, Y. Kitagawa, T. Kawai and H. Nishihara, Angew. Chem., Int. Ed., 2016, 55, 1377 CrossRef PubMed
.
-
(a) T. Tsukamoto, R. Aoki, R. Sakamoto, R. Toyoda, M. Shimada, Y. Hattori, M. Asaoka, Y. Kitagawa, E. Nishibori, M. Nakanoc and H. Nishihara, Chem. Commun., 2017, 53, 3657 RSC
;
(b) J. A. Marafie, D. D. C. Bradley and C. K. Williams, Inorg. Chem., 2017, 56, 5688 CrossRef CAS PubMed
;
(c) X. H. Tian, Y. Z. Zhu, Q. Zhang, R. L. Zhang, J. Y. Wu and Y. P. Tian, Chem. Commun., 2017, 53, 7941 RSC
.
- J. L. Hou, F. Y. Song, L. Wang, G. Wei, Y. X. Cheng and C. J. Zhu, Macromolecules, 2012, 45, 7835 CrossRef CAS
.
- S. Kusaka, R. Sakamoto, Y. Kitagawa, M. Okumura and H. Nishihara, Chem. – Asian J., 2012, 7, 907 CrossRef CAS PubMed
.
- F. Zinna, T. Bruhn, C. A. Guido, J. Ahrens, M. Bröring, L. Di Bari and G. Pescitelli, Chem. – Eur. J., 2016, 22, 16089 CrossRef CAS PubMed
.
Footnote |
† Electronic supplementary information (ESI) available. See DOI: 10.1039/c7qm00560a |
|
This journal is © the Partner Organisations 2018 |
Click here to see how this site uses Cookies. View our privacy policy here.